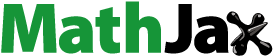
Abstract
Twinning-mediated plasticity has great potential for enhancing the strength and plasticity of Mg alloys, which is limited due to the lack of twin types. Here, we report a novel multistage twinning mode, i.e. the dominant twinning mode changes from single twins to the co-existence of
and
twins, in a deformed Mg-Al-Gd alloy. Introducing novel multistage twinning mode to accommodate strains appreciably along c-axes, combined with the interactions of high-density dislocations and dense twin boundaries, guarantees the high strain hardening of Mg alloys. These findings provide new insights into deformation mechanisms and mechanical properties of Mg-alloys.
GRAPHICAL ABSTRACT
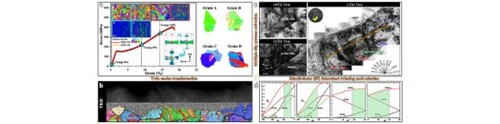
IMPACT STATEMENT
Introducing novel multistage twinning mode to accommodate strains appreciably along c-axes, combined with the interactions of high-density dislocations and dense twin boundaries, guarantees the high strain hardening of Mg alloys.
1. Introduction
Mg-Al based alloys are widely studied and used in high-end applications, such as the aerospace and communications industries [Citation1,Citation2]. However, high-strength Mg-Al-based alloys often exhibit poor plasticity. The limited number of independent slip systems, and the difficulty in activating pyramidal- slips due to the higher critical resolved shear stress (CRSS) lead to difficulties in accommodating the strain along c-axes of hexagonal close-packed (HCP) Mg alloys [Citation3,Citation4]. In addition to dislocation slips, mechanical twinning plays a crucial role in accommodating the strain along c-axes of Mg alloys through the lattice reorientation [Citation5,Citation6]. Therefore, it may be feasible to enhance the plasticity of Mg alloys by manipulating the twinning behavior, on the premise of ensuring strength.
For Mg alloys, although a few twinning modes, such as ,
and
, have been predicted in theory and relevant experimental data have been reported in literature at present [Citation7,Citation8], only
extension twins have been widely observed experimentally [Citation9–11]. Essentially, the
twinning is associated with a lattice rotation of 86° about
axes, resulting in a strong crystallographic texture with the c-axes aligned close to the loading direction during compression. As a consequence, dislocation plasticity becomes unfavorable upon further compression, which leads to reduced plasticity and premature failure. This inherent dilemma that only one extension twinning mode cannot accommodate complex strains along c-axes has propelled numerous studies of new twinning modes, such as
twins in Mg-14 wt.% Gd alloys [Citation12], as well as twin-like
tilt boundaries in a high strain rate deformed Mg-9 wt.% Y alloy [Citation13]. Nevertheless, it is still unclear whether the
twins are extension or contraction twins. Furthermore, the effect of twins on mechanical properties of Mg alloys remains underexplored. Recently, through theoretical analysis and atomic simulations, Cayron [Citation14], Ostapovets [Citation15] and Gao et al. [Citation16] suggest a new extension twinning mode of
that is not predicted by the classical theory of twinning [Citation17,Citation18]. Ostapovets et al. [Citation15] speculate that the
twin may impede dislocation movement, which contributes to the strength of pure Mg. However, up till now, the effect of
twinning on the mechanical behavior of Mg alloys has not been studied and experimentally verified. Therefore, it is necessary to explore the underlying mechanism of the
twinning mode on the plastic deformation behavior of Mg alloys.
Here, we report a novel multistage twinning mode, i.e. the dominant twinning mode changes from single twins to the co-existence of
and
twins.
twins have been identified in an Mg-Al-Gd alloy using delicate microstructure characterizations. Simultaneously, multiple slip systems are activated within
twins, where the mean free path of dislocations is confined by
twin boundaries, which contributes to large work hardening. Additionally, the selection of
twinning mode and resultant maximum theoretical strain induced by
twinning have been determined by calculating the Schmid factor (SF) and lattice distortion. Introducing the multistage twinning mode can break the dilemma that the single
twinning is inadequate to accommodate the plastic deformation along c-axes, which guarantees a high plasticity (∼18%) in the present Mg-Al-Gd alloy upon compression. These findings can provide new perspectives for designing and preparing high-performance Mg alloys by tailoring multiple twinning modes.
