Abstract
Angiogenesis and osteogenesis in tissue-engineered bone are the key factors in the clinical application of tissue-engineering technology to repair large bone defects. In vivo cells that are farther than 200 μm from capillaries cannot survive due to lack of nutrients and oxygen, and thus, the tissue-engineered bone is not suitable for repairing large bone defects. In this study, we constructed a novel artificial bone scaffold loaded with superparamagnetic plasmid gene microspheres. Magnetic micro-movement of the magnetic microspheres in the scaffold was generated by an oscillating magnetic field and a static magnetic field to promote the release of plasmid genes from microspheres for transfection of surrounding cells, resulting in protein expression of vascular endothelial growth factor, thus promoting angiogenesis and osteogenesis in the scaffold, internal vascularization of the artificial bone scaffold and repair of large bone defects.
Introduction
The incidence of hard tissue defects due to trauma, infection and tumor is high, seriously affecting the physical and mental health of patients. Conventional bone grafting methods have limitations, such as increased trauma and insufficient tissue sources, so there is a great demand for hard tissue biomaterials, including bone implants. Research on new medical repair materials using regenerative medicine approaches, including biological materials and tissue engineering for the repair of large bone defects, indicates broad prospects for development. After years of unremitting efforts, researchers developed a variety of bone tissue repair materials [Citation1,Citation2]. However, no ideal bone tissue repair material is currently available for clinical application.
The three basic processes after bone grafting are graft vascularization, bone regeneration and bone fusion [Citation3,Citation4]. Many studies using fundamental experiments and clinical applications have shown that the key to the success of bone regeneration is the early vascularization of the graft; this vascularization affects the entire transplant repair process and plays a decisive role in the result of bone regeneration and bone fusion [Citation5,Citation6]. To achieve early vascularization of the graft, researchers have tested a variety of methods. For example, the pre-vascularized tissue engineering bone was designed [Citation7,Citation8]; however, due to the high technical requirements for microsurgery and the limited tissue materials with blood vessels, its clinical promotion was difficult. Some researchers used a graft combined with the co-culture of endothelial cells, osteoblasts, a variety of stem cells and cytokines along with gene therapy [Citation9–13], but the operation is complex and time-consuming, limiting its clinical application. More importantly, in vivo cells farther than 200 μm from the capillaries cannot survive because of the lack of nutrients and oxygen [Citation14]; thus, in vitro structures of tissue-engineered bone are generally smaller than 5 mm × 5 mm × 5 mm, preventing them from meeting the clinical needs to repair large bone defects.
As research in drug controlled-release technology and non-viral vectors has progressed, biodegradable, controlled-gene-releasing superparamagnetic gene microspheres have become the most promising carriers to perform cell functions in scaffolds [Citation6,Citation15]. It is possible to bring revolutionary innovations to tissue engineering research. Chitosan has good biocompatibility, can effectively bind to DNA to protect the DNA from nuclease degradation, and can be transfected into human cells. Superparamagnetic nanoparticles, as agents containing both active and passive targets, have a specific surface area effect and a magnetic effect [Citation16–19]. When the diameter of a magnetic iron oxide (Fe3O4) crystal is less than 30 nm, the crystal is superparamagnetic [Citation20], and can be used to enrich the loaded drug in the target tissue when an external magnetic field is applied [Citation21–23].
Based on the above theory, we proposed the application of an in vitro non-invasive oscillating magnetic field and static magnetic field to drive the micro-movement of magnetic gene-loaded microspheres inside an artificial bone scaffold to promote the release of plasmid genes from microspheres for transfection of surrounding cells. The in vitro release and local enrichment of the plasmid in vascular endothelial growth factor (VEGF) magnetic microspheres was observed in the presence of an external magnetic field (oscillating magnetic field and static magnetic field). Promotion of the in vivo vascularization and osteogenesis of the artificial bone was also observed. The superparamagnetic chitosan pDsVEGF165-green-N1 gelatin microspheres (SPCPGM) released and caused enrichment of the plasmid for local transfection with the application of the in vitro magnetic field, and this effect was further elucidated. In addition, the functional mechanism was investigated to evaluate the effect of SPCPGM in promoting the vascularization and osteogenesis of artificial bone under the oscillating magnetic field and static magnetic field; this application is expected to provide a new type of angiogenesis strategy and method for bone tissue engineering research.
