Abstract
Plumbagin (PLB), a member of the quinine family, mainly found in the plant Plumbago zeylanica Linn., potentially exhibit anticancer, anti-inflammatory, anti-oxidant, antifungal, neuroprotective, hypolipidemic and antibacterial activities. However, it has been well known that the application of PLB was limited owing to its water insolubility, instability and poor bioavailability. For decades, many attempts have been made to compensate for these disadvantages with the development of improved delivery platforms as the feasible approaches. This review aims to describe the various studies supporting the biopharmaceutical aspects of PLB. In addition, it includes a section devoted to discussing the challenges associated with the drug and strategies to improve the properties of PLB such as solubility, stability and bioavailability. Also, this paper summarizes the recent works on the design and development of novel delivery systems of PLB such as liposomes, niosomes, microsphares, nanoparticles, micelles, complexization, metal nanoparticles, crystals modification, etc., with the goal of harnessing the true difficulties of this multifunctional agent in the clinical arena.
Introduction
In modern drug discovery era, various chemically synthesized new molecules are approved by FDA and are coming on the market, having wide therapeutic efficacy but this therapy causes serious adverse effects which could be life threatening. Conventional therapy provides non-targetability in tissues and organs due to peak and valley fluctuations of plasma drug concentration, and a frequent dose of administration can produce troublesome for allopathic medicines lead to poor patient compliance. In ancient times, herbal remedies and natural extract are consumed by people to cure various diseases. These herbal remedies contain hundreds of phytoconstituents which is working simultaneously against the disease. In recent times, the interest of people in phytopharmaceuticals has been increasing day-by-day among physicians and patients, and it is evident from the global market of herbal medicine and phytopharmaceuticals that has increased from $26 billion from 2011 and $32 from 2016 [Citation1]. Various dietary products and supplements are also derived from the natural origin are also gaining more interest in the industry and the global market for phytopharmaceuticals. Some phytoconstituents derived from the natural origin are having a poor solubility and low bioavailability resulting in a narrow therapeutic index which hinders its novel efficiency, so formulation scientist is working on targeting and controlled drug release of phytoconstituents to provide better therapeutic effect and increased patient compliance.
Novel drug delivery systems for phytomedicine
In the past few decades, considerable attention has been focused on the development of novel drug delivery system (NDDS) for phytoconstituents. The novel carriers should ideally fulfil two prerequisites. Firstly, it should deliver the drug at a rate directed by the needs of the body over the period of treatment. Secondly, it should channel the drug to the site of action. Conventional dosage forms including prolonged-release dosage forms are unable to meet none of these. In phytoformulation research, developing novel drug delivery system such as polymeric nanoparticles and nanocapsules, liposomes, solid lipid nanoparticles, microemulsion, microspheres and nanoemulsion, etc. have a number of advantages for phytoconstituents, including enhancement of solubility and bioavailability, protection from toxicity, enhancement of pharmacological activity, enhancement of stability, improving tissue macrophages distribution, sustained delivery, protection from physical and chemical degradation, etc. Thus, the novel drug delivery systems of phytoconstituents have a potential future for enhancing the activity and overcoming problems associated with plant medicines [Citation2].
Plumbagin
Plumbagin (PLB), a plant-based secondary metabolite belonging to 1,4-naphthoquinone, is the most important class in the quinone family. It is mainly found in root, leaf and stem bark of some plant families including Plumbaginaceae, Ebenceae, Dioncophyllaceae, Ancestrocladaceae and Droseraceae [Citation3]. This natural component has a number of distinct biological activities including anticancer, anti-inflammatory, anti-oxidant, antifungal, neuroprotective, hypolipidemic and antibacterial activities. Currently, number of researchers have focused on the anticancer properties of PLB on various cancers both in vitro and in vivo, such as breast cancer, pancreatic cancer, lung cancer, prostate cancer, etc. [Citation4]. This review aims to describe the various studies supporting the biopharmaceutical aspects of PLB. In addition, it includes a section devoted to discussing the challenges associated with the drug and strategies to improve the properties of PLB such as solubility, stability and bioavailability. Also, this paper summarizes the recent works on the design and development of novel delivery systems of PLB such as liposomes, niosomes, microsphares, nanoparticles, micelles, complexzation, metal nanoparticles, crystals modification, etc.
PLB chemistry and structural activity relationship
PLB, 5-hydroxy-2-methyl-1, 4-naphthoquinone, is an analogue of vitamin K3. The chemical formula of PLB is C11H8O3 and the molecular weight is 188.18 g/mol. The chemical structure of PLB and its summary are shown in and Box 1.
Figure 2. (A) Schematic presentation of mechanisms for anticancer activities of plumbagin in-vitro. (B) Summary of documented molecular-biological effects of plumbagin.
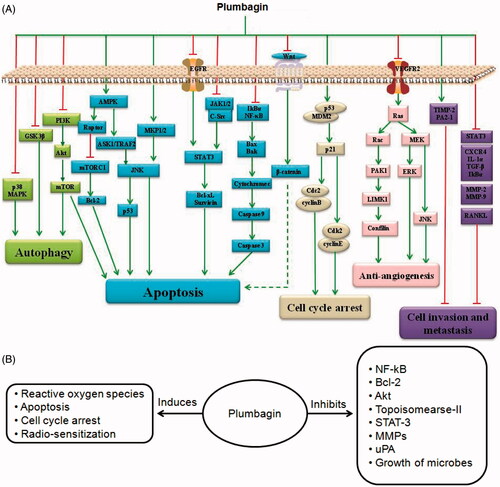
Figure 3. Redesign of plumbagin through various strategies to enhance bioavailability. PL: phospholipid; TPGS: D-a-tocopheryl polyethylene glycol 1000 succinate; FA: folciacid; PLGA: polylactic-co-glycolic acid; β-CyD: betacyclodextrin; PMCA: polymethylcyanoacrylate.
