Abstract
A new modality of drug targeting to tumors has been proposed. The ligand-mediated approach, that already increases the therapeutic index of the drug, can still be optimized by the encapsulation of the drug into sonosensitive nanoparticles. In this work, an endogenous ligand, estrone, was used to synthesize doxorubicin-encapsulating liposomes for estrogen receptor (ER)-positive breast cancer therapy with cyanuric chloride (2,4,6 trichloro-1,3,5 triazine) being used as a linking molecule to attach 3–OH group of estrone to the surface of liposomes. Then, drug release from liposomes was studied using ultrasound waves as a triggering mechanism with different frequencies and power densities. In addition, drug uptake by two cell lines ER-positive (MCF-7) and ER-negative (MDA-MB-231) was assessed, with the former cell line being examined later to study the synergetic effect of the receptor mediator targeting and ultrasound trigger. The sizes of the liposomes loaded with calcein (as a doxorubicin model drug) were determined by dynamic light scattering, and they were characterized as large unilamellar vesicles (LUVs). The release from the prepared liposomes triggered by ultrasound (US) waves at low frequency (20 kHz) and high frequency (1.07 and 3.24 MHz), at several power densities, was determined by monitoring the changes in calcein fluorescence, using a spectrofluorometer. Increasing power densities showed a significant effect on release at high frequencies and during the first two US pulses at low frequency. The echogenicity of the liposomes was proven and characterized at different power densities and frequencies. To confirm the viability of the carrier as a doxorubicin carrier, doxorubicin-encapsulating liposomes were prepared using the ammonium sulfate transmembrane gradient method. The liposomes were LUVs and were US-sensitive, exhibiting similar behavior to calcein-encapsulating liposomes. The calcein uptake by an ER + cell line (MCF-7) was compared with the uptake by an ER-cell line (MDA-MB-231). The MCF-7 uptake was significantly higher than the MDA-MB-231 uptake, which proved the targeting potential of estrone-conjugated liposomes. The exposure to low-frequency ultrasound (LFUS) revealed a statistically significant uptake of calcein compared to uptake without ultrasound. The described drug delivery (DD) system, comprising a new echogenic liposomal formulation, promises a non-immunogenic and site-specific biomedical approach to ER-positive breast cancer therapy.
Graphical Abstract
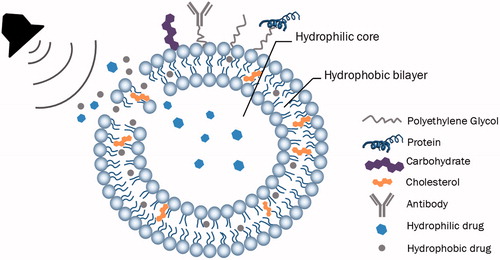
Introduction
Chemotherapy is frequently used in cancer treatment; however, side effects associated with this type of treatment are often detrimental to the patient’s health as the cytotoxic agents do not distinguish between normal and malignant cells. One approach to minimize the side effects associated with conventional systemic chemotherapy and the exposure of normal cells to the drug is the encapsulation of the chemotherapeutic drug in nanocarriers before being administered in vivo. This approach, however, suffered from several drawbacks, which limited the efficiency of the drug.
One of these limitations is the low bioavailability due to opsonization. Foreign bodies, such as the nanocarriers, are marked for removal by the reticulo-endothelial system (RES), resulting in their poor accumulation inside tumors [Citation1]. To solve this issue, researchers found that the circulation time of nanocarriers in vivo can be enhanced by coating them with polymers such as polyethylene glycol (PEG), which are capable of delaying their clearance from circulation and often results in liposomes of smaller size due to reduction in vesicles aggregation [Citation1,Citation2]. A study conducted by Xu and Meng reported a higher circulation time for paclitaxel-loaded stealth liposomes when compared with conventional liposomes (half-life time of 34.2 h versus 13.7 h) [Citation3]. This improvement of the nanocarrier circulation by PEG coating, along with the tumor features of low lymphatic drainage and porous angiogenic endothelium, is responsible for a phenomenon called the enhanced permeability and retention effect (EPR).
Another limitation of drug encapsulation is the decrease in the drug concentration at the tumor site and its internalization into the cancer cells. Thus, to overcome these limitations one of two approaches was used:
Active targeting drug delivery. The basic mechanism of active targeting is either to improve the co-localization of the drug and cancer cells [Citation4], or to improve the cellular internalization via receptor-mediated endocytosis by conjugating ligands to the surface of the nanocarriers [Citation1]. Ligands are defined as molecules that can effectively guide therapies to specific cell biomarkers to achieve certain functionality [Citation5].
Trigger-stimulated drug release. The release of the drug from the nanocarriers at the tumor site was enhanced by either applying external stimulus, such as ultrasonic or magnetic waves, or internal mechanism within the structure of the nanocarriers that responds to tumor-specific environment, such as pH, temperature, enzymes or light stimuli [Citation6–11].
Structure of nanocarriers
A liposome is a hollow structure made up of a phospholipid bilayer and it was first discovered in the 1960 s by the British scientist, Alec Bangham when he was studying the effect of phospholipids on clotting blood [Citation12]. Liposomes are valuable nanocarriers in DD since they are biocompatible, biodegradable and, due to their amphiphilic nature, they can be loaded with hydrophobic drugs in the lipid bilayer and hydrophilic drugs in the inner core [Citation13]. In addition, liposomes can be used efficiently in active targeting by conjugating targeting moieties to the surface of liposomes.
Ligands used in active DD are classified either as exogenous, which initiates immunological reactions, or endogenous ligands, which are non-immunogenic and biocompatible [Citation14]. Endogenous ligands include antibodies, polypeptides, hormones and fusogenic proteins. Some of the receptors binding to endogenous ligands, such as transferrin receptors, lipoprotein receptors and hormone receptors, are overexpressed in cancer cells as compared to normal cells. Of these hormones, estrogens are important steroid hormones that work as chemical messengers in the body and include estrone, estradiol and estriol. The function of estrogens is mainly mediated by two receptor proteins: ERα and ERβ [Citation14–16]. These ERs are present in two-thirds of breast cancers and they are distributed on the nucleus and the cell membrane [Citation17]. The presence of ERs on the cell membrane can be utilized in guiding therapies towards breast cancer cells [Citation17]. In postmenopausal women, the major estrogen is estrone, which is primarily produced from the aromatization of androstenedione, which is mainly (∼95%) produced in the adrenal glands [Citation18]. Estrone (C18H22O2, molecular weight =270.4 g/mol) has the second highest affinity towards ER after estradiol. It transfers much of its signal (60%) to ERα while 37% of its signal is transferred to ERβ [Citation19]. Since breast cancer overexpresses ERα, estrone is considered as a potential ligand in cancer therapeutic drugs. Paliwal et al. [Citation17] developed doxorubicin-encapsulating liposomes anchored with estrone moieties and found a six-fold increase in the accumulation of doxorubicin in breast cancer cells.
Enhancement of cellular uptake of drugs by actively-targeted nanoparticles is widely supported by the literature. Song et al. [Citation20] showed that cellular uptake of liposomal apatinib was enhanced by the conjugation of cyclic-RGD to the liposomal DD system. An 11.2-fold increase in cellular uptake was achieved by the modification of a liposomal doxorubicin system with an SP5–2 moiety by Chang et al. [Citation21] Transferrin-modified nanoparticles demonstrated a six-fold increase in uptake versus control liposomes for a breast cancer cell line [Citation22]. Similarly, several other actively targeted therapies have been developed to deal, specifically, with breast cancer using various targeting ligands including transferrin [Citation23,Citation24], estrogen [Citation25], tamoxifen [Citation26], folic acid [Citation27], RGD peptide [Citation28,Citation29] and many others [Citation30–33], yielding similar success to the previously outlined articles.