2. Materials and methods
The Mg-1Al-1.8Gd (wt.%) alloy was fabricated via melting high-purity Mg (99.99 wt.%), high-purity Al (99.99 wt.%) and Mg-73.49 wt.%Gd alloy, followed by casting under a protective atmosphere. The as-cast ingot was preheated at 450°C for 2 h and subsequently hot-extruded with an extrusion ratio of 20:1. Cylindrical compression samples with dimensions of Ø 8 mm × 12 mm were subjected to compression testing at room temperature. The tests were conducted using an AGS-X-100 kN testing machine at a strain rate of 10−3 s−1, with loading applied along the extrusion direction. The microstructure of the compression samples was characterized using a Zeiss Field Emission Scanning Electron Microscope (Sigma 500) equipped with an EBSD detector. Transmission electron microscopy (TEM) samples were mechanically polished followed by ion-milling using Gatan PIPS691 at −120°C. TEM was performed in a FEI Tecnai G2 using an accelerating voltage of 200 kV. TEM samples were also analyzed by transmission Kikuchi diffraction (TKD) in a field-emission gun scanning electron microscope (SEM) operating at 30 kV, with a step size of 0.2 µm. To provide a more comprehensive background, we have included additional analyses on the tensile deformation of as-extruded Mg-1Al-1.8Gd (wt.%) alloy in Appendix A.
3. Results and discussion
Figure shows the representative compressive engineering stress–strain curve of the as-extruded Mg-Al-Gd alloy. The compressive yield strength (CYS) and ultimate compressive strength (UCS) of the as-extruded alloy are ∼160 MPa and ∼400 MPa, respectively. Notably, the UCS of the low-alloyed Mg-Al-Gd alloy closely rivals that of the high-alloyed WE43 alloy [Citation19]. Moreover, the plasticity of the Mg-Al-Gd alloy reaches ∼18%, which is much higher in comparison to other Mg alloys either containing RE elements or not, e.g. AZ31 (wt.%) alloy (∼14%) [Citation20], AZ80-3(Y, Gd) (wt.%, AEZ830) alloy (∼13%) [Citation21], Mg-3Al-5Bi (wt.%, BA53) alloy (∼13.5%) [Citation22] and Mg-8Gd-4Y-1Zn-Mn (wt.%, E128N) alloy (∼13.2%) [Citation23] reported in literature. To reveal the underlying mechanisms for the high plasticity, compression testing was interrupted at various strains, i.e. 0%, ∼8% and ∼14%, for microstructure observation using EBSD, as indicated by blue pentagonal markers. The representative inverse pole figure (IPF) maps (Figure (a–c)) and corresponding kernel average misorientation (KAM) maps (Figure (d and f)) are presented. The as-extruded alloy exhibits a heterogeneous grain structure, including elongated grains along the ED and equiaxed grains. Extension and contraction twins are seldom discovered (Figure (a and d)). After compressed by ∼8%, multiple lenticular-shaped twins with a boundary misorientation angle of ∼86° (highlighted in red) have formed, which are identified as extension twins (Figure (b and e)) that are similar to the observations in previous studies [Citation24,Citation25]. Moreover, a few twins with a boundary misorientation angle of ∼56° about
axes (highlighted in orange-yellow) are observed in several grains of the 8%-strained sample. It is interesting that these boundaries with a misorientation angle of ∼56° about
axes have developed in addition to the frequently observed
twin boundaries, which is inferred to be a new type of twins (Figure (c and f)). A detailed statistical analysis of the area fraction and grain boundary length occupancy of uncommon twins shows that they are ∼6.8% and ∼13%, respectively.
Figure 1. Typical compressive stress-strain curve of the as-extruded Mg-Al-Gd alloy. IPF maps, KAM maps (overlaid with special boundaries by red and orange-yellow lines) and pole figures of the as-extruded alloy with various compression strains: (a, d and g) 0%, (b, e and h) ∼8% and (c, f and i) ∼14%.