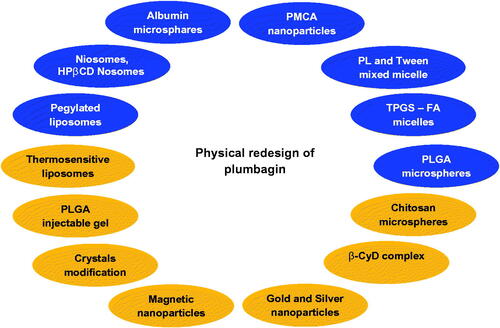
Various synthetic PLB analogues were derived to optimize its bioavailability and pharmacokinetic profiles (). Substitution of N-acetyl-l-amino acid side chain at third position significantly increased antifeedant activity. PLB was demonstrated to be an inhibitor of human DNA topoisomerase II. It was reported that cytotoxicity of compounds containing quinines structure might be due to radical formation via electron transport in the existence of quinine ring. Therefore, cytotoxicity of PLB may be associated with its special structure which contains a quinone ring. The hydroquinonoid, nitro, cyano and methyl ester derivatives of PLB did not show any marked enhancement in the tumour-inhibitory activity [Citation5]. Reduction of naphthoquinone and amide derivatives from 1,4-naphthoquinone carboxylic acid of PLB was shown trypanocidal activity by inhibiting trypanothione reductase (TR) enzyme from Trypanosoma cruzi. PLB homologues (2-alkyl-1,4-naphthoquinones) as well as their 3-methyl derivatives were shown weaker prostaglandin synthetase (PGS)-inhibition activity. In third position halogen substitution shown stronger ichthyotoxicity than free PLB. Thioglycosides and alkylglucoside derivatives showed antibacterial activity by inhibiting N-acetylgluco-saminylinositol (GlcNAc-Ins)-deacetylase (MshB) and mycothiol-S-conjugate amidase (Mca) which are needed for mycothiol synthesis in bacteria. The PLB tethered at C-3 position via 2–5 methylene carbons and an amide linkage to phenyl-2-deoxy-2-amino-1-thio-a-D-glucopyranoside was shown potent inhibitor of MshB. MshB inhibitory activity was shown by large carbon chain length substituted in PLB. The hydroxyl group of PLB increases its electrophilic properties and makes it a more potent cytotoxic molecule via its ability to accept electrons which increases its capacity to generate reactive oxygen species.
Box 1 Drug summary
Drug name: Plumbagin
CAS No: 481-42-5
Chemical name: 2-Methyl-5-hydroxy-1,4-naphthoquinone
Chemical class: 1,4-Naphthoquinone
Molecular formula: C11H8O3
Molecular weight: 188.18 g/mol
Appearance: Orange crystalline powder
Melting point: 78–79 °C
Boling point: 383.9 °C
Log P: 3.04
pKa: 9.48
Half-life: 4 h
Solubility: Poorly aqueous soluble (79.3 μg/ml)
Soluble in methanol, ethanol, DMSO, pyridine, acetic acid and acetone
Bioavailability: 39%.
PLB mechanism of actions
PLB has a wide spectrum of activity ranging from anti-inflammatory to anticancer. is a comprehensive account of the biological activity and mechanism of action of PLB.
Table 1. Biological activities and mechanism of actions of plumbagin.
PLB toxicity
The maximum tolerated doses of PLB in the acute and subacute toxicity testing were 150 (single oral dose) and 25 (daily doses for 28 days) mg/kg body weight, respectively. The 50% lethal dose (LD50) of PLB for the acute and subacute toxicity testing was 250 and 50–100 mg/kg body weight, respectively [Citation6]. PLB is associated with normal tissue toxicity and short biological half-life with rapid elimination in vivo. Being a quinone moiety is reported to be highly toxic and acts as spindle poison by inhibiting cell mitosis at low concentrations and at higher concentrations it exhibits radiomimetic and cytotoxic effects. Literature suggests that the structure of PLB is similar to vitamin K and therefore the chronic administration of PLB leads to prolonged bleeding time by altering platelet adhesiveness and coagulation [Citation20]. Other toxic effects of PLB include diarrhoea, skin rashes, increases in white blood cell and neutrophil counts, increases in serum phosphatase and acid phosphatase levels, hepatic toxicity and cardiotoxicity [Citation21]. In a recent study in mice, hepatotoxicity of PLB through unbalancing of the anti-oxidative system was observed, that is, marked increased plasma ALT and AST levels, hepatic lipid peroxidation and glutathione peroxidise activity; but decreased superoxide dismutase and catalase activities.