Triggering stimulus
Triggering in drug delivery is defined as the method that allows the control of drug release in its amount, its location and the period in which it is being released [Citation6]. Several triggering techniques, such as pH, temperature, enzymes and light stimuli, have been suggested to release liposome-encapsulated drugs [Citation7–10]. In addition to these techniques, research has shown that drug release can be controlled efficiently via US waves [Citation11,Citation34–47].
In liposomal drug delivery, US is utilized to control the release of a certain drug via mechanical and/or thermal effects. This technique of drug release is applied to echogenic and emulsion liposomes (eLiposomes). The two main parameters of US involved in triggering the release of drugs are the frequency and the intensity. In DD, high-intensity focused US (HIFU) is used mainly in the release of drugs from temperature-sensitive liposomes, but HIFU can also treat (kill) tissues by inducing elevated temperatures through a process known as hyperthermia [Citation47]. Release of drugs can also be achieved using the non-thermal effects of LFUS.
Several mechanisms involved in the US-induced uptake of nanoparticles in general, and liposomes in particular, have been discussed in several papers [Citation35,Citation37]. The three main mechanisms that lead to a change in the cell accumulation of the molecule loaded in the nanoparticle are, (1) the enhancement of the release before the nanoparticles reaches the cell, (2) the enhancement of cellular uptake mainly by endocytosis and (3) the sonoporation of the cell membrane. The exact phenomena induced by the mechanical effect of US on the liposomes or the cells and their in vivo effects are unclear, however. Cavitation (mostly transient cavitation) has been proposed as the main driver of release and cellular uptake [Citation39,Citation41,Citation43], but evidence points to radiation force-induced acoustic streaming as well [Citation48]. Also, the increase in the enthalpy of drug internalization after US exposure has been hypothesized and seems to contribute to the increment in the uptake of small drugs loaded in nanoparticles [Citation37].
Several parameters affect the release of drugs from nanoparticles. Husseini et al. [Citation42] demonstrated that, in vitro, LFUS has the ability to release contents of nanoparticles and that the effect is enhanced by increasing power density. Pulsed US exposure reduces the temperature increase in tissue caused by energy dissipation (versus continuous US wave exposure) limiting the thermal effects of US and limiting healthy tissue damage [Citation49]. Kost et al. [Citation45] demonstrated that the exposure to LFUS can increase the permeability of biological barriers. This phenomenon has been used to increase the permeability of many biological barriers, such as cell membranes and tumors, thus facilitating the extravasation of the drug molecules into the desired site [Citation44]. Schlicher et al. [Citation50] showed that cell membranes can be disrupted via LFUS-induced cavitation. The LFUS results in transient pore-like disruptions in the membrane with diameters lower than 28 nm, and for a duration of several minutes, after which the cellular repair mechanism recovers the intact membrane configuration.
In this work, estrone-conjugated liposomes were prepared to target an estrone-positive breast cancer cell line (MCF-7), which overexpresses the corresponding receptors. Modified liposomes were prepared by attaching the active and endogenous ligand estrone (ES) to the PEGylated phospholipid 1,2-distearoyl-sn-glycero-3-phosphoethanolamine-N- [amino(polyethylene glycol)-2000] (DSPE-PEG2000-NH2) and were loaded with either the model drug, calcein, or the chemotherapeutic drug, doxorubicin. The release from those liposomes triggered by ultrasound waves at low and high frequencies was then investigated. Then, the effect of actively-targeted liposomes and LFUS on MCF-7 (ER-positive) cells and MDA-MB-231 (ER-negative) cells was investigated to determine the improvement in cell uptake of the fluorophore, calcein, and to determine whether there is a synergistic effect between ultrasound and targeted liposomal drug delivery.
Experimental section
Materials
The DPPC and DSPE-PEG2000-NH2 (ammonium salt) were obtained from Avanti Polar Lipids Inc. (Alabaster, AL, USA). Sephadex G-100, Sephadex G-25, estrone (ES), calcein disodium salt, potassium bromide (KBr), and 2,4,6 trichloro-1,3,5 triazine (cyanuric chloride (CC)) were obtained from Sigma-Aldrich (St. Louis, MO, USA). Triethylamine (TEA) was obtained from Reidel-de Haёn (Seelze, Lower Saxony, Germany). Chloroform was obtained from Panreac Quimica S.A. (Castellar del Vall`s, Barcelona, Spain). Cholesterol was obtained from AlfaAesar (Ward Hill, MA, USA). Doxorubicin-Hydrochloride was obtained from Euroasian Chemicals (Lower Parel, Mumbai, India).
Cell cultures
The MCF-7 (ER-positive human breast adenocarcinoma) and MDA-MB-231 (ER-negative human breast adenocarcinoma) cell lines were a gift from NanoBB group, iMed, University of Lisbon, Portugal. Both cell lines were cultured in RPMI medium supplemented with 10% heat inactivated fetal bovine serum (FBS) and 1% penicillin-streptomycin (Sigma-Aldrich, St Louis, MO, USA). The cell cultures were maintained at 37 °C in a humidified atmosphere with 5% CO2. 24 h before the uptake studies, exponentially growing cells were harvested with 3 ml of trypsin (0.25% from Sigma-Aldrich) and 3 × 105 cell/mL of growth medium were seeded in 6-well plates to reach confluency at the time of the experiment.
Synthesis of the DSPE-PEG2000-CC-ES modified lipids
Cyanuric chloride (2,4,6 trichloro-1,3,5 triazine (CC)) was used as a linking molecule to attach the small targeting moiety ES to DSPE-PEG2000-NH2. ES was reacted with CC in a 1:1 molar ratio, in the presence of two molar equivalents of triethylamine (TEA), a chloride acceptor [Citation51]. To achieve the mono-substitution of chlorine, the reaction was performed at 0 °C for more than three hours [Citation51]. The solution of estrone with TEA dissolved in dry chloroform was added dropwise to a solution of CC dissolved in dry chloroform at 0 °C. The functionalized ligand 2,4 dichloro, 6 estrone-1,3,5 triazine (CC-ES) was then reacted with the lipids DSPE-PEG2000-NH2 in a 1:1 molar ratio, in the presence of two equivalents of TEA using chloroform as a solvent, and the reaction was carried at 0 °C for three hours, then the mixture was left stirring overnight at room temperature. If the prepared lipids were not used immediately, then chloroform was evaporated by purging with argon and the remaining solid is stored at −20 °C.
Preparation of control and estrone-targeted liposomes encapsulating calcein
Various procedures are available to prepare liposomes. Non-targeted or control liposomes (with DSPE-PEG2000-NH2 lipid) and ES-targeted liposomes (with DSPE-PEG2000-CC-ES modified lipid) were prepared according to the modified lipid film hydration method described by Lasch et al. [Citation52] and Cullis et al. [Citation53].