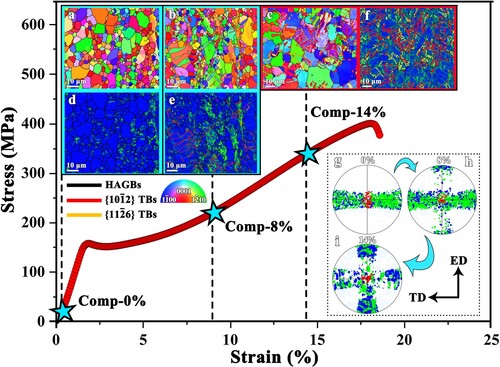
Moreover, the main texture component in pole figures (PFs) under the strain of 0∼14% reflects the orientation dependence of the deformation mechanisms (Figure (g–i)). At ϵ = 0 (Figure (g)), fairly strong preferred orientations, i.e. the (green) and
(blue), are nearly perpendicular to ED. As the strain increases (ϵ = ∼8% and ϵ = ∼14%), the formation of ED texture components is mainly attributed to the twinning [Citation26,Citation27] (Figure (h and i)).
An enlarged view of a representative region from Figure (c) is shown in Figure , which indicates that twins can be classified into two types based on boundary misorientation angles: (i) ∼86° (highlighted in red) and (ii) ∼56° (highlighted in orange-yellow) in the IPF and KAM maps (Figure (a and b)). Several grains containing twins, such as A, B, C and D in Figure (b), are depicted in Figure (c) together with their corresponding PFs. Within grains A and B, twin boundaries (TBs) delineated by ∼56° about axes are identified; whereas grains C and D display two characteristic misorientation relationships: one at ∼86° about
axes and the other at ∼56° about
axes. Additionally, the misorientation angle (θ) distributions derived from Figure (a) are shown in Figure (d), where the presence of a peak at ∼86° about
axes are attributed to
TBs [Citation28]. The concentration of misorientation angles at ∼56° forms mainly owing to the ∼56°
twins. Also, the changes in crystal orientations during compression can be macroscopically reflected on the PFs (Figure (e)).
Figure 2. Typical EBSD result of the as-extruded Mg-Al-Gd alloy compressed by ∼14%: (a) IPF map. (b) Corresponding grain boundary map. (c) Enlarged IPF maps for grains A, B, C, and D with corresponding PFs. (d) Misorientation angle distribution maps corresponding to (a) and (e) Pole figures corresponding to (a): (red),
(green) and
(blue).
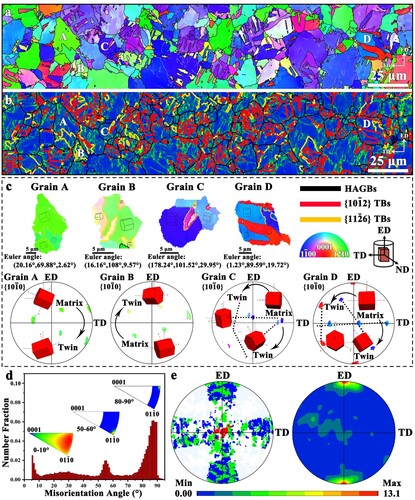
We also analyzed the two twin types using TKD and TEM (Figure ). The IPF (Figure (a)) and SEM (Figure (b)) micrographs accurately correlate the crystallographic orientations with the microscale morphology of the TKD sample. TEM micrographs (Figure (e–k)) reveal two characteristic twin boundaries with misorientation angles of ∼86° (red) and ∼56° (orange-yellow). Figure (e) shows the bright-field TEM (BF-TEM) image of twin lamella structures. The inset is the selected area electron diffraction (SAED) pattern from both the matrix and twin. Based on the SAED pattern (Figure (e)) and the high-resolution TEM (HRTEM) image with the fast Fourier transform (FFT) patterns (Figure (f)) taken around the twin boundary along the zone axes, the twins are identified as
twins. The crystallographic characteristic of
twin agrees well with previous works [Citation29,Citation30]. Figure (g) demonstrates a low-magnification image of the twin lamella viewed slightly off the
zone axes. The enlarged BF-TEM image in Figure (h), highlights the interface between the twin and the matrix. The corresponding SAED pattern in Figure (i) is derived from the twin boundary in Figure (h). Further analysis reveals that the net reorientation of the basal plane is ∼56° about
axes, and the twin boundary plane belongs to
planes (Figure (j)). Figure (k) shows an HRTEM image from the twin boundary in Figure (h), viewed along the
zone axes. The insets in Figure (k) show the FFT pattern from the matrix and twin, respectively. Combining the results in Figure (i–k), the twin is identified to be
twins. Correspondingly, the multistage twinning mode is unambiguously determined, i.e. from a single
twinning mode at a lower strain level (∼8%) to the co-existence of
and
twins at a higher strain level (∼14%).