Biopharmaceutics of PLB
PLB being a potential candidate with different pharmacological activities has been extensively studied for its biopharmaceutical and pharmacokinetic parameters in different animal model and human volunteers. All the pharmacokinetic parameters, absorption, distribution, metabolism and excretion of PLB are well understood. Permeability of the compound across the human colon epithelial cell monolayer Caco-2 has been shown to be moderate and the transport mechanism is likely to be a passive transport. Oral bioavailability of PLB was 38.775% in Sprague–Dawley rats [Citation22]. After single oral dose (100 mg/kg) administration in mice, PLB (49%) was excreted through faeces, Cmax was 0.35 mg/ml at 1 h after which its declined rapidly, AUC was 271.9 mg/kg with Tmax of 150 min. Moreover, the conjectural metabolites of PLB were found in rat urine. These results indicate that the metabolic pathway of PLB may go through phase-I aliphatic hydroxylation and phase II glucuronidation [Citation22]. In another study (), Sumsakul et al. found that changing the species (Wistar vs. Sprague–Dawley rats) showed significant impact on oral pharmacokinetics of PLB attributed to its limited biopharmaceutical properties such as high lipophilicity (log P 3.04) and insolubility in water () [Citation3]. Chen et al. investigated the effects of PLB on CYP1A2, CYP2B1/6, CYP2C9/11, CYP2D1/6, CYP2E1 and CYP3A2/4 activities in human and rat liver [Citation23]. In humans, PLB was not only a mixed inhibitor of CYP2B6, CYP2C9, CYP2D6, CYP2E1 and CYP3A4, but also a non-competitive inhibitor of CYP1A2 with Ki values no more than 2.16 μM. In rats, the mixed inhibition of CYP1A2 and CYP2D1, and competitive inhibition for CYP2B1, CYP2C11 and CYP2E1 with Ki values less than 9.93 μM.
Table 2. The pharmacokinetic parameters of plumbagin after oral administration of 100 mg/kg body weight of plumbagin to healthy male Wistar rats [Citation3].
PLB delivery systems
Animal studies suggested that orally administered PLB produces only 39% of bioavailability owing to its limited biopharmaceutical properties such as high lipophilicity, insolubility in water, short biological half-life and low melting point [Citation24]. Being a quinine moiety is reported to be highly toxic and acts as spindle poison by inhibiting cell mitosis at low concentrations and by exhibiting radiomimetic and cytotoxic effects at higher concentrations. PLB also has a tendency to cause haemorrhage on chronic administration due to competitive inhibition of vitamin K activity. Considering these limitations, researchers have designed various drug delivery systems to enhance its therapeutic efficacy (). Each of these systems has been discussed in detail in the following sections.
Liposomes
Liposomes are small spherical shape lipoid vesicles fabricated from cholesterol and various natural phospholipids. They help to improve the solubility, bioavailability, intracellular uptake of components, in vitro and in vivo stability, the pharmacokinetics and bio-distribution pattern of active moieties [Citation25]. Biocompatibility, biodegradability, site-specificity, low toxicity and ability to entrap both hydrophobic and hydrophilic molecule are the striking features of liposomes that make them highly favoured in cosmetic and pharmaceutical industries [Citation26]. Literature revealed that numerous liposomal products are available in market and many are in various stages of clinical trials. Apart from above mentioned reasons, flexibility and compatibility were the drawing force for the development of liposomal delivery systems that were expected to overcome the problems associated with therapeutic potential of PLB.
Tiwari et al. formulated PLB-loaded thermosensitive small unilamellar liposomes comprising dipalmitoyl phosphatidylcholine (DPPC) and distearoyl phosphatidylcholine (DSPC) using thin film hydration method [Citation27]. The liposome size and encapsulation efficiency were of 0.19 nm and 19%, respectively. The results of the in vitro release studies for liposomes at various temperatures indicated that the maximum release (42%) occurred at 42 °C and less than 5% release occurred at temperatures less than 37 °C. At high temperatures, the bilayer of the liposomes was in the liquid crystalline fluid state (disordered fluid state) and drug entrapped in lipid phase of the vesicles was easily migrating to the bulk aqueous phase. At physiological temperature (37 °C), the bilayer was in a crystalline solid state (ordered gel state) which prevented the leakage of the entrapped drug to bulk aqueous phase. The maximum tumour (melanoma B16F1) response in terms of volume doubling time and growth delay was observed in C57BL/6J mice when combined with localized hyperthermia treatment was applied for 1 h in combination with thermosensitive PLB liposomes. Significant increase in volume doubling time and growth delay was observed when liposomal PLB + hyperthermia treatment (1 h) was compared to liposomal PLB + hyperthermia treatment (30 min) or to free PLB + hyperthermia treatment (30 min or 1 h).
Sunil Kumar et al. formulated PLB-loaded long circulating pegylated liposomes by thin film hydration method and subsequently size reduction by high pressure homogenization to enhance PLB plasma half-life and anticancer potential using B16F1 melanoma as solid tumour model in vivo [Citation28]. The developed formulation showed encapsulation efficiency, particle size, zeta potential and cumulative drug release were of 52.51%, 123.95 nm, −23.4 mV and 58.27% in 24, respectively. Also, it exhibited 36.38- and 3.13-fold improvement in plasma half-life and bioavailability with significant (p < .001) reduction in the tumour volume in B16F1 melanoma cells growth in vivo in C57BL/6l mice as compared to free PLB. It is attributed that the pegylation process leads to the formation of hydrophilic coat on the liposomal surface and there by repel opsonin penetration and adsorption [Citation29]. This reduced marking by the opsonins leads to slower uptake of the pegylated liposomes by the cells of RES in comparison to the conventional liposomes and hence extended systemic circulation half-life and bioavailability leading to increased local concentration of PLB at the tumour site via enhanced permeability and retention (EPR) effect. No signs of normal tissue toxicity in both the free PLB and pegylated liposomal treated group indicating its non-toxic nature.