Liposomes were synthesized at 60 °C (i.e. above the transition temperature Tm of lipids) using DPPC, cholesterol and DSPE-PEG2000-NH2 (or DSPE modified lipid as described above) at a molar ratio of 65:30:5, which is close to the optimum lipid to cholesterol ratio reported by Bhardwaj et al [Citation54]. For one batch of liposomes, 2 µmol of the synthesized lipids (DSPE-PEG2000-NH2 or DSPE-PEG2000-CC-ES) were dissolved in 3 ml of dry chloroform in a round bottom flask, followed by the addition of 4.7 mg (12 µmol) of cholesterol and 19.2 mg (26 µmol) of DPPC. Chloroform was then evaporated to dryness in a rotary evaporator at 60 °C for 15 min until a greasy-like film formed on the flask walls. This lipidic film was hydrated with 30 mM (In vitro Sonosensitivity Experiments) or 50 mM calcein (Cellular Uptake of Liposomes Studies) in phosphate buffered saline (PBS) at a pH of 7.4 for 45 min at 60 °C using the rotary evaporator without vacuum. Sonication was then performed in a 40 kHz sonicator bath (Elma D-78224, Melrose Park, IL, USA) at 60 °C, for 15 min, after which the sample was extruded 30 times at the same temperature, using the Avanti® Mini-extruder with 0.2 µm polycarbonate filters (Avanti Polar Lipids, Inc., Alabaster, AL, USA). Liposomes were purified using a Sephadex G-100 gel filtration column (GE Healthcare Life Sciences, Pittsburgh, PA, USA)), which was previously equilibrated with PBS, pH 7.4. The collected turbid liposome fractions were labeled and stored at 4 °C until use.
The same protocol described above was used to prepare liposomes encapsulating ammonium sulfate instead of calcein. In addition, the ammonium sulfate gradient [Citation55] method was followed with minor variations to encapsulate doxorubicin. For more details, refer to the Supporting Information.
Determination of liposome size by dynamic light scattering (DLS)
The mean size of the liposomes was determined by Dynamic Light Scattering (DLS) using the DynaPro® NanoStar™ (Wyatt Technology Corp., Santa Barbara, CA, USA). The hydrodynamic radius (Rh) of the liposomes was determined at room temperature after diluting 10 µL of the liposomal sample in 1 ml of PBS.
In vitro sonosensitivity
Both low- and high-frequency ultrasound (LFUS and HFUS) release studies were conducted. In LFUS studies, the release of calcein from liposomes was triggered using 20 kHz at three power densities (6.08, 6.97, 11.83 W/cm2). The power densities of US radiation were estimated by hydrophone measurement as described by Ahmed et al. [Citation11]. At HFUS, release studies were conducted at 1.07 MHz (10.5 and 50.2 W/cm2) and 3.24 MHz (∼173 W/cm2). For further details, see the Supporting Information.
Cellular uptake of liposomes
Non-targeted calcein liposomes or ES-conjugated calcein liposomes hydrated with 30 mM of calcein were added to MCF-7 or MDA-MB-231 confluent cells at a concentration of 200 µM of DPPC. The concentration of DPPC was estimated by the spectrophotometrical Stewart assay [Citation56]. The liposomes were incubated with the cells in 6-well plates for 30 min in humidified air at 37 °C and 5% CO2. Subsequently, the cell plates were washed with PBS and harvested with a trypsin solution for subsequent flow cytometry measurements (Beckman coulter FC500, Brea, CA, USA). As aforementioned, samples were analyzed to measure calcein fluorescence intensity using an excitation and emission wavelengths of 480 and 520 nm, respectively. Two or more independent assays were performed.
To study the cellular uptake of liposomes in an ultrasound exposure environment, ES-conjugated calcein liposomes hydrated with 50 mM of calcein were incubated with MCF-7 cells at the same conditions. After the initial 30 min of incubation, the plates were sonicated floating in a 40 kHz water bath for 60 s (pulsed sonication at 10 s on and 20 s off cycles). No temperature increase was observed in cell-containing wells during sonication. After US exposure, liposomes and cells were incubated for 2.5 h in humidified air at 37 °C and 5% CO2. Later, the cells were washed and harvested with a trypsin solution for calcein uptake quantification.
Control samples were kept without sonication in the same liposome-containing medium at the same temperature and for the same time. In each insonation experiment, the sonolysis studies (the effect of US on cell viability) were performed using the Trypan blue exclusion assay and the cell viabilities were always higher than 90%.
Statistical analysis
The differences between the results were compared using a two-tailed t-test with the assumption of unequal variances. Two values were considered significantly different when p < .05.
Results and discussion
Synthesis of the DSPE-PEG2000-CC-ES modified lipids
In order to use a rapid, simple and safe way to couple DSPE–PEG to different targeting ligands, we chose cyanuric chloride (CC), which is a bifunctional coupling reagent. The first two chloride substitutions of CC can be achieved upon reacting with nucleophiles under slightly basic conditions, the first reaction at 0 °C and the second at room temperature. At the same conditions, the third chloride substitution reaction is strongly depressed and is predicted to occur above 60 °C [Citation51].
The second step of the reaction is the substitution of the second chloride in the cyanuric ring. The nucleophilic conjugation of the amine of DSPE-PEG-NH2 to the ES-CC conjugate at room temperature in basic condition is described in . For more details, see Figures S1 and S2 in the Supporting Information.
Liposome particle size by DLS
The radius of ES-conjugated, calcein liposomes was found to be 92.9 ± 15.8 nm, while a radius of 96.8 ± 3.8 nm was obtained for non-targeted (control) calcein liposomes. Further, a radius of 97.1 ± 14.4 nm was found for the ES-conjugated, doxorubicin liposomes. Thus, based on size, all three types of liposomes are categorized as LUVs [Citation57].
LFUS-induced release
Calcein liposomes were insonated using a 20 kHz probe, and drug release was investigated at three power densities: 6.08, 6.97, and 11.83 W/cm2 [Citation11]. The normalized-averaged release profiles, highlighted with standard deviation, of control liposomes are shown in . As can be seen, the initial rate of release increased with increasing power amplitudes during the first 200 s. After 13 min of pulsed insonation, TX-100 was added to lyse liposomes and the fluorescence was measured for an additional 2 min to reach maximum release. However, by adding TX-100, it was noticed that the fluorescence level was only slightly above the preceding plateau, which indicates that liposomes have released most of their encapsulated calcein contents.
Figure 2. Normalized release profiles for DSPE-PEG2000-NH2 liposomes triggered by 20-kHz LFUS at three power densities. Results are average ± standard deviation of 2 liposome batches (3 replicates each).
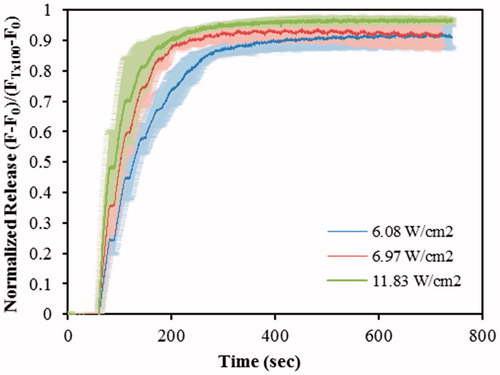
The initial and final rates of release were calculated, see Table S1 (in Supporting Information) using the release profiles shown in . The initial release rates were approximated by the normalized fluorescence values obtained after the first (60 to 90 s) and second pulses (90 to 120 s) of US. The final release percent was calculated after 13 min of pulsed insonation by adding TX-100 to lyse the remaining liposomes in solution. The release percent, calculated after the first US pulse, increased significantly (p < .05) as the power density increased; the release percent at 6.08 W/cm2 is significantly lower (p = 5.87 × 10–3) than the release observed at 6.97 W/cm2 and also significantly lower (p = 1.42 × 10–6) than at 11.83 W/cm2. The release at 6.97 W/cm2 is also significantly lower (p = .042) than the one at 11.83 W/cm2. Similar results were obtained when comparing the release observed after the second US pulse: the release significantly increases (p < .05) with increasing power density. These results show that the release is more pronounced at the higher power densities investigated.
