Abstract
A novel nanoparticle (Au-LTSL-GA.A) uses the thermosensitive liposome (LTSL) to encapsulate ganoderic acid A (GA.A), which successfully transforms the polarity of GA.A and has excellent water solubility. The multifunctional Au-LTSL-GA.A, a self-assembled thermal nanomaterial, was used in antibacterial and anticancer applications in combination with near-infrared (NIR) irradiation. The designed Au-LTSL-GA.A nanoparticle was used as a nano-photosensitizer to achieve synergistic photochemotherapy based on the phototherapy sensitization property of Au nanorods (NRs) and antitumour activity of GA.A. In the antibacterial experiments, the Au-LTSL-GA.A + NIR irradiation had a broad-spectrum antibacterial effect, exhibiting a strong antibacterial activity against drug-resistant Escherichia coli (E. coli) and Staphylococcus aureus (S. aureus) compared with the raw GA.A and LTSL-GA.A. In the anticancer experiments, Au-LTSL-GA.A + NIR irradiation, which combined phototherapy sensitization property of Au NRs with antitumour activity of GA.A, exhibited high anticancer activity against MCF-7 cells. The IC50 value of Au-LTSL-GA.A + NIR irradiation (12.1 ± 1.3 μg/mL) was almost similar to cisplatin in MCF-7 cells. The evaluation of the potential in vivo toxicity of Au-LTSL-GA.A revealed no toxicity in mice. The results of this study suggest that Au-LTSL-GA.A has a wide range of potential industrial and clinical applications, such as in antibacterial treatment and cancer photochemotherapy.
Introduction
The lingzhi mushroom is a traditional Chinese medicinal mushroom and has been recognized as a valuable source of inhibitory lead compounds against multi-drug resistance bacterial and cancer [Citation1–5]. Most of the potential active compounds are extracted from one of its component fungal species, namely Ganoderma lucidum. Among them, ganoderic acid (GA) is the most potent therapeutic biomolecule known [Citation6]. Ganoderic acid A (GA.A) is a highly oxygenated C30 lanostane-type triterpenoid, which has significant antibacterial, anticancer, anti-HIV, antioxidation and anti-inflammatory activities [Citation2,Citation4,Citation7,Citation8]. Due to the extremely low water solubility of GA.A, the bioavailability of raw GA.A is low, which limits its utilization in the pharmaceutical industries. However, such a limitation can be overcome, to a certain extent, with the novel nanoparticle (NP)-based drug delivery system, loaded with active drugs, which can greatly improve bioavailability.
NP-based drug delivery systems (including liposomes) are capable of targeting anticancer drugs to tumour; antibacterial drugs to bacteria, thus their development and optimization have attracted increasing interest in the field of drug delivery [Citation9–13]. To increase the effectiveness of drug delivery to the target treatment area and minimize side effects, target-specific drug delivery is essential [Citation14]. Lysolipid-containing thermosensitive liposomes (LTSL) function as drug delivery vehicles by protecting the drug from degradation during blood circulation, and rapidly releasing the drug in the target area [Citation15–18]. Multifunctional LTSL carriers coated with a hydrophilic polymer, such as polyethylene glycol, show prolonged survival in the circulation due to a stealth effect by reducing the conditioning effect on the surface for protection [Citation19,Citation20]. The thermosensitive drug delivery method is stable at 37 °C, keeps the drug in the liposome during blood transport, and releases it at higher temperatures (45 °C) in an ultrafast manner so as to counteract the rapid blood passage time and prevent washout from the targeted tumour [Citation21–23]. However, releasing drugs from LTSL under high-temperature conditions requires sufficient external heating. Traditional external heating is not uniform, and it is not possible to target the heating to a single cell and have a large harmful effect on the receptor. Therefore, a green and safe, stable and uniform and a high-efficiency heating method is worth exploring. Some studies have also reported a liposome-gold nanorod vesicular system that consists of liposomes and gold nanorods (Au NRs) designed to allow deep tissue detection, therapy, and monitoring in living animals [Citation24,Citation25]. Au NRs as a kind of plasmonic material with different compositions, structures, shapes, and surface coatings can be used to generate heat upon excitation by near-infrared (NIR) irradiation [Citation26–28]. The liposome-Au NR vesicular system can be used as a self-assembled thermal nanomaterial for thermosensitive drug delivery.