Figure 3. TEM and TKD results of two types of twins in the Mg-Al-Gd alloy compressed by ∼14%: (a) TKD orientation map with special boundary misorientation angles: ∼86° (red) and ∼56° (orange-yellow). (b) SEM image corresponding to (a). (c, d) Enlarged images corresponding to the rectangle in (a, b). (e) BF-TEM image of the twin. (f) HRTEM image showing the
TB. (g) BF-TEM image of the
twin. (h) BF-TEM image corresponding to the rectangle in (g). (i) The SAED pattern from both the matrix and
twin. (j) Schematic diagram of the SEAD pattern in (i). (k) HRTEM image showing the
TB.
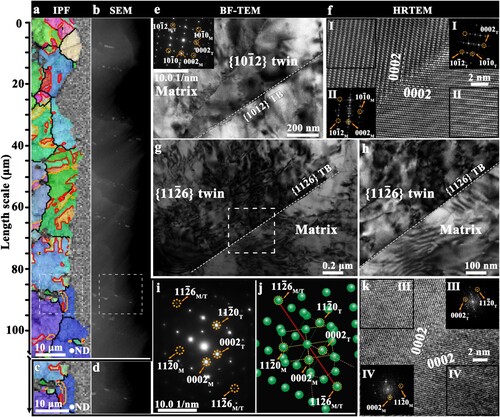
The selection of twinning mode can be determined by Schmid factors (SF) calculations. A continuous loading is assumed to be applied in crystals, where the loading directions of type I and II are along
and
, respectively. The angle ω (the angle between the loading direction and the crystal orientation) was measured to evaluate the variation of SF values for twins. The SF values of
and
twin variants were calculated. Details of SF calculations are described in Supplementary Appendix B & Figure S3.
For Type I, twin variants are more likely to be activated, as the SF values for such twins are larger than 0.3. Note that the maximum SF values of
twin variants are as high as 0.499 in certain ω ranges (−20° < ω < −15°) (Figure (a and b)). For Type II, both
twin variants, and
twin variants (B5 and B6, as shown in Figure (j)) have higher SF values (0.4 < SF < 0.5). Considering that certain deviations could exist between the applied stress and the actual stress states, it is also feasible that the
twins with relatively low SF values could be activated.
Figure 4. Schmid-factor (SF) determined twinning mode selection. The Schmid-factor maps and corresponding orientation maps of twins: (a to c) Higher SF values (0.441–0.499) in Type I. (d to i) Lower SF values in Type I. (j to l) Higher SF values (0.399–0.409) in Type II. (m to r) Lower SF values in Type II.
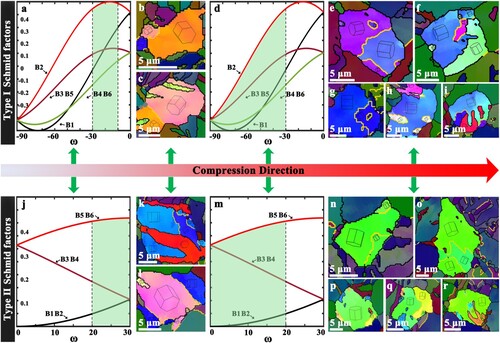
It has been reported that, in HCP Mg alloys, the easily activated twin with the Burgers vector
and the shear strain
of 0.129
[Citation31] can provide a lattice strain of ∼6.65%. In the present work, a maximum lattice strain along c-axes due to the
twins with the Burgers vector
, is estimated to be ∼5.2%. Theoretically, the coexistence of
and
twins can provide a maximum strain higher than that provided by either the single
or
twinning mode. Thereby, the co-existence of
or
twinning could play an essential role in strain accommodation along c-axes.
From TKD characterizations (Figure (a)), we can observe that a typical grain contains a twin. This facilitates the accommodation of deformation incompatibilities at grain boundaries [Citation32] and leads to a redistribution of local stresses [Citation33,Citation34]. To further elaborate the influence of the
twins on dislocation slips, the theoretical SFs of slip systems (SS) are considered for both matrix and
twins. As shown in Table , the maximum SF value of dislocation slip systems in the parent grain belongs to basal-
slips (
= 0.45, SS1), whereas the maximum SF value in the
twin belongs to pyramidal-
dislocations (
= 0.48, SS13). Therefore, it is reasonable to hypothesize that non-basal slip might be activated within
twins.