Figure S3, shown in the Supporting Information, provides evidence of liposomal membrane sonoporation upon exposing our targeted nanocarriers to ultrasound as evidenced by the increase in fluorescence level during the “on” cycles. This phenomenon is expected and well documented and is mainly due to collapse/transient cavitation at LFUS as discussed by Schroeder et al. [Citation58], Richardson et al. [Citation59], and Pitt et al. [Citation60]. Schroeder et al. [Citation47] reported that the power density threshold for cavitation induced by LFUS is approximately 1.2 W/cm2 at 20 kHz. Evjen [Citation38] further investigated whether liposomes disintegrate upon exposure to US by conducting an HPLC study on doxorubicin-encapsulating DOPE-based and DSPE-based liposomes before and after exposure to 40 kHz and 1 MHz US. The analysis concluded that molecules of doxorubicin, cholesterol and phospholipids did not disintegrate after being exposed to US but kept their original chemical structure. Based on that, we concluded that sonoporation is the main mechanism behind this acoustically activated drug release phenomenon and that LFUS did not affect the chemical integrity of the drug or the lipids.
ES-targeted calcein liposomes were sonicated at the same frequency and power densities as the control liposomes. The release profiles are shown in , and the initial rates are presented in Table S2. No statistically significant difference (p > .05) were observed between the initial release rates (first and second US pulses) and the final release percent between 6.08 W/cm2 and 6.97 W/cm2. But releases after the first and second pulses were found significantly higher for the highest power density when compared to the 6.08 W/cm2 (p = 8.85 × 10–6 and p = 3.13 × 10–7, respectively) and to the ones obtained at 6.97 W/cm2 (p = 4.39 × 10–3 and p = 1.10x10–3, respectively). We believe that there is ample evidence that cavitation has a major role in the ultrasound-assisted release of the model drug from these targeted liposomes.
Figure 3. Normalized release profiles for DSPE-PEG2000-CC-ES liposomes triggered by 20-kHz LFUS at three power densities.
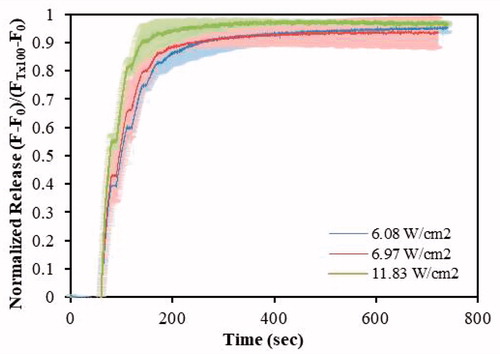
Researchers have conducted extensive studies on micellar [Citation37,Citation40,Citation42,Citation43,Citation60–66] and liposomal [Citation6,Citation11,Citation36,Citation38,Citation39,Citation47,Citation65] US-triggered release and enhanced cellular uptake. Studies using ES-conjugated liposomes and US, however, are scarce. Paliwal et al. [Citation17] investigated doxorubicin release of a PC-based liposomal formulation with DPPE-PEG-ES using the dialysis tube method. Assuming that sonosensitivity is independent of the encapsulated chemical (i.e. doxorubicin or calcein), as reported by Evjen [Citation38], the maximum doxorubicin release achieved by Paliwal et al. for ES-conjugated liposomes using the dialysis tube method was 47.3 ± 1.34% after 24 h [Citation17]. In this work, we achieved high-release levels (54.96 ± 3.43%) with one US pulse (20 s insonation), at a power density of 11.83 W/cm2.
A comparison between control and ES-conjugated liposomes in terms of US-actuated calcein release is shown in . A two-tailed t-test with unequal variance showed no statistically significant difference (p > .05) between the final (after 13 min of the experiment) targeted and non-targeted liposomal release at all power densities investigated. In contrast, targeted liposomes showed a significantly higher release (compared to non-targeted liposomes) at 6.08 W/cm2 and 11.83 W/cm2, after the first (p = 1.36 × 10–6 and p = 6.63 × 10–5, respectively) and the second US pulses (p = 6.64 × 10–5 and p = 2.54 × 10–6, respectively).
Figure 4. Comparison of calcein release from DSPE-PEG2000-CC-ES and DSPE-PEG2000-NH2 liposomes triggered by 20-kHz LFUS at the indicated power densities. (A) Release after the first US pulse, (B) Release after the second US pulse, (C) Final release.
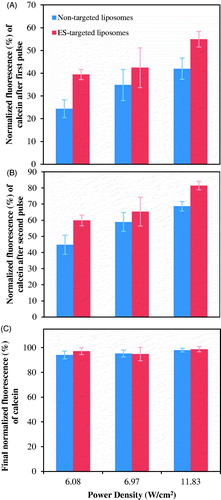
Estrone, which is very similar to cholesterol [Citation67], is insoluble in aqueous solutions [Citation68]. Hence, there is a high probability that estrone molecules, anchored to the surface of liposomes, escape the surrounding medium (i.e. the aqueous PBS buffer) and incorporate their structures into the bilayer membrane. The inclusion/intercalation of estrone molecules into the structure of a phospholipid membrane is believed to affect its nature and integrity as reported by Troxell et al. [Citation67]. This may cause disorder and defects in the membrane, which ultimately may facilitate release. ES-targeted doxorubicin liposomes were sonicated at the same frequency and power densities as was conducted for calcein. The sonosensitivity of these liposomes was confirmed and the release profile was similar to the ones shown in and . This reaffirms Evjen’s [Citation38] conclusion that sonosensitivity does not depend on the encapsulated drug.
HFUS-induced release
HFUS is a term used for US waves with frequencies higher than 1 MHz [Citation47]. In this work, two high frequencies were investigated, 1.07 and 3.24 MHz, at two power densities (10.5 and 50.2 W/cm2) for the former, and one power density (∼173 W/cm2) for the latter frequency. Non-targeted calcein liposomes were sonicated at HFUS frequencies and power densities mentioned above; the release profiles are shown in .
Figure 5. Normalized calcein release profiles from (A) DSPE-PEG2000-NH2 liposomes, (B) DSPE-PEG2000-CC-ES liposomes, triggered by 1.07 and 3.24 MHz HFUS, at the power densities indicated in the legend. Results are average ± standard deviation (3 replicates).
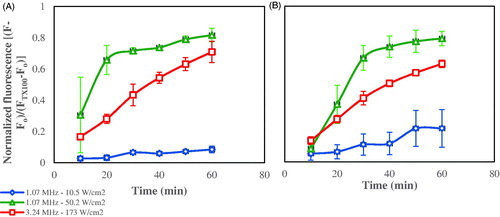
Using 1.07 MHz HFUS, the release significantly increased (p < .05) with power density at each time point, except at 10 min (). The release at 10.5 W/cm2 was much lower than at 50.2 W/cm2, and it showed little increase with time, ultimately reaching a final release of 8.45% after 60 min of insonation. On the other hand, the release at the higher power density (50.2 W/cm2) showed rapid increase during the first 20 min of insonation, after which the increase continued at a slower rate. At this power density, the final release achieved after 60 min of insonation was 81.67%, approximately 10 times more than that achieved at 10.5 W/cm2.