Niosomes
Niosomes are nonionic surfactant based vesicles formed by the self-assembly of nonionic amphiphiles in aqueous media resulting in closed bilayer structures. Niosomes have been prepared from different classes of nonionic surfactants such as polyoxyethylene alkyl ethers, sorbitan monoesters (Span 20, 40, 60 and 80) and polyoxyethylene sorbitan monoesters (Tween 20, 60, 61 and 80) [Citation30]. Cholesterol added to the nonionic surfactant provides rigidity and orientation order to the niosomal bilayer. Niosomes have many advantages such as better patient compliance and therapeutic effect than conventional oily formulations, high capability of entrapping hydrophilic, lipophilic as well as amphiphilic drugs, more stable than liposomes. Besides, it can also protect the drugs from biological enzymes and deliver targeting drugs to the certain organs [Citation30].
D’souza et al. loaded the inclusion complex of PLB with hydroxyl propyl betacyclodextrin (HPβCD) in the aqueous layer of niosomes and compared with niosomes of the plain PLB loaded in lipid layer [Citation31]. The niosomes were unilamellar and spherical in shape with size and entrapment efficiency of 25 nm and 68%, respectively. Complex niosomes showed 40% cumulative release for 6 h with neither a burst effect nor a very slow release pattern. Due to the inclusion of drug in aqueous layer the release rats were faster compared to 27% cumulative release of plain drug where entrapment was in lipid layer. The slower release of PLB from the lipid layer attributed to slow portioning and diffusion of PLB from the lipid layer to the surrounding aqueous layer. However, when the drug was incorporated in the aqueous layer, the drug diffused out due to concentration gradient as well as the erosion and biodegradation of lipid layer. The complex-loaded niosomes showed promising antifertility activity as compared plain PLB-loaded niosomes at a dose of 5 mg/kg intraperitoneal route.
Oommen et al. prepared PLB-beta cyclodextrin (β-CyD) complex loaded into the aqueous layer of niosomes by lipid layer hydration method using cholesterol, Span 60 and dicetyl phosphate [Citation32]. The prepared niosomes were of spherical or occasionally oval in shape with diameter and entrapment efficiency of 12 mm and 74%, respectively. The PLB-β-CyD complex, being hydrophilic, released out at a higher rate initially by diffusion and then by erosion of the lipid bilayers from niosomes. Anti-tumour activity was more pronounced for niosomal PLB-β-CyD complex when given subcutaneously as compared free PLB in C57BL/6J mice bearing melanoma B16F1. Naresh et al. developed PLB loaded niosome using a lipid layer hydration method [Citation33]. The niosomal PLB showed better anti-tumour activity in a solid tumour (sarcoma-180) and Ehrlich ascites model in terms of LD50 survival studies and study of tumour volume doubling time with less toxicity than free PLB. Kini et al. formulated controlled release PLB-loaded niosomes and albumin microsphares with a view to increase efficiency and reduce toxicity of PLB [Citation34]. At a dose of 5 mg/kg, ip the albumin microspheres showed promising anti-tumour when tested against B16F1 melanoma grown in vivo and antifertility activity as compared to the niosomes on control. Animal survival data also indicated slight improvement in survival rate and thus anti-tumoural activity. Also, the antifertility activity was affected through an antiovulatory action as evidence from histopathological studies.
Polymeric delivery system
Polymeric nanoparticles
Polymeric nanoparticles are colloidal solid particles prepared from variety of biodegradable polymers and their co-polymers. Commonly used polymers are chitosan and collagen or non-biodegradable polymers such as poly(lactic acid) (PLA) and poly(lactic co-glycolic acid) (PLGA) [Citation35]. These multi-functionalized polymeric material yield micelles, nanocapsules, nanospheres, dendrimers, core–shells, nanoparticle incorporated polymer matrices, etc. Their small size (50–300 nm) allows the particles to penetrate capillaries and to be taken up by the cells, increasing the accumulation of the drug at the target site of action [Citation36].
Indhumathi et al. prepared PLB-loaded polymethylcyanoacrylate nanoparticles using emulsion polymerization in continues aqueous phase method [Citation37]. The nanopaertidle surface was treated with polymer such as PEG 4000, Tween 80 and span 80. The particle size and entrapment efficiency were of <100 nm and 71.63%, respectively. They claimed that the emulsion polymerization in continuous aqueous phase is a better method of choice for preparation of nanoparticles.
Polymeric micelles
Bothiraja et al. fabricated Phospholipid and Tween® 80 mixed micelles by self-assembly method and investigated as an effective injectable nanocarrier for PLB with the aim to improve anticancer efficiency [Citation38]. The mixed micelles were spherical and 46 nm in size. Zeta potential, drug loading and encapsulation efficiency were of 5.04 mV, 91.21 and 98.38% respectively. Micelles demonstrated sustained release and caused a 2.1-fold enhancement in vitro anti-tumour activity of PLB towards human breast cancer MCF-7 cells than its free form. Micelles proved safe for intravenous injection as PLB was stable at high pH; micelle size and encapsulation efficiency were retained upon dilution.