Methods
Preparation and characterization of the superparamagnetic (nano-Fe3O4) chitosan gene vector
SPFCN was prepared by the co-precipitation method [Citation24–27]: a 57.40 g mixture of ammonium ferrous sulphate and ammonium ferric sulphate [Fe2+/Fe3+ (mol) = 1.2] was dissolved with 2% chitosan (w/v) in acetic acid solution (pH 5.5) to obtain solution A, which was transferred into a 500 ml three-necked flask; 6 mol/l NaOH was prepared as solution B; and under the protection of a nitrogen atmosphere, solution A was heated to 55 °C with vigorous stirring. Solution B was added dropwise into solution A to react for 10 min. The stirring speed was lowered, and the reaction mixture was heated to 85 °C for 90 min. An appropriate amount of glutaraldehyde was added to react for 30 min. The pH of the resulting suspension was adjusted to 5.5 with diluted hydrochloric acid. The obtained black colloidal precipitate was washed with distilled water and dried under vacuum to form a dry powder, which was stored at room temperature.
The diameter and distribution of the particles were measured using a laser diffraction particle size analyser. The SPFCN morphology was observed by TEM. The structural characteristics of the samples were evaluated using Fourier transform infrared spectroscopy (FT-IR 8900, Shimadzu Co., Ltd., Shanghai, China).
Construction of superparamagnetic chitosan plasmid (pReceiver-VEGF165/DH5a) complex
The pReceiver-VEGF165/DH5a plasmid gene was amplified and purified. SPFCN (nitrogen content of 0.007 g/l by the Kjeldahl method) was dissolved in sodium acetate buffer (pH 5.4) and heated to 55 °C. The 20% (w/v) concentrated plasmid was heated to 55 °C. The plasmid and SPFCN were mixed in different nitrogen/phosphorus ratios by vortexing for 20 s, followed by a reaction at 55 °C for 1 h. The binding of the plasmid to SPFCN was observed by agarose gel electrophoresis.
In vitro transfection of the superparamagnetic chitosan plasmid (pReceiver- VEGF165/DH5a) complex
HUVEC-1 and human osteogenic sarcoma cells (MG-63) were seeded onto 12-well cell culture plates at 1 × 105 cells per well. After 24 h of culturing, gene transfection was conducted, with a negative control for both types of cells. The prepared SPFCN was added to the sample wells, with 20 μl per well. The naked plasmid was added to the negative control wells. Then, the cell culture plate was placed in a CO2 incubator for culture, and the medium was replaced with 1 ml fresh medium every 48 h [Citation18,Citation23,24]. The cells were routinely observed for the generation of red fluorescent protein using inverted fluorescence microscopy. Four days after the transfection, the transfection efficiency of the plasmid was determined by flow cytometry. Some cells were fixed with 4% glutaraldehyde and observed using TEM.
Preparation of superparamagnetic chitosan plasmid (pReceiver-VEGF165/DH5a) gelatin microspheres (SPCPGM)
SPCPGM was prepared using cross-linking and solidification methods [Citation24–27]. The procedures of these methods were as follows: magnetic chitosan nanoparticles (approximately 800 mg) were added to 5 ml acetic acid buffer (pH 5.5) and heated to 55 °C; 8 ml 20% (w/v) concentrated plasmid (pDsVEGF165-Red1-N1) was heated to 55 °C; and they were mixed by vortexing for 20 s, followed by a binding reaction for 1 h. Preheated 20% gelatin solution (w/v) at 50 °C was added and mixed to form a composite emulsion. This composite emulsion was added to paraffin oil (50 °C) preheated with homogenizer (10,000 rpm). After the resulting microspheres were observed microscopically, the temperature was lowered to 4 °C using an ice bath. An appropriate amount of 37% formaldehyde and isopropanol was added, and the stirring was continued for 1 h. The samples were washed 5–8 times with ether and deionized water (the washing solution was saved to determine its plasmid content). The microspheres were collected by centrifugation, lyophilized and stored at −20 °C. The superparamagnetic chitosan was replaced with chitosan to prepare CPGM.
In vitro release of SPCPGM under a magnetic field
The sample (2.00 mg; SPCPGM containing approximately 1.06 mg plasmid) was placed in a 25 ml Erlenmeyer flask, and 10 ml PBS (0.01 M, pH 7.4) was added prior to incubation in a constant temperature water bath (37 °C) at an oscillation frequency of 100 times/min. A 0.5 ml sample was collected at the assigned time point, and PBS was supplemented to maintain the volume of the eluted solution. The content of the plasmid in the eluate was measured using a SmartSpec™ 3000 Nuclide Protein Analyzer (Bio-Rad Laboratories, Inc., California, USA) (260 nm). The daily and accumulated plasmid release values were calculated, and an in vitro release curve was prepared. For the experiments using an oscillating magnetic field [Citation28,Citation29], the oscillating magnetic field was generated by a rotating permanent magnet, with a magnetic field strength of 800 mT at 50–80 rpm. An oscillating magnetic field disturbance was applied 1 h before each sampling. The CPGM (containing approximately 1.30 mg plasmid) and the non-oscillating magnetic field interference both served as controls.