The release profile at 3.24 MHz showed a more sustained pattern of linear increase with time, but the final release after one hour of insonation (70.9%) is still less than that achieved at the higher power density investigated at 1.07 MHz.
The liposomes synthesized in this work are DPPC-based (i.e. the major lipid constituent), with small amounts of DSPE-PEG and cholesterol. The transition temperature of DPPC is 41.3 °C [Citation69], and the transition temperature of DSPE is even higher at 74 °C [Citation70], but the inclusion of cholesterol counteracts the effect of DSPE by lowering the transition temperature of saturated lipids. Therefore, it is believed that hyperthermia may be one reason for permeating the membrane and for releasing calcein, especially at 3.24 MHz because the attenuation of US waves (and conversion to heat) increases with increasing frequency [Citation60]. Another mechanism that may contribute to calcein release is acoustic streaming, which has a mechanical effect rising from the partial absorption of US energy by the fluid. This energy is translated then to a convective flow in the direction of US propagation, thus inducing mixing and nanoparticle collisions that may ultimately rupture the membrane and release the encapsulated agent.
Liposomes targeted with an ES-conjugate were tested for release at the same two frequencies (1.07 and 3.24 MHz) and power densities (as employed in the non-targeted liposomes study mentioned above). As observed earlier, calcein release increases as the power density increased at each time interval. And according to , at the power density of 50.2 W/cm2, the calcein release increased at a higher rate during the first 30 min of insonation compared to the last 30 min. Similar behavior was also noticed with control liposomes. The release at 10.5 W/cm2 showed a slight increase with time and reached a final 60 min release percent of 21.51% compared to 79.31% at 50.2 W/cm2.
The release at 3.24 MHz (∼173 W/cm2) exhibited a linear increase with time and a final release of 63.02% was measured after 1 h of insonation, which is lower than that achieved at 1.07 MHz and 50.2 W/cm2. The mechanism of release is expected to be due to hyperthermia and/or stable cavitation at 10.5 W/cm2 and collapse/transient cavitation at 50.2 and 173 W/cm2. In addition, acoustic streaming and hyperthermia may also have contributed to release at 3.24 MHz. The release increased significantly as the power density increased for the first 10 min of insonation.
A statistical comparison between ES-conjugated liposomes and control liposomes at each frequency and power density was also performed. No statistically significant differences were calculated between the two types of liposomes in all the HFUS parameters studied in this work. Hence, the effect of the targeting moiety (ES) seems to be negligible, i.e. the targeting moiety does not interfere with release percent/profile/dynamics when HFUS is used.
Cellular uptake of ES-conjugated calcein liposomes vs non-targeted calcein liposomes
In order to evaluate the effect of the presence of ERs on the cellular uptake of the ES-conjugated liposomes, flow cytometry was used to measure the cellular uptake of calcein by an ER-positive (MCF-7) and ER-negative (MDA-MB-231) human breast adenocarcinoma cell lines. To optimize our experiments, several concentrations of liposomes and fluorophore were examined. The optimal results were obtained with 200 µM of liposomes (concentration estimated by the amount of the major lipid incorporated by the nanoparticle, DPPC, using the Stewart assay) and 30–50 mM of calcein.
Liposomes were incubated for 30 min with both cell lines and flow cytometry analysis was performed to evaluate the efficacy of ES-conjugated liposomes in actively targeting the ER-positive breast cancer cell line.
The cellular uptake of calcein from ES-conjugated liposomes was statistically higher in MCF-7 than the uptake in MDA-MB-231 (p = 6.98 × 10–4). The average geometric mean of the peak fluorescence for ES-conjugated liposomes in MCF-7 was 13,300 ± 6208 while, it was 3447 ± 629 in MDA-MB-231. shows representative flow cytometry histograms that compare uptake in MCF-7 and in MDA-MB-231. The results indicate the prevalence of ligand-mediated endocytosis regulated by the binding of ES on the ERs. The ES moiety gives the carrier the ability to recognize and confer site specificity.
Figure 6. Calcein uptake of ER-negative MDA-MB-231 (on the left) and ER-positive MCF-7 (on the right) cells incubated for 30 min with ES-conjugated calcein liposomes.
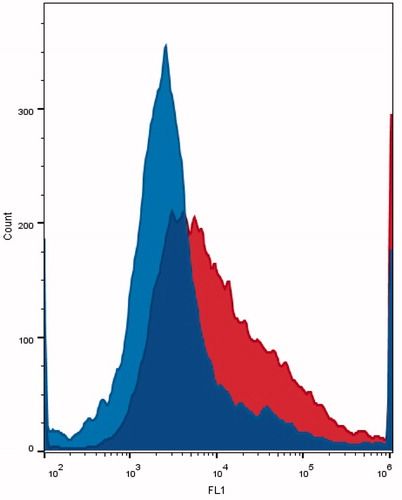
To confirm the importance of this pathway in the accumulation of ES-targeted liposomes in ER-positive breast cancer cells, targeted and non-targeted liposomes were incubated with ER-negative (MDA-MB-231) cells. The geometric means of the average cellular calcein uptake for ES-conjugated targeted and non-targeted liposomes were 2,351 ± 1,166 and 2,294 ± 285, respectively. These values were not statistically different (p = .47), which indicated that the accumulation of calcein in ER-negative cell lines is not affected by the presence of the targeting moiety (ES) on the surface of our DD system.
Estrogen receptors control cell proliferation [Citation71]. Research proved that 70% of breast cancers are ERα positive [Citation72], but for long, ERs were thought to localize only in nucleus and cytosol [Citation73–75]. Recent studies confirmed the presence of plasma membrane ERs, in which 5–10% of total ER is found at the plasma membrane [Citation76,Citation77]. Thus, our results support the receptor-mediated endocytosis triggered by the binding of ES with the plasma membrane ERα.
The utilization of ES-conjugated liposomes to deliver doxorubicin in breast cancer research was already proposed by Rai et al. [Citation78] and Paliwal et al. [Citation17]. The former study dealt with preparing PC-based liposomes with a DSPE-ES conjugate as the active lipid used (in contrast to the DSPE-PEG-CC-ES prepared in this study). Their liposomes had a similar size and were found to accumulate 13.9 times higher in the breast and 12.7 times in the uterus when compared to the administration of free drug. The latter study [Citation17], prepared liposomes that are PC-based with PEGylated lipid components to avoid carriers’ accumulation in the heart. They conducted a cytotoxicity test using three formulations: estrone anchored stealth liposomes encapsulating doxorubicin (ES-SL-DOX), stealth liposomes encapsulating doxorubicin (SL-DOX), and free DOX on both MCF-7 and MDA-MB-231 cell lines with 4 h of incubation. The study showed a higher cytotoxicity of ES-SL-DOX compared to the other two formulations in MCF-7, while cell viability in MDA-MB-231 reflected a non-significant difference in the uptake of ES-SL-DOX and SL-DOX. The results reported by Paliwal et al. [Citation17] agree with our findings, thus proving the targeting ability of estrone in ER-positive breast cancer cell line.