Table 1. Calculated Schmid factors of the eighteen slip systems in Parent grain (Euler angle: 125°, 86°, 17°) and the twin (Euler angle: 84°, 121°, 8°).
The twins have been further investigated by TEM. Figure (b) presents a BF-TEM image showing a
twin, viewed along the
zone axes. Based on the invisibility criterion, dislocation analysis reveals the activation of multiple slip systems, including high-density basal-
(red arrow), non-basal-
(green arrow) and pyramidal-
(blue arrow) dislocations within the
twin. Herein, the
dislocations can be determined by analyzing various two-beam conditions, i.e. g =
and g =
, as shown in Supplementary Figure S4. High density of
dislocations is observed under g =
, while dislocations with
-type Burgers vectors are clearly discerned at the same location under the g =
condition. Cross-slips between basal and non-basal planes are also observed (Figure (b)), evidenced by wavy slip lines [Citation35,Citation36]. Generally, the activation of non-basal slips is much more difficult than the basal-
slips in Mg alloys due to the higher CRSS [Citation37,Citation38]. The activated non-basal-
slip systems with lower SF values (m < 0.3) and pyramidal-
slips inside the
twins are mainly attributed to (i) the stacking-fault energy reduction caused by the Gd addition [Citation39,Citation40] and (ii) the changed parent grain orientation by twinning to a favorable orientation for activating non-basal slips.
The activated multiple slip systems within twins that satisfy the Von Mises criteria [Citation41], enable to coordinate the complex plastic deformation along both the a and c-axes, effectively promoting isotropic deformation and reducing the likelihood of premature fracture at grain boundaries [Citation42]. Additionally, the mean free paths of dislocations are confined by the
twin boundaries, leading to a strong tendency for tangling of dislocations inside the twin and long-range back stresses [Citation43,Citation44] at twin boundaries (Figure (b)). These factors contribute to sustained strain hardening and enhanced plasticity. The formation mechanisms associated with the
twin are detailed in Appendix C.
4. Conclusions
In summary, a novel multistage twinning mode has been identified in a Mg-Al-Gd alloy subjected to compressive deformation at room temperature. Correlative EBSD and TEM analysis reveal that the dominant twinning mode changes from twins to the co-existence of
and
twins. Furthermore, the interactions of high-density pyramidal-
and non-basal-
dislocations and dense twin boundaries contribute to the high strain hardening. These findings are expected to shed some new insights into deformation mechanisms of Mg alloys, which are useful for designing high-performance Mg alloys.
Supplemental Material
Download MS Word (4.5 MB)Disclosure statement
No potential conflict of interest was reported by the author(s).
Additional information
Funding
References
- Shi Q, Natarajan AR, Van der Ven A, et al. Partitioning of Ca to metastable precipitates in a Mg-rare earth alloy. Mater Res Lett. 2022;11(3):222–230. doi:10.1080/21663831.2022.2138724
- Zhu G, Wang L, Wang J, et al. Highly deformable Mg-Al-Ca alloy with Al2Ca precipitates. Acta Mater. 2020;200:236–245. doi:10.1016/j.actamat.2020.09.006
- Wang JY, Li N, Alizadeh R, et al. Effect of solute content and temperature on the deformation mechanisms and critical resolved shear stress in Mg-Al and Mg-Zn alloys. Acta Mater. 2019;170:155–165. doi:10.1016/j.actamat.2019.03.027
- Zha M, Ma X, Jia H-L, et al. Dynamic precipitation and deformation behaviors of a bimodal-grained WE43 alloy with enhanced mechanical properties. Int J Plast. 2023;167:103682. doi:10.1016/j.ijplas.2023.103682