In this work, GA.A was encapsulated into LTSL to form LTSL-GA.A in order to enhance the intracellular delivery efficiency of GA.A. Then, Au NRs were modified to form a new liposome-Au NR vesicular system structure on the surface of the thermosensitive liposomes as a self-assembled thermal nanomaterial. This new heating method has the advantages of providing green environmental protection, uniform and stable heat production, and having low side effects compared with the traditional heating method. Additionally, the Au-LTSL-GA.A was synthesized and characterized. Then, the antibacterial and antitumour activities of the Au-LTSL-GA.A nanoparticles in combination with NIR irradiation were evaluated. The novel Au-LTSL- GA.A nanoparticles mainly use the thermosensitive liposome (LTSL) to encapsulate the GA.A, which successfully transforms the polarity of the GA.A, causing it to have good water solubility. Thus, through the modification of the Au NRs combined with the use of NIR irradiation, a self-assembled thermal nanomaterial with high activity can be prepared.
Materials and methods
Materials
Au NRs were synthesized using a modified seed-mediated method. The reagents 1,2-Dipalmitoyl-sn-glycero-3-phosphatidylcholine (DPPC), 1,2-Distearoyl-sn-glycero-3-phosphocholine (DSPC), 1,2-distearoyl-sn-glycero-3-phosphoethanolamine-N-[methoxy-(polyethylene glycol)-2000] (DSPE-PEG2000) were purchased from Sigma-Aldrich Chemical (Saint Louis, MO, USA). GA.A was purchased from (Aladdin Shanghai Biochemical Technology Co., Ltd, Shanghai, China). Dimethyl thiazolyl diphenyl tetrazolium bromide (MTT), propidium iodide (PI), Calcein and Alexa Fluor 488 annexin V were purchased from Sigma-Aldrich Chemical. All aqueous solutions were prepared in double-distilled water, which was obtained from a Milli-Q water purifying system (Millipore Corporation, Bedford, MA, USA). All other reagents were of the best commercially available grade.
Synthesis of Au NRs-LTSL-GA.A
The liposomes were prepared by the film hydration method. The film formulated with DPPC/DSPC/DSPE-PEG2000 = 9:1:1(mol ratio), and GA.A was encapsulated into liposomes at 1:20 (w/w, drug/lipid). Briefly, lipids were dissolved in trichloromethane under a stream of nitrogen gas for 5 min, and then the solvent was removed under reduced pressure. The thin lipid film was dried under vacuum overnight to get rid of residual organic solvent and then hydrated at 60 °C for 2 h with PBS with or without Au NRs (1 mg/mL). The new liposome (Au-LTSL-GA.A) suspensions were ultrasonicated and subsequently passed through a 0.22-μm membrane filter to control liposome size [Citation11,Citation25].
Characterization of Au NRs-LTSL-GA.A
The characteristic UV-Vis spectra were recorded using a UV-Vis spectrophotometer (Agilent Technologies, Santa Clara, CA, USA), Fourier transform infrared spectroscopy (FTIR) analysis was performed on a Spectrum GX FTIR spectrometer (Perkin-Elmer, Waltham, MA, USA). The morphology of the nanoparticles and liposomes was characterized by transmission electron microscopy (TEM) using a Hitachi HT7700 transmission electron microscope (Hitachi Corp, Tokyo, Japan) operated at 80 kV, and by scanning electron microscopy (SEM), using a Hitachi S-4800 scanning electron microscope (Hitachi Corp.) at an acceleration voltage of 3.0 kV. The average particle size, size distribution, and zeta potential were detected using a Malvern Zetasizer Nano ZS (Malvern Instruments, Malvern, Worcs., UK) at room temperature [Citation29].
Bacterial cell culture
Drug-resistant Escherichia coli ATCC 8739 (E. coli), Staphylococcus aureus ATCC 6538 (S. aureus), Micrococcus tetragenus ATCC 2835 (M. tetragenus), Bacillus subtilis ATCC 6633 cell (B. subtilis) were obtained from the Anhui Agricultural University. The bacterial cells were grown to the logarithmic phase (log phase) by reinoculating the overnight culture (1:100) into fresh media and culturing them in a shaking incubator at 37 °C [Citation30].