In this work, we go one step further to propose a multimodal drug delivery system that uses ES to actively target the cancer and ultrasound to trigger the release of the agent once the nanocarrier reaches the diseased site.
Cellular uptake of ES-conjugated calcein liposomes after ultrasound exposure
Sonication of MCF-7 cell plates incubated with ES-conjugated calcein liposomes floating in a 40 kHz water bath significantly increased the intracellular uptake of calcein (p = 1.026 × 10–3). An example of the flow cytometry histograms of MCF-7 cells incubated with these targeted nanoparticles before and after sonication are shown in . The average geometric mean of the peak fluorescence for the sonicated and control cells were 55,148 ± 14,625 and 36,357 ± 11,409, respectively, which represents an increase of 70% in calcein accumulation in MCF-7 cells after ultrasound exposure.
Figure 7. Calcein uptake of MCF-7 incubated with ES-conjugated liposomes before sonication (on the left) and after sonication (on the right). Ultrasound exposure conditions: 40-kHz water bath for 60 s (pulsed sonication at 10 s on and 20 s off cycles).
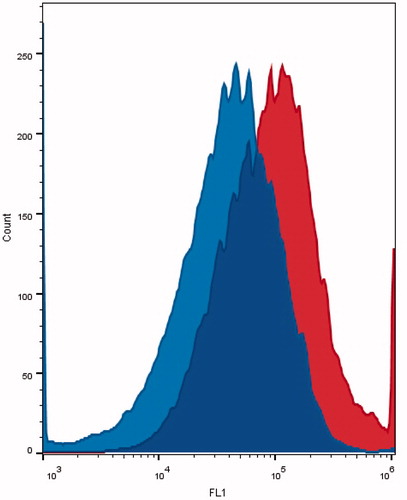
Several mechanisms could play a part in causing the US-induced release and uptake observed. Many studies showed that US can enhance the permeability of cell membranes through a phenomenon known as sonoporation, which results from cavitation events. Sonoporation can be reversible or irreversible. In reversible sonoporation, the cell can heal the damage in the membrane caused by the formation of micropores as a result of bubble implosion upon US exposure [Citation79–81]. Hence, sonoporation improves the uptake of large molecules by human cells. A study conducted by Jelenc et al. [Citation81] investigated the effect of LFUS on cell morphology and uptake. In the study, mouse melanoma B16-F1 cells incubated with propidium iodide (PI) were exposed to 29.6 kHz US at an intensity of 21.1 W/cm2 for 300 s. Cells with intact membrane morphology were detected by a fluorescence microscope, and some showed a significant level of fluorescence due to PI uptake. Normally, PI cannot penetrate through cell membrane unless it is damaged, thus the study proved the enhanced permeability of cell membranes caused by reversible sonoporation. Fan et al. [Citation82,Citation83] conducted similar studies in sonoporation, and they were able to control and quantify the pore size and the resealing rates of pores of a plasma membrane (HEK-293) targeted by microbubbles and upon exposure to ultrasound. In another study, Liu et al. [Citation84] proved reversible sonoporation of erythrocytes once exposed to US at 24 kHz.
The effect of sonoporation on the cell accumulation of small molecules like calcein or doxorubicin is highly controversial, however. It is argued that the cell retention of small molecules is compromised by the presence of micropores. The drug concentration inside the sonicated cells is reported to be higher than the extracellular concentration. The poration of the cell membrane can lead to the leaking of free drug if the molecule is not already attached to a cell structure like the DNA to satisfy the thermodynamic equilibrium established across the cell membrane [Citation37].
Conclusions
The novel liposomes proposed in this article are echogenic nanoparticles conjugated with the estrone bioligand of native origin to avert opsonization, allow long circulation of the carrier containing the chemotherapeutic drug and efficiently target the ER-positive breast cancer cells. After the demonstration of the targetability of the new echogenic estrone liposomes, the synergetic effect of the receptor mediator targeting and ultrasound stimulation was also proved in ER-positive cell line. This combination effect leads to a 70% improvement on cell uptake when liposomes and cells were simultaneously exposed to US.
Our results suggest that the biomedical approach based on the use of focused ultrasound on the diseased site after chemotherapeutic-based nanoparticle injection could be developed with the focus on the therapeutic improvement of ER-positive breast cancer.
To improve the potential of clinical application of this novel drug delivery system, the optimization of US parameters, including the acoustic frequency, power density and pulse duration, to enhance drug release and cellular uptake is underway. Also, the decrease in accumulation of calcein under certain US-exposure conditions, probably due to exocytosis [Citation80] or leakage of the calcein from the cells, needs to be addressed through proper optimization of US-exposure conditions, nanoparticle composition and drug concentration.
Abbreviation | ||
ER | = | Estrogen receptor |
LUV | = | large unilamellar vesicles |
LFUS | = | low frequency ultrasound |
DD | = | drug delivery |
RES | = | reticulo-endothelial system |
PEG | = | polyethylene glycol |
EPR | = | enhanced permeability and retention |
mAb | = | monoclonal antibody |
eLiposomes | = | emulsion liposomes |
HIFU | = | high-intensity focused US |
ES | = | estrone |
DSPE-PEG2000-NH2 | = | 1,2-distearoyl-sn-glycero-3-phosphoethanolamine-N-[amino (polyethylene glycol)-2000] |
DPPC | = | 1,2-dipalmitoyl-sn-glycero-3-phosphocholine |
CC | = | 2,4,6 trichloro-1,3,5 triazine (cyanuric chloride) |
TEA | = | triethylamine |
PBS | = | phosphate buffered saline |
TX-100 | = | Triton X-100 |
Supporting_Information_for_Publication.pdf
Download PDF (991.6 KB)Acknowledgements
The authors would like to acknowledge the support received by the American University of Sharjah Faculty Research Grant 1 (AUS/FRG1–2012); Patient’s Friends Committee, Sharjah; Al Jalila Foundation (AJF201555); and Al Qasimi Foundation.
Disclosure statement
No potential conflict of interest was reported by the authors.
Additional information
Funding
References
- Cukierman E, Khan DR. The benefits and challenges associated with the use of drug delivery systems in cancer therapy. Biochem Pharmacol. 2010;80:762–770.
- Haghiralsadat F, Amoabediny G, Helder MN, et al. A comprehensive mathematical model of drug release kinetics from nanoliposomes, derived from optimization studies of cationic PEGylated liposomal doxorubicin formulations for drug-gene delivery. Artif Cells Nanomed Biotechnol. 2017;46:169–177.
- Xu Y, Meng H. Paclitaxel-loaded stealth liposomes: development, characterization, pharmacokinetics, and biodistribution. Artif Cells Nanomed Biotechnol. 2016;44:350–355.
- Staples BJ, Pitt WG, Roeder BL, et al. Distribution of doxorubicin in rats undergoing ultrasonic drug delivery. J Pharm Sci. 2010;99:3122–3131.
- Kim D, Friedman D, Liu R. Tetraspecific ligand for tumor-targeted delivery of nanomaterials. Biomaterials. 2014;35:6026–6036.
- Moussa HG, Martins AM, Husseini GA. Review on triggered liposomal drug delivery with a focus on ultrasound. Curr Cancer Drug Targets. 2015;15:282–313.
- Connor J, Yatvin MB, Huang L. pH-sensitive liposomes: acid-induced liposome fusion. Proc Natl Acad Sci USA. 1984;81:1715–1718.