In vitro release and enrichment of SPCPGM under the influence of a magnetic field
A porous bone implant scaffold was prepared using weakly crystallized, nonstoichiometric apatite and a new collagen-like polyamide composite (provided by Nanobiomaterials Research Center of Sichuan University, China). Next, 2.0 mg SPCPGM was loaded into the porous bone implant scaffold. The scaffold containing SPCPGM was fixed in the middle of a plastic pipe (length of 30 cm and diameter of 2.0 cm), and its long axis was the same as the long axis of the plastic pipe. On top of the plastic pipe, a 0.3 cm hole was drilled every 1 cm for sample collection. A static magnetic field with a magnetic field strength of 100 mT was placed at the midpoint of the pipe. In a 37 °C water bath, 25 ml acetic acid pepsin solution (0.25 g pepsin in 50 ml 0.1 mol/l acetic acid/0.1 mol/l sodium acetate) (pH 5.5) was added to the plastic pipe, which was kept in the dark on a stand. On the third day, a 500 μl sample was collected from each hole, and the plasmid concentration was determined [Citation30]. The CPGM (containing approximately 1.30 mg plasmid) and the non-oscillating magnetic field interference each served as control conditions. SPCPGM served as the control for zero calibration.
In vivo study of the release of microspheres controlled by the superparamagnetic chitosan plasmid gelatin to promote artificial vascularization and osteogenesis
In total, 36 adult New Zealand rabbits, weighing 2.2–2.5 kg, male or female, were randomly divided into three groups, with 12 rabbits in each group. The model of a large radius defect was established. A bone scaffold loaded with SPCPGM was implanted into the bone defect. A button-shaped NdFeB permanent magnet (static magnetic field of 200 mT) was continuously placed outside the bone defect for 8 weeks. During the first 2 weeks, the animals were treated with an oscillating magnetic field (800 mT) generated by a programmed and digitized magnetic field generator for 1 h once a day. The control groups received no magnetic field or no SPCPGM.
At 2, 4, 6, 8 and 12 postoperative weeks, the animals were anesthetized using Sumianxin (0.2–0.3 ml/kg, by intramuscular injection). After the perfusion of blood vessels for ink staining, the animals were sacrificed by an auricular vein injection of air. The general healing situation of the implant and the surrounding tissue was observed. The scaffold was cut in some cases to observe the growth of the vessel in the hollow part of the scaffold. After X-ray images were obtained, the samples were fixed with 4% paraformaldehyde, and sectioning was performed for histopathological observation. The neovascularization was observed using CD31 immunohistochemical staining and ink staining of the sections. HE staining was used to observe the osteogenesis. The image analysis software system Tiger 920 G (Tiger institute of image engineering technology, Chongqing, China) was used to analyse the images, and the area of the new bone was determined using a planimeter [Citation31].
Statistical analysis
We used Student’s t-test to compare the differences in drug loading and in vitro transfection efficiencies. For the other statistical analyses, we used one-way ANOVA to determine significance. When the p values were .05 or less, the differences were considered statistically significant. All analyses were performed using Statview software Version 5.0 (Abacus Concept Inc., Berkeley, CA, USA).
Results
Preparation and characteristics of superparamagnetic iron oxide chitosan particles (SIOCPs)
SIOCPs were prepared by co-precipitation [Citation26]. By optimizing the preparation parameters, magnetic Fe3O4 chitosan microparticles with an average particle size of 46 ± 24 μm were prepared. The particles were nearly spherical and aggregated (). The magnetization curve of the sample () indicated that the magnetic Fe3O4 chitosan microparticles were superparamagnetic. The infrared spectroscopy results showed that the specific vibrational absorption peaks of Fe3O4 crystal and chitosan were observed in superparamagnetic Fe3O4 chitosan nanoparticles (SPFCNs). The coating procedure successfully combined chitosan and Fe3O4 nanoparticles to generate chitosan Fe3O4 nanoparticles.
Preparation of superparamagnetic chitosan particles/plasmid complexes
The human VEGF165-green fluorescent fusion protein eukaryotic expression plasmid (pReceiver-VEGF165/DH5a) in the concentration of 0.30 ± 0.05 mg/ml was electrostatically recombined with SIOCPs in different ratios of nitrogen to phosphorus, and the binding of SIOCPs was observed by agarose gel electrophoresis. The results showed that the minimum ratio of nitrogen to phosphorus for the complete binding of SIOCPs to plasmid was 2.5/1 (). The nitrogen/phosphorus ratio of 2.5/1 was used in the subsequent experiments to construct the plasmid complexes of superparamagnetic chitosan nanoparticles.
Figure 2. In vitro magnetic transfection of superparamagnetic chitosan nanoparticle complexes. (A) Plasmid binding experiment, with the marker λEcoT14 I digest. (B) Atomic force microscopy structural diagram. (C) Cell phagocytosis of the superparamagnetic chitosan plasmid complex (×1000) under a transmission electron microscope. (D) HUVEC-1 cells engulfed the superparamagnetic chitosan plasmid complexes to form endosomes (×1000). (E) MG-63 cells engulfed the superparamagnetic chitosan plasmid complexes to form endosomes (×1000). (F) Cell magnetic transfection observed under an inverted fluorescence microscope. (G) Magnetic transfection efficiency.