- Sahoo SK, Biswas S, Toth LS, et al. Strain hardening, twinning and texture evolution in magnesium alloy using the all twin variant polycrystal modelling approach. Int J Plast. 2020;128:102660. doi:10.1016/j.ijplas.2020.102660
- Sahoo SK, Toth LS, Biswas S. An analytical model to predict strain-hardening behaviour and twin volume fraction in a profoundly twinning magnesium alloy. Int J Plast. 2019;119:273–290. doi:10.1016/j.ijplas.2019.04.007
- Gong MY, Xu S, Jiang YY, et al. Structural characteristics of 10–12 non-cozone twin-twin interactions in magnesium. Acta Mater. 2018;159:65–76. doi:10.1016/j.actamat.2018.08.004
- Zhu YT, Liao XZ, Wu XL. Deformation twinning in nanocrystalline materials. Prog Mater Sci. 2012;57(1):1–62. doi:10.1016/j.pmatsci.2011.05.001
- Gong M, Hirth JP, Liu Y, et al. Interface structures and twinning mechanisms of twins in hexagonal metals. Mater Res Lett. 2017;5(7):449–464. doi:10.1080/21663831.2017.1336496
- Hu Y, Turlo V, Beyerlein IJ, et al. Disconnection-mediated twin embryo growth in Mg. Acta Mater. 2020;194:437–451. doi:10.1016/j.actamat.2020.04.010
- Yaddanapudi K, Leu B, Kumar MA, et al. Accommodation and formation of 10–12 twins in Mg-Y alloys. Acta Mater. 2021;204:116514. doi:10.1016/j.actamat.2020.116514
- He C, Li Z, Kong D, et al. Origin of profuse 11–21 deformation twins in Mg-Gd alloys. Scr Mater. 2021;191:62–66. doi:10.1016/j.scriptamat.2020.08.041
- Zhang H, Li Y, Ding Z, et al. Origin of twin-like 33–64 tilt boundary and associated solute segregation in a high strain rate deformed Mg-Y alloy. Scr Mater. 2021;201:113982. doi:10.1016/j.scriptamat.2021.113982
- Cayron C. The (11–22) and (-12–16) twinning modes modelled by obliquity correction of a (58°, a+2b) prototype stretch twin. Acta Crystallogr. 2018;74:44–53.
- Andriy Ostapovets JB, Krahula K, Král L, et al. On the relationship between 11–26 and 11–22 conjugate twins and double extension twins in rolled pure Mg. Philos Mag A. 2017;97:1088–1101. doi:10.1080/14786435.2017.1290846
- Gao Y, Zhao L, Zha M, et al. Twinning-induced plasticity with multiple twinning modes and disclinations in Mg alloys. Int J Plast. 2023;164:103595. doi:10.1016/j.ijplas.2023.103595
- Bilby BA, Crocker AG. The theory of the crystallography of deformation twinning. Royal Soc. 1965;288:240–255.
- YOO MH. Slip, twinning, and fracture in hexagonal close-packed metals. Metall Trans A. 1981;12A:409–418.
- Jahedi M, McWilliams BA, Moy P, et al. Deformation twinning in rolled WE43-T5 rare earth magnesium alloy: influence on strain hardening and texture evolution. Acta Mater. 2017;131:221–232. doi:10.1016/j.actamat.2017.03.075
- Ghaderi A, Barnett MR. Sensitivity of deformation twinning to grain size in titanium and magnesium. Acta Mater. 2011;59(20):7824–7839. doi:10.1016/j.actamat.2011.09.018
- Bu F, Yang Q, Qiu X, et al. Study on the assemblage of Y and Gd on microstructure and mechanical properties of hot extruded Mg-Al-Zn alloy. Mat Sci Eng a-Struct. 2015;639:198–207. doi:10.1016/j.msea.2015.05.008
- Go J, Lee JH, Yu H, et al. Significant improvement in the mechanical properties of an extruded Mg–5Bi alloy through the addition of Al. J Alloy Compd. 2020;821:153442. doi:10.1016/j.jallcom.2019.153442
- Wang K, Wang J, Huang S, et al. Formation of an abnormal texture in Mg-Gd-Y-Zn-Mn alloy and its effect on mechanical properties by altering extrusion parameters. Mat Sci Eng a-Struct. 2022;831:142270. doi:10.1016/j.msea.2021.142270
- Gengor G, Mohammed ASK, Sehitoglu H. 10–12 twin interface structure and energetics in HCP materials. Acta Mater. 2021;219:117256. doi:10.1016/j.actamat.2021.117256
- Li Z, Zhang J, Zhai Y, et al. Dynamic mechanisms of strengthening and softening of coherent twin boundary via dislocation pile-up and cross-slip. Mater Res Lett. 2022;10(8):539–546. doi:10.1080/21663831.2022.2065892