- Needham D, Anyarambhatla G, Kong G, et al. A new temperature-sensitive liposome for use with mild hyperthermia: characterization and testing in a human tumor xenograft model. Cancer Res. 2000;60:1197–1201.
- Ghadiali GE, Stevens MM. Enzyme-responsive nanoparticle systems. Adv Mater. 2008;20:4359–4363.
- Gerasimov OV, Boomer JA, Qualls MM, et al. Cytosolic drug delivery using pH- and light-sensitive liposomes. Adv Drug Deliv Rev. 1999;38:317–338.
- Ahmed S, Moussa HG, Martins AM, et al. Effect of pH, ultrasound frequency and power density on the release of calcein from stealth liposomes. Eur J Nanomed. 2016;8:31–43.
- Lasic D. Liposomes synthetic lipid microspheres serve as multipurpose vehicles for the delivery of drugs, genetic material and cosmetics. Am Sci. 1992;80:20–31.
- Mishra GP, Bagui M, Tamboli V, et al. Recent applications of liposomes in ophthalmic drug delivery. J Drug Deliv. 2011;2011:1–14.
- Rai S, Paliwal R, Vaidya B, et al. Estrogen(s) and analogs as a non-immunogenic endogenous ligand in targeted drug/DNA delivery. Curr Med Chem. 2007;14:2095–2109.
- Luconi M, Forti G, Baldi E. Genomic and nongenomic effects of estrogens: molecular mechanisms of action and clinical implications for male reproduction. J Steroid Biochem Mol Biol. 2002;80:369–381.
- Björnström L, Sjöberg M. Mechanisms of estrogen receptor signaling: convergence of genomic and nongenomic actions on target genes. Mol Endocrinol. 2005;19:833–842.
- Paliwal SR, Paliwal R, Mishra N, et al. A novel cancer targeting approach based on estrone anchored stealth liposome for site-specific breast cancer therapy.Curr. Cancer Drug Targets. 2010;10:343–353.
- Barnes RB, Levrant SG. Pharmacology of estrogens. In: Lobo, RA, editor. Treatment of the postmenopausal woman: basic and clinical aspects. 3rd ed. California: Elsevier; 2007. p. 767.
- Berkson DL. Safe hormones smart women. New York: iUniverse, Inc.; 2010. p. 31.
- Song Z, Lin Y, Zhang X, et al. Cyclic RGD peptide-modified liposomal drug delivery system for targeted oral apatinib administration: enhanced cellular uptake and improved therapeutic effects. Int J Nanomedicine. 2017;12:1941–1958.
- Chang D-K, Li P-C, Lu R-M, et al. Peptide-mediated liposomal doxorubicin enhances drug delivery efficiency and therapeutic efficacy in animal models. PLoS One. 2013;8:e83239.
- Li J-L, Wang L, Liu X-Y, et al. In vitro cancer cell imaging and therapy using transferrin-conjugated gold nanoparticles. Cancer Lett. 2009;274:319–326.
- Wu J, Lu Y, Lee A, et al. Reversal of multidrug resistance by transferrin-conjugated liposomes co-encapsulating doxorubicin and verapamil. J Pharm Pharm Sci. 2007;10:350–357.
- Choi M, Shin DH, Kim J-S. Repositioning of zoledronic acid for breast cancer using transferrin-conjugated liposome. J Pharm Investig. 2013;43:461–469.
- Reddy BS, Banerjee R. 17Beta-estradiol-associated stealth-liposomal delivery of anticancer gene to breast cancer cells. Angew Chem Int Ed Engl. 2005;44:6723–6727.
- Jain AS, Goel PN, Shah SM, et al. Tamoxifen guided liposomes for targeting encapsulated anticancer agent to estrogen receptor positive breast cancer cells: in vitro and in vivo evaluation. Biomed Pharmacother. 2014;68:429–438.
- Goren D, Horowitz AT, Tzemach D, et al. Nuclear delivery of doxorubicin via folate targeted-liposomes with bypass of multidrug-resistance efflux pump. Clin Cancer Res. 2000;6:1949–1957.
- Jiang J, Yang SJ, Wang JC, et al. Sequential treatment of drug-resistant tumors with RGD-modified liposomes containing siRNA or doxorubicin. Eur J Pharm Biopharm. 2010;76:170–178.
- Veneti E, Tu RS, Auguste DT. RGD-targeted liposome binding and uptake on breast cancer cells is dependent on elastin linker secondary structure. Bioconjugate Chem. 2016;27:1813–1821.
- Hare JI, Moase EH, Allen TM. Targeting combinations of liposomal drugs to both tumor vasculature cells and tumor cells for the treatment of HER2-positive breast cancer. J Drug Targeting. 2013;21:87–96.
- Shroff K, Kokkoli E. PEGylated liposomal doxorubicin targeted to α5β1-expressing MDA-MB-231 breast cancer cells. Langmuir. 2012;28:4729–4736.
- Li H-F, Wu C, Xia M, et al. Targeted and controlled drug delivery using a temperature and ultra-violet responsive liposome with excellent breast cancer suppressing ability. RSC Adv. 2015;5:27630–27639.
- Eliaz RE, Szoka FC Jr. Liposome-encapsulated doxorubicin targeted to CD44: a strategy to kill CD44-overexpressing tumor cells. Cancer Res. 2001;61:2592–2601.
- Frenkel V. Ultrasound mediated delivery of drugs and genes to solid tumors. Adv Drug Delivery Rev. 2008;60:1193–1208.
- Afadzi M, Strand SP, Nilssen EA, et al. Mechanisms of the ultrasound-mediated intracellular delivery of liposomes and dextrans. IEEE Trans Ultrason Ferroelectr Freq Control. 2013;60:21–33.
- Frenkel V, Etherington A, Greene M, et al. Delivery of liposomal doxorubicin (Doxil) in a breast cancer tumor model: investigation of potential enhancement by pulsed-high intensity focused ultrasound exposure. Acad Radiol. 2006;13:469–479.
- Chandrapala J, Martin GJO, Zisu B, et al. The effect of ultrasound on casein micelle integrity. J Dairy Sci. 2012;95:6882–6890.
- Evjen T. Sonosensitive liposomes for ultrasound-mediated drug delivery. Ph.D. [dissertation]. Norway: University of Tromsø; 2011.
- Graham SM, Carlisle R, Choi JJ, et al. Inertial cavitation to non-invasively trigger and monitor intratumoral release of drug from intravenously delivered liposomes. J Controlled Release. 2014;178:101–107.
- Husseini GA, Pitt WG. The use of ultrasound and micelles in cancer treatment. J Nanosci Nanotechnol. 2008;8: 2205–2215.
- Husseini GA, Diaz de la Rosa MA, Richardson ES, et al. The role of cavitation in acoustically activated drug delivery. J Control Release. 2005;107:253–261.
- Husseini GA, Myrup GD, Pitt WG, et al. Factors affecting acoustically triggered release of drugs from polymeric micelles. J Controlled Release. 2000;69:43–52.
- Husseini GA, Rapoport NY, Christensen DA, et al. Kinetics of ultrasonic release of doxorubicin from pluronic P105 micelles. Colloids Surf B. 2002;24:253–264.
- Khaibullina A, Jang BS, Sun H, et al. Pulsed high-intensity focused ultrasound enhances uptake of radiolabeled monoclonal antibody to human epidermoid tumor in nude mice. J Nucl Med. 2008;49:295–302.