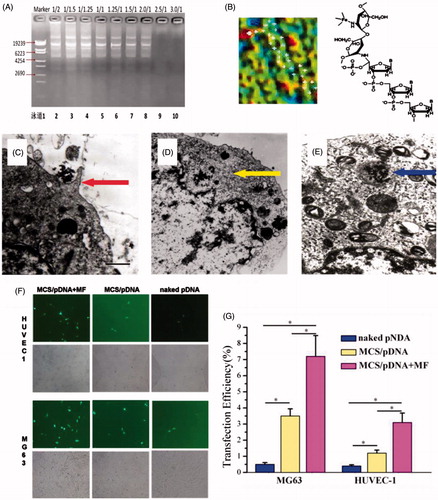
shows the atomic force microscopy structure of the superparamagnetic chitosan complex. The iron atom may form a covalent bond with the NH– in chitosan, which is consistent with the results of infrared spectroscopy. The resulting superparamagnetic chitosan nanoparticles were electrostatically adsorbed onto the plasmid to successfully construct the superparamagnetic chitosan plasmid complexes. The constructed superparamagnetic chitosan plasmid complex was used in the subsequent study.
In vitro magnetic transfection
In this study, human umbilical vein endothelial cells (HUVEC-1) and human osteosarcoma cells (MG-63) were selected as the study subjects for the in vitro transfection of the superparamagnetic chitosan plasmid complex under a static magnetic field. The observation of the cell transfection by inverted fluorescence microscopy showed that fluorescence was emitted from both HUVEC-1 cells and MG-63 cells, indicating that the complex was phagocytized into the cells. As shown in , the fluorescence intensity of the HUVEC-1 cells decreased in the order magnetic chitosan/plasmid DNA + magnetic field (MCS/pDNA + MF) > MCS/pDNA > naked pDNA, which is consistent with the order of the fluorescence intensity in the MG-63 cells. The transmission electron microscopy results showed that pseudopodia formed in the cells (); thus, the MCS/pDNA complex was encapsulated, and endosomes formed ().
The transfection efficiency of the plasmid (pReceiver-VEGF165/DH5a) was determined by flow cytometry. As shown in , the transfection efficiencies of HUVEC-1 cells and MG-63 cells for naked plasmid were 0.5 ± 0.11% and 0.4 ± 0.08%, respectively. After the plasmid was coated with SIOCPs, the transfection efficiencies were increased to 3.5 ± 0.45% (p < .05) and 1.2 ± 0.19% (p < .05), respectively. Under a magnetic field, the transfection efficiencies were further increased to 7.2 ± 1.29% (p < .05) and 3.1 ± 0.58% (p < .05), respectively. The MCS/pDNA complex could enhance the transfection efficiency of the plasmid.
In vitro release and distribution
The average diameter of superparamagnetic chitosan pDsVEGF165-green-N1 gelatin microspheres (SPCPGM) was 65.36 ± 20.93 μm, and they were spherical. In the absence of a magnetic field, the dispersion was good (). Under the influence of a magnetic field, the microspheres were in a beaded arrangement along the magnetic flux (). The encapsulation efficiency of SPCPGM was 98.51 ± 1.34% (w/w), and the drug loading capacity was 53.42 ± 1.54% (w/w). The encapsulation efficiency of the non-magnetic chitosan pDsVEGF165-Red1-N1 plasmid gelatin microspheres (CPGM) was 97.56 ± 1.69% (w/w), and the drug loading capacity was 65.20 ± 1.52% (w/w). There was no significant difference in the encapsulation efficiency between the two types of microspheres (p > .05), but the drug loading capacities were significantly different (p < .001). The drug loading capacity of SPCPGM was relatively low, mainly due to the addition of nano-Fe3O4, which increased the weight of the microspheres, thus reducing the percentage of the plasmid in the microcapsules and resulting in a decreased drug loading capacity.
Figure 3. Study on the in vitro release of the superparamagnetic chitosan nanoparticle complex. (A) Distribution with no magnetic field. (B) Distribution under the influence of a magnetic field. (C) In vitro release curves. (D) Scanning electron microscopy results before the release. (E) Scanning electron microscopy results after the in vitro release.