Loading of PLB into D-α-tocopheryl polyethylene glycol 1000 succinate (vitamin E TPGS1k or TPGS) – folic acid conjugates nanomicelles (denote as PTFM) can achieve controlled and targeted delivery with synergistic anticancer potency and reduces PLB toxicity. Loading of PLB into TPGS micelles without folic acid conjugates (denote as PTM) and PTFM shows smaller in size and good encapsulation efficiency. PTM and PTFM increase 3.8- and 4.8-fold PLB bioavailability with long circulation time, slower plasma elimination and no sign of blood and tissue toxicity as compared to free PLB. Micelles demonstrate higher in vitro anticancer activity in folate over expressed human breast cancer MCF-7 cells. PTFM shows targeting effect. Concentration of the drug need for growth inhibition of 50% cells in a designed time period (GI50) is 13.15 ± 1.31 μg/ml for PLB while it decrease by 40.68% for the PTM. Furthermore, the GI50 value of PTFM is 3.2 ± 0.4 μg/ml, that is, a 75.67% decrease as compared to free PLB. A synergistic effect shows by TPGS and PLB. A new concept that the polymeric micellar drug delivery system where a carrier having therapeutic effects brings about reduction in dose as well as cost [Citation39].
Microparticulate system
Microparticles are small solid particles within the size range of 1–200 μm. Depending upon the method of preparation, the drug is dissolved, entrapped and encapsulated to the microparticle matrix. The microparticles offer a variety of opportunities such as protection and masking of entrapped dug, reduced dissolution rate, facilitation of handling and spatial targeting of the active ingredient [Citation40]. From last few years, polymeric microparticles with different geometries have gained numerous interesting applications in biomedical science, especially drug delivery. Usually, microparticles consist of a heterogeneous distribution of different polymers together with encapsulated agents integrated inside a polymeric shell layer. Based on the ultimate application, different hydrophobic and hydrophilic polymers can be utilized during the fabrication process [Citation40].
Singh et al. formulated PLB-loaded poly(d,l-lactide)-co-glycolide (PLGA) microspheres by a solvent evaporation method [Citation41]. The microspheres were smooth and spherical without aggregation. The mean particle diameter and entrapment were of 13.84 nm and 70%, respectively. Microspheres showed triphasic drug release patterns for about four weeks. The initial burst effect was attributed to the release of the drug located near or on the surface of the microspheres. The second phase in which drug release was slow due to the release medium being diffused slowly into the polymer matrix, whereby degradation occurred and drug diffused out into the medium. The final stage (the secondary burst effect) occurred when the matrix became water-soluble which resulted in the erosion of the matrix and collapse of the microsphere. The toxicity of PLB was reduced in mice after subcutaneous injection. The volume doubling time of PLGA-loaded microspheres was significantly higher compared with the plain PLB in sarcoma-I80 tumour bearing BALB/c mice.
Mandala et al. developed intramuscular injectable chitosan-based PLB-loaded microspheres by emulsion cross-linking method using glutaraldehyde (GA) as a cross-linking agent [Citation42]. The chitosan microspheres were spherical, porous outer surface with size and encapsulation efficiency of 106.35 μm and 80.12%, respectively. Microspheres showed sustained release with 96.72% of cumulative release for up to 48 h and followed anomalous (non-fickian) transport. It showed 22-, 25- and 9-fold improvement in half-life, mean residence time and bioavailability with enhanced anti-tumour efficacy of PLB with reduced systemic toxicity as compared to free PLB.
Cyclodextrin complexes
Cyclodextrins (CDs) are cyclic oligosaccharides with a hydrophilic outer shell and a lipophilic cavity in the centre. The α, β and γ are the three most commonly used forms of CDs. Bio-adaptability and multi-functional properties of CDs help to improve the solubility, bioavailability, tolerability and also to eliminate negative side-effects of drugs [Citation43]. CD complexes can act as hosts for a wide variety of lipophilic drugs and are a versatile platform for novel drug delivery systems.
Singh and Udupa prepared inclusion complex of PLB with betacyclodextrin (1:1 molar ratio) employing neutralization method. The LD50 for the plain PLB and the complex were found to be 8.51 and 12.88 mg/kg body weight, respectively. The complex reduced toxicity and enhanced anti-tumour efficacy of PLB. The enhanced anti-tumour efficacy of complex at similar doses compared to plain PLB again indicated that complexation increased the solubility of PLB so that it got absorbed faster in systemic circulation to have a better anti-tumour activity.
Metal nanoparticles
Srinivas et al. synthesized gold nanoparticles conjugated with PLB (AuPB) and compared anticancer activity and reactive oxygen species generation ability of PLB with bromoderivatives of 1,4 naphthoquinones such as 2-bromo-1,4-naphthoquinone (2-BNQ) and 2,3-dibromo-1,4-naphthoquinone (2,3-DBNQ) and their gold nanoconjugates [Citation44]. PLB and bromoderivatives showed reduced cytotoxicity and apoptosis compared with the pristine compounds. Chemical bonding between PLB and Au affected the hydroxyl group of PLB, that is, the electrophilic moiety, made it less toxic molecule, resulted in an ability to quench reactive oxygen species generating capacity of PLB.
Appadurai and Rathinasamy synthesized colloidal silver nanoparticles by chemical reduction method and complexed with PLB (PLB-AgNPs) in a single incubation step [Citation45]. The metal nanoparticles showed particle size, zeta potential and encapsulation efficiency of 32 ± 8 nm, −46.7 mV and 50%, respectively. PLB-AgNPs enhanced internalization of PLB, inhibited proliferation of human cervical cancer cells HeLa with IC50 value of about 18 ± 0.6 μM by inducing mitotic block in a concentration-dependent manner.