- Kost J, Leong K, Langer R. Ultrasonically controlled polymeric drug delivery. Makromol Chem Macromol Symp. 1988;19:275–285.
- Rapoport N, Payne A, Dillon C, et al. Focused ultrasound-mediated drug delivery to pancreatic cancer in a mouse model. J Ther Ultrasound. 2013;1:1–11.
- Schroeder A, Kost J, Barenholz Y. Ultrasound, liposomes, and drug delivery: principles for using ultrasound to control the release of drugs from liposomes. Chem Phys Lipids. 2009;162:1–16.
- Oerlemans C, Deckers R, Storm G, et al. Evidence for a new mechanism behind HIFU-triggered release from liposomes. J Control Release. 2013;168:327–333.
- Kramer JF. Ultrasound: evaluation of its mechanical and thermal effects. Arch Phys Med Rehabil. 1984;65:223–227.
- Schlicher RK, Radhakrishna H, Tolentino TP, et al. Mechanism of intracellular delivery by acoustic cavitation. Ultrasound Med Biol. 2006;32:915–924.
- Blotny G. Recent applications of 2,4,6-trichloro-1,3,5-triazine and its derivatives in organic synthesis. Tetrahedron. 2006;62:9507–9522.
- Lasch J, Weissig V, Brandl M. Chapter 1: preparation of liposomes. In: Torchilin VP, Weissig V, editors. Liposomes: a practical approach. 2nd ed. Oxford: Oxford University Press; 2003. p. 3–15.
- Cullis PR, Mayer LD, Bally MB, et al. Generating and loading of liposomal systems for drug-delivery applications. Adv Drug Delivery Rev. 1989;3:267–282.
- Bhardwaj A, Kumar L, Narang RK, et al. Development and characterization of ligand-appended liposomes for multiple drug therapy for pulmonary tuberculosis. Artif Cells Nanomed Biotechnol. 2013;41:52–59.
- Barenolz Y, Haran G, inventors; Yissum Research Development Co of Hebrew University, assignee. Method of amphiphatic drug loading in liposomes by pH gradient. U.S. Patent 5,192,549 A. 1993 March 9.
- Stewart JC. Colorimetric determination of phospholipids with ammonium ferrothiocyanate. Anal Biochem. 1980;104:10–14.
- Monteiro N, Martins A, Reis RL, et al. Liposomes in tissue engineering and regenerative medicine. J R Soc Interface. 2014;11:1–24.
- Schroeder A, Avnir Y, Weisman S, et al. Controlling liposomal drug release with low frequency ultrasound: mechanism and feasibility. Langmuir. 2007;23:4019–4025.
- Richardson ES, Pitt WG, Woodbury DJ. The role of cavitation in liposome formation. Biophys J. 2007;93:4100–4107.
- Pitt WG, Husseini GA, Kherbeck LN. Ultrasound-triggered release from micelles. In: Lorenzo C, Concheiro A, editors. Smart materials for drug delivery. Vol. 1. Cambridge: Royal Society of Chemistry; 2013. p. 148–178.
- Marin A, Sun H, Husseini GA, et al. Drug delivery in pluronic micelles: effect of high-frequency ultrasound on drug release from micelles and intracellular uptake. J Controlled Release. 2002;84:39–47.
- Rapoport N. Ultrasound-mediated micellar drug delivery. Int J Hyperthermia. 2012;28:374–385.
- Tanbour R, Martins AM, Pitt WG, et al. Drug delivery systems based on polymeric micelles and ultrasound: a review. Curr Pharm Des. 2016;22:2796–2807.
- Martins AM, Tanbour R, Elkhodiry MA, et al. Ultrasound-induced doxorubicin release from folate-targeted and non-targeted P105 micelles: a modelling study. Eur J Nanomed. 2016;8:17–29.
- Elkhodiry MA, Momah CC, Suwaidi SR, et al. Synergistic nanomedicine: passive, active, and ultrasound-triggered drug delivery in cancer treatment. J Nanosci Nanotechnol. 2016;16:1–18.
- Martins AM, Ahmed S, Vitor RF, et al. Ultrasonic drug delivery using micelles and liposomes. In: Ashokkumar M, editor. Handbook of ultrasonics and sonochemistry. Singapore: Springer; 2016. p. 1–35.
- Troxell EM, Troxell ML, Bell JD. The effect of estrone on the interaction between phospholipase A and the phospholipid bilayer. J Undergrad Res Brigham Young Univ. 2013.
- Boyer TD, Zakim D, Vessey DA. Direct, rapid transfer of estrone from liposomes to microsomes. J Biol Chem. 1980;255:627–631.
- Biltonen RL, Lichtenberg D. The use of differential scanning calorimetry as a tool to characterize liposome preparation. Chem Phys Lipids. 1993;64:129–142.
- Kastantin M, Ananthanarayanan B, Karmali P, et al. Effect of the lipid chain melting transition on the stability of DSPE-PEG(2000) micelles. Langmuir. 2009;25:7279–7286.
- Lee HR, Hwang KA, Park MA, et al. Treatment with bisphenol A and methoxychlor results in the growth of human breast cancer cells and alteration of the expression of cell cycle-related genes, cyclin D1 and p21, via an estrogen receptor-dependent signaling pathway. Int J Mol Med. 2012;29:883–890.
- DeSantis C, Siegel R, Bandi P, et al. Breast cancer statistics, 2011. CA Cancer J Clin. 2011;61:409–418.
- Sumida C, Magdelenat H, Pasqualini JR. Cytosol and nuclear estrogen receptors (occupied and unoccupied sites) and progesterone receptors in human breast cancer. Breast Cancer Res Treat. 1985;5:165–169.
- Beato M, Herrlich P, Schütz G. Steroid hormone receptors: many actors in search of a plot. Cell. 1995;83:851–857.
- Tsai MJ, O’Malley BW. Molecular mechanism of action of steroid/thyroid receptor superfamily members. Annu Rev Biochem. 1994;63:451–486.
- Levin ER. Plasma membrane estrogen receptors. Trends Endocrinol Metab. 2009;20:477–482.
- Song RX, Santen RJ. Membrane initiated estrogen signaling in breast cancer. Biol Reprod. 2006;75:9–16.
- Rai S, Paliwal R, Vaidya B, et al. Targeted delivery of doxorubicin via estrone-appended liposomes. J Drug Targeting. 2008;16:455–463.
- Tachibana K, Uchida T, Ogawa K, et al. Induction of cell-membrane porosity by ultrasound. Lancet. 1999;353:1409
- Lentacker ID, Cock I, Deckers RD, et al. Understanding ultrasound induced sonoporation: definitions and underlying mechanisms. Adv Drug Delivery Rev. 2014;72:49–64.
- Jelenc J, Miklavcic D, Lebar AM. Low-Frequency Ultrasound in vitro: changes of cell morphology. LAHA. 2013;2013:58–60.
- Fan Z, Liu H, Mayer M, et al. Spatiotemporally controlled single cell sonoporation. Proc Natl Acad Sci USA. 2012;109:16486–16491.
- Fan Z, Kumon RE, Park J, et al. Intracellular delivery and calcium transients generated in sonoporation facilitated by microbubbles. J Controlled Release. 2010;142:31–39.
- Liu J, Lewis TN, Prausnitz MR. Non-invasive assessment and control of ultrasound-mediated membrane permeabilization. Pharm Res. 1998;15:918–924.