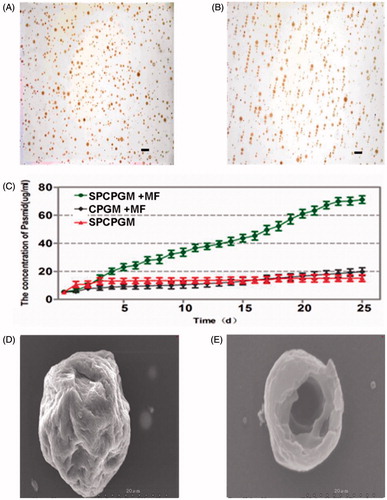
The results for the in vitro release of SPCPGM are shown in . Under the influence of a magnetic field, SPCPGM could continue to promote the in vitro release of the plasmid for 25 days, with the cumulative release of the plasmid being 4–5 times higher than that without a magnetic field. The morphology of the microspheres after the release was observed by electron microscopy, and the microspheres had a hollow structure after the release of the plasmid ().
The results of the in vitro enrichment experiments are shown in . At day 3, concentrations of plasmid at 1 cm and 3 cm for the SPCPGM + MF group were significantly higher than those for the non-magnetic field group, but the plasmid concentration at 5 cm was significantly lower than that for the SPCPGM group. Under a magnetic field, the SPCPGM + MF group showed a significant concentration gradient of the plasmid at the detection sites, and the difference in the plasmid concentration between 3 cm and 5 cm was approximately 30 μg/ml. However, there was no significant difference in the concentration gradient between the SPCPGM group and the CPGM group, and the difference in the plasmid concentration between 3 cm and 5 cm was less than 5 μg/ml. The plasmid was thus enriched in a radius of approximately 3 cm under the influence of a static magnetic field of 100 millitesla.
In vivo angiogenesis and osteogenesis
An artificial bone scaffold in a hollow structure with lateral holes was prepared using nano-hydroxyapatite/polyamide bone cement with good histocompatibility [Citation31] (), and SPCPGM was loaded into the hollow portion of the scaffold (). A model of a large segmental radius bone defect was established in New Zealand rabbits [Citation31]. The artificial bone scaffold loaded with SPCPGM was implanted (), and the angiogenesis and osteogenesis results of the artificial bone scaffold were observed under the influence of magnetic field.
Figure 5. Repair of a radius defect in a New Zealand white rabbit with an artificial bone scaffold loaded with superparamagnetic chitosan pDsVEGF165-Red1-N1 gelatin microspheres. (A) Artificial bone scaffold (B) Artificial bone scaffold loaded with superparamagnetic chitosan pDsVEGF165-Red1-N1 gelatin microspheres. (C) The artificial bone scaffold loaded with superparamagnetic chitosan pDsVEGF165-Red1-N1 gelatin microspheres was implanted in the radial bone defect of a New Zealand rabbit. (D) General observation at 6 postoperative weeks. (E) X-ray images.
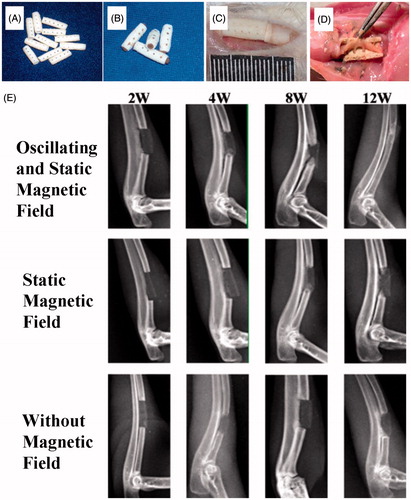
A postoperative radiograph is shown in . At 12 weeks after the operation, the two bone fragments of the radial bone defect were completely connected under the influence of a magnetic field, and most of the bone marrow cavity had re-canalized. This result indicated that the artificial bone scaffold had achieved the repair of the bone defect. Without the influence of a magnetic field alone, the bone fragments of the radial bone defect were not fully connected. In the absence of SPCPGM, only a certain amount of bone callus was formed at the ulnar side and the proximal fracture of the bone defect.
Ink staining is a good method to observe the localized neovascularization in a bone defect [Citation32,Citation33]. The results of the postoperative ink staining are shown in . The Tiger 920 G image analysis software system was used to analyze the ink-staining images, and the results are shown in . From week 2 to week 8, the vessel density of the group with a magnetic field was significantly higher than that of the group without a magnetic field and the group with no SPCPGM. The results of the CD31 immunohistochemical staining () were similar to those of the ink staining. The image analysis of the ink staining and CD31 immunohistochemical staining, combined with the results of the in vitro transfection and enriching experiments, showed that under the influence of a magnetic field, the superparamagnetic chitosan plasmid gelatin microspheres promoted angiogenesis of the artificial bone scaffold at the tissue defect.