Hafeez et al. developed PLB-loaded magnetic nanoparticle (MNP-PLB) formulation composed of an iron oxide core with particle size and zeta potential of 125 nm and −10.79 mV. MNP-PLB exhibited efficient (fourfold) internalization in human PanCa cells (HPAF-II, AsPc1 and Panc-1) with fourfold inhibition in colony formation compared to free PLB [Citation46]. MNP-PLB treatment more efficiently inhibited oncogenic CXCL12/CXCR4 signalling pathway in both PanCa and patient derived stromal fibroblast cells. Also, it showed decreased expression of CXCR4 protein levels in PanCa cells. Moreover, MNP-PL treatment inhibited stromal derived factor 1 (SDF-1)/CXCL12 expression in stromal fibroblasts as compared to free PLB.
Shahida Parveen et al. developed nanoplumbagin (NPn) encapsulated with cetyltrimethylammonium bromide or β-cyclodextrin using ultrasonication technique [Citation47]. NPn was spherical in shape with tunable size between 75 and 110 nm with narrow particle size distribution. DNA cleavage, electrochemical, circular dichroism and electronic absorption studies revealed that PLB and Npn were partially intercalated to the herring sperm DNA, in that Npn intercalated with DNA more significantly compared to the commercial PLB attributed to its smaller in size with enhanced solubility of PLB in aqueous medium.
Caging of PLB in silver nanoparticles imparted selectivity and sensitivity to PLB for selective killing of cancer cells due to the cumulative expression of the properties of PLB and nanoparticles which specifically affected the differential cancer cell microenvironment by altering the pyruvate kinase activity that regulates the ROS challenge in cancerous cells. The positive surface charge of PLB caged silver nanoparticles (PCSN) aids in getting them targeted towards anionic cancerous cells due to the exposed terminal carboxyl group of sialic acid residues [Citation48].
Miscellaneous
Bothiraja et al. prepared surface modified PLB crystals by cold recrystallization technique using a variety of polar and non-polar solvents [Citation49]. Platy crystals, the most significant forms, obtained from cyclohexane possessed small size (62.93 ± 3.74 μm), higher bulk density (0.108 ± 0.014 g/ml) and lower enthalpy of fusion (ΔH 62.62 ± 3.67 J/g). These demonstrated approximately twofold increase in saturation solubility (155.01 ± 3.86 μg/ml), higher Q5min (cumulative percentage dissolution in 5 min) and lower t65% (time required for 65% dissolution) owing to greater surface area. In vivo anti-inflammatory study in Wistar rats demonstrated improvement in therapeutic efficacy of recrystallized PLB.
Rajalakshmi et al. prepared different PLB crystals using anti-solvent precipitation (PPL), melt solidification (MPL), melt quenching (MQPL), sonocrystallization (SPL) and melt sonocrystallization (MSPL) processes [Citation50]. No significant changes in solubility and dissolution rate were observed for sintered MPL and MQPL crystals, whereas slightly higher Q30min (cumulative percentage release in 30 min) and lower t50% (time required for 50% w/w drug release) were observed for irregular shaped PPL crystals due to a twofold decrease in the crystal size. As compared to needle shaped reference PLB crystals, the spongy and thread shaped crystals of SPL and MSPL showed two- and threefold increase in solubility, seven- and eightfold increase in Q30min due to five- and 10-fold reduction in crystal size with increased surface area and reduced diffusion pathway of SPL and MSPL, respectively. Moreover, an in vivo study in Wistar rats also justified improvement in the therapeutic efficacy of SPL and MSPL. They demonstrated the utility of sonoprocess to improve biopharmaceutical properties of PLB. Various novel drug delivery systems for PLB and their key findings are summarized in .
Table 3. Various novel drug delivery systems of plumbagin along with their key findings.
Conclusion
Compounds from natural sources have found considerable interest in the pharmaceutical industry in the last few decades and are being exploited for the development of novel formulations for improving their potential activities. The major drawback associated with delivery of PLB is its poor biopharmaceutical properties such as high lipophilicity, insolubility in water, short biological half-life and low melting point, although it has been found to possess oral bioavailability (38.775%). PLB also has a tendency to cause haemorrhage on chronic administration due to competitive inhibition of vitamin K activity. A wide range of pharmaceutical research using PLB as a potential candidate is being conducted with the aim of improving the bioavailability, increasing the stability and minimizing the toxicity related to the drug. The present review provides detailed information about the therapeutic activity and biopharmaceutics of PLB, issues related to drug delivery along with the different approaches utilized to improve the bioavailability of the drug. Thus, it is expected that this discussion will prompt the research community to develop an ideal drug delivery system of PLB.
Acknowledgements
The authors are grateful to Dr. D. Y. Patil College of Pharmacy, Akurdi, Pune 411044 and Poona College of Pharmacy, Bharati Vidyapeeth Deemed University, Pune 411038, Maharashtra, India, for support and institutional facilities.
Disclosure statement
The authors report no conflicts of interest. The authors alone are responsible for the content and writing of this article.
References
- Salanki A, Kutty SK. Plant derived compounds in clinical trials. Drug Discov Today. 2007;13:161–171.
- Saraf AS. Applications of novel drug delivery system for herbal formulations. Fitoterapia. 2010;81:680–689.
- Sumsakul W, Karbwang J, Na-Bangchang K. Application of SPECT/CT imaging system and radiochemical analysis for investigation of blood kinetics and tissue distribution of radiolabelled plumbagin in healthy and Plasmodium berghei-infected mice. Exp Parasitol. 2016;161:54–61.
- Kawiak A, Zawacka-Pankau J, Lojkowska E. Plumbagin induces apoptosis in Her2-overexpressing breast cancer cells through the mitochondrial-mediated pathway. J Nat Prod. 2012;75:747–751.