- Jeong J, Alfreider M, Konetschnik R, et al. In-situ TEM observation of 10–12 twin-dominated deformation of Mg pillars: twinning mechanism, size effects and rate dependency. Acta Mater. 2018;158:407–421. doi:10.1016/j.actamat.2018.07.027
- Ma L, Xie K, Cai J, et al. Non-dissociated <c+a> dislocations in an AZ31 alloy revealed by transmission electron microscopy. Mater Res Lett. 2020;8(4):145–150. doi:10.1080/21663831.2020.1721586
- Lu SH, Wu D, Chen RS, et al. Reasonable utilization of 10–12 twin for optimizing microstructure and improving mechanical property in a Mg-Gd-Y alloy. Mater Des. 2020;191:108600. doi:10.1016/j.matdes.2020.108600
- Han G, Noh Y, Chaudry UM, et al. 10–12 extension twinning activity and compression behavior of pure Mg and Mg-0.5Ca alloy at cryogenic temperature. Mat Sci Eng a-Struct. 2022;831:142189. doi:10.1016/j.msea.2021.142189
- Zhou X, Su H, Ye H, et al. Removing basal-dissociated <c+a> dislocations by 10–12 deformation twinning in magnesium alloys. Acta Mater. 2021;217:117170. doi:10.1016/j.actamat.2021.117170
- Molodov KD, Al-Samman T, Molodov DA, et al. On the twinning shear of 10–12 twins in magnesium—experimental determination and formal description. Acta Mater. 2017;134:267–273. doi:10.1016/j.actamat.2017.05.041
- Meyers MA, Vohringer O, Lubarda VA. The onset of twinning in metals: a constitutive description. Acta Mater. 2001;49(19):4025–4039. doi:10.1016/S1359-6454(01)00300-7
- Capolungo L, Marshall PE, McCabe RJ, et al. Nucleation and growth of twins in Zr: a statistical study. Acta Mater. 2009;57(20):6047–6056. doi:10.1016/j.actamat.2009.08.030
- Orozco-Caballero A, Lunt D, Robson JD, et al. How magnesium accommodates local deformation incompatibility: a high-resolution digital image correlation study. Acta Mater. 2017;133:367–379. doi:10.1016/j.actamat.2017.05.040
- Agnew SR, Duygulu Ö. Plastic anisotropy and the role of non-basal slip in magnesium alloy AZ31B. Int J Plast. 2005;21(6):1161–1193. doi:10.1016/j.ijplas.2004.05.018
- Koike J, Kobayashi T, Mukai T, et al. The activity of non-basal slip systems and dynamic recovery at room temperature in fine-grained AZ31B magnesium alloys. Acta Mater. 2003;51(7):2055–2065. doi:10.1016/S1359-6454(03)00005-3
- Ovri H, Markmann J, Barthel J, et al. Mechanistic origin of the enhanced strength and ductility in Mg-rare earth alloys. Acta Mater. 2023;244:118550. doi:10.1016/j.actamat.2022.118550
- Wang T, Zha M, Du C, et al. High strength and high ductility achieved in a heterogeneous lamella-structured magnesium alloy. Mater Res Lett. 2022;11(3):187–195. doi:10.1080/21663831.2022.2133976
- Li RG, Yan Y, Pan HC, et al. Achieving a high-strength binary Mg-15Gd alloy by nano substructure with Gd segregation and nano clusters. Mater Res Lett. 2022;10(10):682–689. doi:10.1080/21663831.2022.2086834
- Nie JF, Zhu YM, Liu JZ, et al. Periodic segregation of solute atoms in fully coherent twin boundaries. Science. 2013;340:957–960. doi:10.1126/science.1229369
- Graff S, Brocks W, Steglich D. Yielding of magnesium: from single crystal to polycrystalline aggregates. Int J Plast. 2007;23(12):1957–1978. doi:10.1016/j.ijplas.2007.07.009
- Ogawa Y, Singh A, Somekawa H. Activation of non-basal <c+a> slip in coarse-grained Mg-Sc alloy. Scr Mater. 2022;218:114830. doi:10.1016/j.scriptamat.2022.114830
- Basu I, Chen M, Wheeler J, et al. Segregation-driven exceptional twin-boundary strengthening in lean Mg-Zn-Ca alloys. Acta Mater. 2022;229:117746. doi:10.1016/j.actamat.2022.117746
- Jia Y, Jiang S, Tan J, et al. The evolution of local stress during deformation twinning in a Mg-Gd-Y-Zn alloy. Acta Mater. 2022;222:117452. doi:10.1016/j.actamat.2021.117452