Figure 6. Repair of a radius defect in a New Zealand white rabbit with an artificial bone scaffold loaded with superparamagnetic chitosan pDsVEGF165-Red1-N1 gelatin microspheres. (A) With the effect of magnetic field and superparamagnetic chitosan pDsVEGF165-Red1-N1 gelatin microspheres, ink staining at 2 postoperative weeks. (B) Without the effect of magnetic field, ink staining at 4 postoperative weeks. (C) With the effect of superparamagnetic chitosan pDsVEGF165-Red1-N1 gelatin microspheres, ink staining at 8 postoperative weeks. (D) Postoperative ink staining pictures were analyzed by Tiger 920G image analysis software system. (E) CD31 immunohistochemical staining. (F) With the effect of magnetic field and superparamagnetic chitosan pDsVEGF165-Red1-N1 gelatin microspheres, haematoxylin and eosin (HE) staining at 8 postoperative weeks. (G) Without the effect of magnetic field, HE staining at 12 postoperative weeks. (H) Area density of the new bone tissue.
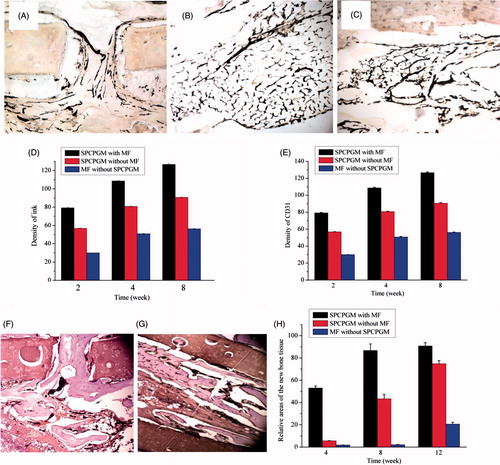
The postoperative histological observation is shown in . The relative areas of the new bone tissue were measured using a planimeter. With the progression of time, the new bone tissues in each group gradually increased. At week 8, additional cartilage callus and osteoid formed in the group with a magnetic field, and the new bone tissue occupied approximately 86.80% of the artificial bone scaffold body cavity. A bone wedge-like structure was formed at the lateral hole of the implant, while only a few instances of new bone tissue formed near the bone defect in the groups without a magnetic field or SPCPGM. At week 12, more than 90% of the space of the artificial bone scaffold in the group with a magnetic field was occupied by the new bone tissue. The new bone tissue was plasticized to form a mature trabecular bone structure. The new bone trabeculae in the mesh were interconnected to form a network and plate, with no significant boundaries at the original bone defect. The new bone tissue in the group without a magnetic field occupied approximately 74.80% of the total cavity of the artificial bone scaffold, and the new bone tissue formed fine trabeculae. The new bone tissue in the group with no SPCPGM occupied only approximately 21%. The amount of new bone tissue in the group with a magnetic field was significantly higher than the amounts in the group with no magnetic field or SPCPGM. The magnetic field together with SPCPGM could significantly improve the effect of the artificial bone scaffold in repairing large segmental bone defects.
Discussion
In the presence of a magnetic field, the transfection efficiency was significantly improved, which is consistent with the findings reported in the literature [Citation18,Citation19,Citation34]. Chitosan could encapsulate the plasmid DNA in its microparticles, thus avoiding the degradation by nuclease. Chitosan could destroy endosomes through the swelling effect, causing the release of the plasmid DNA into the cytoplasm and thus significantly improving the transfection efficiency of the plasmid. SIOCPs carry a positive charge. SIOCPs can bind not only to a plasmid with a negative charge through electrostatic adsorption but also to the surface of negatively charged cell membranes through electrostatic adsorption, thus bridging the cell and the plasmid and facilitating the process of phagocytosis [Citation19]. Under a magnetic field, the MCS/pDNA complex can closely contact the cells in a short period of time, which can also increase the local concentration of DNA and greatly accelerate the DNA transfection process.
In this study, SPCPGM released the chitosan plasmid complex through natural degradation in the absence of a magnetic field, whose local concentration was low. Considering the in vivo destruction by various enzymes and the loss by diffusion, only a small amount of released chitosan plasmid complex remained, so the local angiogenesis was relatively weak. With a magnetic field, the plasmid was enriched, and thus, the local concentration of plasmid was relatively high, resulting in the improved angiogenesis compared to that of the condition without a magnetic field. With the combined effect of the static magnetic field and the oscillating magnetic field, the chitosan plasmid complex was released and enriched at a high localized concentration; thus, the angiogenesis was faster and greater.