- Hazra B, Sarkar R, Bhattacharyya S, et al. Synthesis of plumbagin derivatives and their inhibitory activities against Ehrlich ascites carcinoma in vivo and Leishmania donovani Promastigotes in vitro. Phytother Res. 2002;16:133–137.
- Jackson JK, Higo T, Hunter WL, et al. Topoisomerase inhibitors as anti-arthritic agents. Inflamm Res. 2008;57:126–134.
- Zhang Z, Deng W, Kang R, et al. Plumbagin protects mice from lethal sepsis by modulating immunometabolism upstream of PKM2. Mol Med. 2016;22:162–172.
- Sankar R, Devamanoharan P, Raghupathi G, et al. Lipid peroxidation in plumbagin administered rats. J Biosci. 1987;12:267–271.
- Suraveratum N, Krungkrai SR, Leangaramgul P, et al. Purification and characterization of Plasmodium falciparum succinate dehydrogenase. Mol Biochem Parasitol. 2000;105:215–222.
- Aziz MH, Dreckschmidt NE, Verma AK. Plumbagin, a medicinal plant-derived naphthoquinone, is a novel inhibitor of the growth and invasion of hormone-refractory prostate cancer. Cancer Res. 2008;68:9024–9032.
- Chen CA, Chang HH, Kao CY, et al. Plumbagin, isolated from Plumbago zeylanica, induces cell death through apoptosis in human pancreatic cancer cells. Pancreatology. 2009;9:797–809.
- Sandur SK, Pandey MK, Sung B, et al. 5-Hydroxy-2-methyl-1,4-naphthoquinone, a vitamin K3 analogue, suppresses STAT3 activation pathway through induction of protein tyrosine phosphatase, SHP-1: potential role in chemosensitization. Mol Cancer Res. 2010;8:107–118.
- Xu TP, Shen H, Liu LX, et al. Plumbagin from Plumbago zeylanica L. induces apoptosis in human non-small cell lung cancer cell lines through NF-κB inactivation. Asian Pac J Cancer Prev. 2013;14:2325–2331.
- Hsu YL, Cho CY, Kuo PL, et al. Plumbagin (5-hydroxy-2-methyl-1,4-naphthoquinone) induces apoptosis and cell cycle arrest in A549 cells through p53 accumulation via c-Jun NH2-terminal kinase-mediated phosphorylation at serine 15 in vitro and in vivo. J Pharmacol Exp Ther. 2006;318:484–494.
- Shieh JM, Chiang TA, Chang WT, et al. Plumbagin inhibits TPA-induced MMP-2 and u-PA expressions by reducing binding activities of NF-kappaB and AP-1 via ERK harmacody pathway in A549 human lung cancer cells. Mol Cell Biochem. 2010;335:181–193.
- Wang CC, Chiang YM, Sung SC, et al. Plumbagin induces cell cycle arrest and apoptosis through reactive oxygen species/c-Jun N-terminal kinase pathways in human melanoma A375.S2 cells. Cancer Lett. 2008;259:82–98.
- Shih YW, Lee YC, Wu PF, et al. Plumbagin inhibits invasion and migration of liver cancer HepG2 cells by decreasing productions of matrix metalloproteinase-2 and urokinaseplasminogen activator. Hepatol Res. 2009;39:998–1009.
- Ding Y, Chen ZJ, Liu S, et al. Inhibition of Nox-4 activity by plumbagin, a plant-derived bioactive naphthoquinone. J Pharm Pharmacol. 2005;57:111–116.
- Srinivas P, Gopinath G, Banerji A, et al. Plumbagin induces reactive oxygen species, which mediate apoptosis in human cervical cancer cells. Mol Carcinog. 2004;40:201–211.
- Vijayakumar R, Senthilvelan M, Ravindran R, et al. Plumbago zeylanica action on blood coagulation profile with and without blood volume reduction. Vascul Pharmacol. 2006;45:86–90.
- Jeong SH, Choi JS, Ko YK, et al. The discovery of bioisoster compound for plumbagin using the knowledge-based rational method. J Mol Struct. 2015;1085:84–89.
- Hsieh YJ, Lin LC, Tsai TH. Measurement and pharmacokinetic study of plumbagin in a conscious freely moving rat using liquid chromatography/tandem mass spectrometry. J Chromatogr B Analyt Technol Biomed Life Sci. 2006;844:1–5.
- Chen A, Zhou X, Tang S, et al. Evaluation of the inhibition potential of plumbagin against cytochrome P450 using LC–MS/MS and cocktail approach. Sci Rep. 2016;6:28482.
- Bothiraja C, Pawar AP, Mali AJ, et al. Improved pharmaceutical properties of surface modified bioactive plumbagin crystals. Int J Surf Sci Eng. 2013;7:181–195.
- Bardania H, Tarvirdipour S, Dorkoosh F. Liposome-targeted delivery for highly potent drugs. Artif Cells Nanomed Biotechnol. 2017;45:1478–1489.
- Panahi Y, Farshbaf M, Mohammadhosseini M, et al. Recent advances on liposomal nanoparticles: synthesis, characterization and biomedical applications. Artif Cells Nanomed Biotechnol. 2017;45:788–799.
- Tiwari SB, Pai RM, Udupa N. Temperature sensitive liposomes of plumbagin: characterization and in vivo evaluation in mice bearing melanoma B16F1. J Drug Target. 2002;10:585–591.
- Sunil Kumar MR, Aithal BK, Udupa N, et al. Formulation of plumbagin loaded long circulating pegylated liposomes: in vivo evaluation in C57BL/6J mice bearing B16F1 melanoma. Drug Deliv. 2011;18:511–522.