The results of this study showed that under a magnetic field, SPCPGM could promote neovascularization and osteogenesis in the artificial bone scaffold. At week 8, the vascular surface density was 126.69 ± 0.93, and new tissue and new bone could be found throughout the entire artificial bone scaffold; thus, a large segmental bone defect could be completely repaired. Without a magnetic field, the 8-week vascular surface density was 90.64 ± 0.58. In the absence of SPCPGM, the 8-week vascular surface density was only 56.23 ± 0.49, and osteogenesis only occurred at the ulnar side outside the artificial bone scaffold where the blood circulation was relatively abundant. These results fully demonstrated that the magnetic micro-movement could promote the plasmid release of SPCPGM and the local transfection, promote the completion of neovascularization and osteogenesis within the large artificial bone scaffold, and successfully lead to in vivo vascularization and osteogenesis in a large artificial bone scaffold (). However, Meng et al. found that super-paramagnetic c-Fe2O3 nanoparticles incorporated in the nanofibers endowed the scaffolds super-paramagnetic responsive under the applied static magnetic field, which accelerated new bone tissue formation and remodeling in the rabbit defect [Citation35]. In this study, magnetic micro-movement was successfully used to stimulate plasmid release from microspheres, and local enrichment of the magnetic plasmid for the transfection of the surrounding cells in a large artificial bone scaffold through three mechanisms: the magnetic susceptibility of the magnetic microspheres in the scaffold, the slow release of the plasmid gene, and the magnetic transfection. The VEGF165 growth factor was expressed in the transfected cells, eventually leading to vascularization in the scaffold and thus promoting osteogenesis. This study describes the development of a highly efficient non-invasive system for nutrient exchange, slow gene release and gene delivery with magnetic micro-movement under the influence of a magnetic field, which is a promising strategy and method for a new type of angiogenesis for various implants. Thus, the volume of the artificial engineered tissue implants can meet the clinically needed size range, and this technique is expected to be widely applied in the repair of large bone tissue defects.
Figure 7. The mechanism of the promotion of angiogenesis and osteogenesis in the scaffold by the magnetic micro-movement and magnetic transfection of the magnetic microspheres in an oscillating magnetic field. The wave symbols indicate that the magnetic microspheres generate magnetic micro-movement in an oscillating magnetic field; the magenta arrows indicate that the magnetic field facilitates the magnetic transfection of the plasmids.
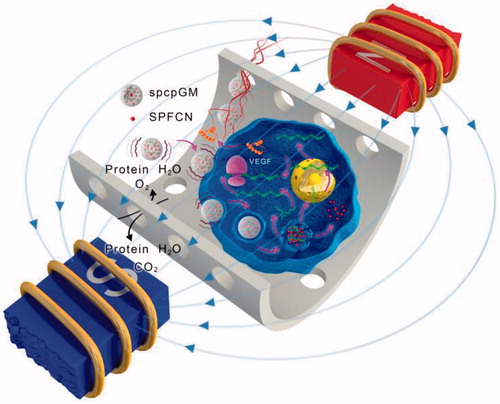
Disclosure statement
No potential conflict of interest was reported by the authors.
Additional information
Funding
References
- Xu XL, Qiu SJ, Zhang YX, et al. PELA microspheres with encapsulated arginine-chitosan/pBMP-2 nanoparticles induce pBMP-2 controlled-release, transfected osteoblastic progenitor cells, and promoted osteogenic differentiation. Artif Cells Nanomed Biotechnol. 2017;45(2):330–339.
- Henkel J, Woodruff MA, Epari DR, et al. Bone regeneration based on tissue engineering conceptions – a 21st century perspective. Bone Res. 2013;1:216–248.
- Gross TP, Cox QG, Jinnah RH. History and current application of bone transplantation. Orthopedics. 1993;16:895–900.
- Kirkeby OJ, Nordsletten L, Skjeldal S, et al. Circulation in corticocancellous bone grafts measured with laser Doppler flowmetry. An experimental study in rats. Scand J Plast Reconstr Surg Hand Surg. 1994;28:249–254.
- Cassell OC, Hofer SO, Morrison WA, et al. Vascularisation of tissue-engineered grafts: the regulation of angiogenesis in reconstructive surgery and in disease states. Br J Plast Surg. 2002;55:603–610.
- Garcia JR, Clark AY, Garcia AJ. Integrin-specific hydrogels functionalized with VEGF for vascularization and bone regeneration of critical-size bone defects. J Biomed Mater Res A. 2016;104:1845.
- Safavi-Abbasi S, Komune N, Archer J, et al. Surgical anatomy and utility of pedicled vascularized tissue flaps for multilayered repair of skull base defects. J Neurosurg. 2016;125:419–430.
- Barabaschi GD, Manoharan V, Li Q, et al. Engineering pre-vascularized scaffolds for bone regeneration. Adv Exp Med Biol. 2015;881:79–94.
- Perez RA, Kim JH, Buitrago JO, et al. Novel therapeutic core-shell hydrogel scaffolds with sequential delivery of cobalt and bone morphogenetic protein-2 for synergistic bone regeneration. Acta Biomater. 2015;23:295–308.
- Lim SS, Kook SH, Bhattarai G, et al. Local delivery of COMP-angiopoietin 1 accelerates new bone formation in rat calvarial defects. J Biomed Mater Res A. 2015;103:2942–2951.
- Zhang W, Chang Q, Xu L, et al. Graphene oxide-copper nanocomposite-coated porous CaP scaffold for vascularized bone regeneration via activation of Hif-1alpha. Adv Healthc Mater. 2016;5:1299–1309.
- Almubarak S, Nethercott H, Freeberg M, et al. Tissue engineering strategies for promoting vascularized bone regeneration. Bone. 2016;83:197–209.
- Sun X, Kang Y, Bao J, et al. Modeling vascularized bone regeneration within a porous biodegradable CaP scaffold loaded with growth factors. Biomaterials. 2013;34:4971–4981.
- Chow DC, Wenning LA, Miller WM, et al. Modeling pO2 distributions in the bone marrow hematopoietic compartment. I. Krogh's Model. Biophys J. 2001;81:675–684.
- Vlad M, Andronescu E, Grumezescu AM, et al. Carboxymethyl-cellulose/Fe3o4 nanostructures for antimicrobial substances delivery. Bio-Med Mater Eng. 2014;24:1639–1646.
- Alves NM, Mano JF. Chitosan derivatives obtained by chemical modifications for biomedical and environmental applications. Int J Biol Macromol. 2008;43:401–414.
- Yu H, VandeVord PJ, Mao L, et al. Improved tissue-engineered bone regeneration by endothelial cell mediated vascularization. Biomaterials. 2009;30:508–517.
- Mura S. Stimuli-responsive nanocarriers for drug delivery. Nat Mater. 2013;12:991.
- Casettari L, Vllasaliu D, Lam JK, et al. Biomedical applications of amino acid-modified chitosans: a review. Biomaterials. 2012;33:7565–7583.
- Benyettou F, Lalatonne Y, Sainte-Catherine O, et al. Superparamagnetic nanovector with anti-cancer properties: gamma Fe2O3@Zoledronate. Int J Pharm. 2009;379:324–327.
- Shapiro B. Towards dynamic control of magnetic fields to focus magnetic carriers to targets deep inside the body. J Magn Magn Mater. 2009;321:1594–1594.
- Namiki Y, Namiki T, Yoshida H, et al. A novel magnetic crystal-lipid nanostructure for magnetically guided in vivo gene delivery. Nat Nanotechol. 2009;4:598–606.
- Ghosh D, Lee Y, Thomas S, et al. M13-templated magnetic nanoparticles for targeted in vivo imaging of prostate cancer. Nat Nanotechnol. 2012;7:677–682.
- Chao SC, Wang MJ, Pai NS, et al. Preparation and characterization of gelatin-hydroxyapatite composite microspheres for hard tissue repair. Mater Sci Eng C Mater Biol Appl. 2015;57:113–122.
- Sima M, Fatemeh ZS, Samad MF, et al. Current methods for synthesis of magnetic nanoparticles. Artif Cells Nanomed Biotechnol. 2016;44(2):722–734.
- Guo L, Liu G, Hong RY, et al. Preparation and characterization of chitosan poly(acrylic acid) magnetic microspheres. Mar Drugs. 2010;8:2212–2222.
- Dratviman-Storobinsky O, Lubin BCRA, Hasanreisoglu M, et al. Effect of subconjuctival and intraocular bevacizumab injection on angiogenic gene expression levels in a mouse model of corneal neovascularization. Mol Vis. 2009;15:2326–2338.
- Heiss M, Hellstrom M, Kalén M, et al. Endothelial cell spheroids as a versatile tool to study angiogenesis in vitro. FASEB J. 2015;29:3076–3084.
- Finotelli PV, et al. Microcapsules of alginate/chitosan containing magnetic nanoparticles for controlled release of insulin. Colloids Surf B Biointerfaces. 2010;81:206–211.
- Engebrecht J, Heilig JS, Brent R. Preparation of bacterial plasmid DNA. Curr Protoc Neurosci. 2001;Appendix 1:A.1J.1–A.1J.10.
- Itou A, Azuma H, Isono M, et al. Comparison of measuring an area with a planimeter and by rectangular dimensional methods. Nihon Jibiinkoka Gakkai Kaiho. 1996;99:926–933.
- He X, Dziak R, Yuan X, et al. BMP2 genetically engineered MSCs and EPCs promote vascularized bone regeneration in rat critical-sized calvarial bone defects. PLoS One. 2013;8:e60473.
- Berggren KL, Kay JJ, Swain RA. Examining cerebral angiogenesis in response to physical exercise. Methods Mol Biol. 2014;1135:139–154.
- Fatemeh ZS, Sima M, Nasrin N, et al. Magnetic nanoparticles as potential candidates for biomedical and biological applications. Artificial Cells, Nanomedicines, and Biotechnology. 2016;44(3):918.
- Meng J, Xiao B, Zhang Y, et al. Super-paramagnetic responsive nanofibrous scaffolds under static magnetic field enhance osteogenesis for bone repair in vivo. Sci Rep. 2013;3:2655.