- Drummond DC, Meyer O, Hong K, et al. Optimizing liposomes for delivery of chemotherapeutic agents to solid tumors. Pharmacol Rev. 1999;51:691–743.
- Behnam B, Rezazadehkermani M, Ahmadzadeh A, et al. Microniosomes for concurrent doxorubicin and iron oxide nanoparticles loading; preparation, characterization and cytotoxicity studies. Artif Cells Nanomeds Biotechnol. 2017 [Apr 4]; [11 p.]. DOI:https://doi.org/10.1080/21691401.2017.1296850
- D’Souza Singh UV, Aithal KS, et al. Antifertility activity of niosomal HPβCD-Plumbagin complex. Indian J Pharm Sci. 1998;60:36–40.
- Oommen E, Dinesh Shenoy B, Udupa N, et al. Antitumour efficacy of cyclodextrin-complexed and harmac encapsulated plumbagin in mice bearing melanoma B16F1. Pharm Pharmacol Commun. 1999;5:281–285.
- Naresh RA, Udupa N, Devi PU. Niosomal plumbagin with reduced toxicity and improved anticancer activity in BALB/C mice. J Pharm Pharmacol. 1996;48:1128–1132.
- Kini DP, Pandey S, Shenoy BD, et al. Antitumor and antifertility activities of plumbagin controlled release formulations. Indian J Exp Biol. 1997;35:374–379.
- Sah AK, Vyas A, Suresh PK, et al. Application of nanocarrier-based drug delivery system in treatment of oral cancer. Artif Cells Nanomeds Biotechnol. 2017 [Sep 7]; [8 p.]. DOI:https://doi.org/10.1080/21691401.2017.1373284
- Afsharzadeh M, Hashemi M, Mokhtarzadeh A, et al. Recent advance in co-delivery systems based on polymeric nanoparticle for cancer treatment. Artif Cells Nanomeds Biotechnol 2017 [Sep 28]; [16 p.]. DOI:https://doi.org/10.1080/21691401.2017.1376675
- Indhumathi D, Remya PN, Sangeetha S. Formulation trails on nanoparticular preparation for easy scale up through different techniques. J Chem Pharm Sci. 2013;6:170–174.
- Bothiraja C, Kapare HS, Pawar AP, et al. Development of plumbagin-loaded phospholipid-tween mixed micelles: formulation, optimization, effect on breast cancer cells and human blood/serum compatibility testing. Ther Deliv. 2013;4:1247–1259.
- Pawar A, Patel R, Arulmozhi S, et al. D-a-Tocopheryl polyethylene glycol 1000 succinate conjugated folic acid nanomicelles: towards enhanced bioavailability, stability, safety, prolonged drug release and synergized anticancer effect of plumbagin. RSC Adv. 2016;6:78106–78121.
- Wang W, Lei Y, Sui H, et al. Fabrication and evaluation of nanoparticle-assembled BSA microparticles for enhanced liver delivery of glycyrrhetinic acid. Artif Cells Nanomeds Biotechnol. 2017;45:740–747.
- Singh UV, Bisht KS, Rao S, et al. Plumbagin-loaded PLGA microspheres with and enhanced antitumour efficacy reduced in mice toxicity. Pharm Pharmacol Commun. 1996;2:407–409.
- Mandala RSK, Aithal K, Anandam A, et al. Preparation, in vitro characterization, pharmacokinetic, and harmacodynamics evaluation of chitosan-based plumbagin microspheres in mice bearing B16F1 melanoma. Drug Deliv. 2010;17:103–113.
- Gorjikhah F, Jalalian FA, Salehi R, et al. Preparation and characterization of PLGA-β-CD polymeric nanoparticles containing methotrexate and evaluation of their effects on T47D cell line. Artif Cells Nanomeds Biotechnol. 2017;45:432–440.
- Srinivas P, Patra CR, Bhattacharya S, et al. Cytotoxicity of naphthoquinones and their capacity to generate reactive oxygen species is quenched when conjugated with gold nanoparticles. Int J Nanomedicine. 2011;6:2113–2122.
- Appadurai P, Rathinasamy K. Plumbagin-silver nanoparticle formulations enhance the cellular uptake of plumbagin and its antiproliferative activities. IET Nanobiotechnol. 2015;9:264–272.
- Hafeez BB, Kashyap VK, Boya VN, et al. Novel nanoparticle formulation of Plumbagin for pancreatic cancer treatment. In: Proceedings of the 107th Annual Meeting of the American Association for Cancer Research; 2016 Apr 16–20; New Orleans, LA. Philadelphia (PA): AACR; Cancer Res 2016;76(14 Suppl).
- Shahida Parveen SD, Affrose A, Suresh Kumar B, et al. Synthesis, characterization, and DNA binding studies of nanoplumbagin. J Nanomater. 2014;2014:9.
- Duraipandy N, Lakra R, Kunnavakkam VS, et al. Caging of plumbagin on silver nanoparticles imparts selectivity and sensitivity to plumbagin for targeted cancer cell apoptosis. Metallomics. 2014;6:2025–2033.
- Bothiraja C, Pawar AP, Mali AJ, et al. Improved pharmaceutical properties of surface modified bioactive plumbagin crystals. Int J Sur Sci Eng. 2013;7:181–195.
- Rajalakshmi S, Pawar AP, Mali AJ, et al. Crystal engineering of bioactive plumbagin using anti-solvent precipitation, melt solidification and sonocrystallization techniques. Mater Res Express. 2014;1:1–19.