Colony-forming unit (CFU) and minimum inhibitory concentration (MIC) assays
First, a log phase solution of drug-resistant bacteria (M. tetragenus, E. coli, B. subtilis and S. aureus) were inoculated at 1:40 in fresh LB culture medium containing 50 μg/mL of Au-LTSL-GA.A solution or the same concentration of other solutions (Au NRs, GA.A or LTSL-GA.A) with or without NIR irradiation and then incubated in a shaking incubator at 37 °C for 12 h. A solution with the same concentration of Au-LTSL-GA.A was incubated for 2 h and measured for 5 min upon irradiation (0.25 W/cm2) and then incubated in a shaking incubator at 37 °C for 12 h. The blank group was prepared in the same way but without any treatment. For each culture, LB agar plates containing the same number of the four kinds of bacterial cells of the above solutions were used as blank, and all experiments for each group were performed in a triplicate. Viable bacteria colony formation using the above number of cells in culture was analyzed by determining the CFU count. Every culture was plated in triplicate on LB agar plates after serial dilutions and incubated at 37 °C for 12 h and the formation of the bacterial colony was counted and recorded [Citation31]. The MICs of the different solutions (GA.A, Au-LTSL-GA.A, Au-LTSL-GA.A + NIR irradiation, ampicillin and kanamycin solution) were also determined by the tube dilution method. Briefly, log phase cells were spotted on a series of liquid LB containing dilutions of the reagents and incubated at 37 °C for 12 h. Kanamycin and ampicillin were used as positive control group. All measurements were performed in a triplicate or quadruplicate [Citation32].
Fluorescent-based live/dead antibacterial assay
Further analysis of the antibacterial properties of Au-LTSL-GA.A was performed using live/dead staining techniques for E. coli and S. aureus. Log phase cells treated with GA.A (50 μg/mL), LTSL-GA.A (50 μg/mL), Au-LTSL-GA.A (50 μg/mL) were used as control groups. Cells were treated with different concentrations of Au-LTSL-GA.A (30 and 50 μg/mL), incubated for 2 h and measured for 5 min upon irradiation (0.25 W/cm2). The blank group was prepared in a similar manner but without any treatment. The cells were collected and then washed three times with distilled water to remove traces of medium. Cells were stained (for 30 min) with SYTO9 (green) and PI (red) to assess antibacterial activity. The cell suspension without materials was used as a blank group. The cells were analyzed using an Olympus FV1000 laser scanning fluorescence microscope (Olympus Corp., Tokyo, Japan) [Citation33].
Observation of bacterial cell morphology by SEM, and TEM in antibacterial assay
SEM analysis was performed to evaluate the changes in the surface morphology of E. coli and S. aureus after each treatment. Log phase cells treated with GA.A (50 μg/mL), LTSL-GA.A (50 μg/mL), Au-LTSL-GA.A (50 μg/mL) were used as control groups. Cell were treated with Au-LTSL-GA.A (50 μg/mL), incubated for 2 h, and measured for 5 min upon irradiation (0.25 W/cm2). The blank group was prepared in the same manner but without any treatment. Cells were collected and fixed with a 2.5% glutaraldehyde solution at 4 °C overnight. Then, the samples were sequentially dehydrated by immersion in 30, 50, 70, 80, 90 and 100% ethanol for 5 min, followed by immersion in 100% acetone, and then immediately subjected to critical point drying (CPD), using a critical point drier (Emitech K850, Quorum Technologies, East Grinstead, East Sussex, UK). The samples were spread onto a microscope slide, airdried, and coated with gold under vacuum, followed by SEM examination [Citation34,Citation35]. Samples were prepared for TEM analysis following the procedure described for the antibacterial activity tests. Log phase cells treated with GA.A (50 μg/mL), or Au-LTSL-GA.A (50 μg/mL) were used as control groups. Cells were treated with different concentration of Au-LTSL-GA.A (30 and 50 μg/mL), incubated for 2 h and measured for 5 min upon irradiation (0.25 W/cm2). The blank group was prepared in the same way but without any treatment. The cells were fixed with 1% osmium tetroxide and 2% glutaraldehyde at room temperature and then visualized by TEM [Citation36].
Cell lines
The human cancer cell lines, MCF-7, Hela, A549 and BGC-823 were obtained from the Anhui Agricultural University. The normal fibroblasts (Hs68) were also obtained from Anhui Agricultural University. All cell lines were maintained in RPMI 1640 supplemented with fetal bovine serum (10%) at 37 °C in 5% CO2 incubator.
MTT and real time cell analysis
The cell toxicity of Au-LTSL-GA.A was determined in MCF-7 cells (2.0 × 103 per/well) incubated in 96-well plates for 24 h at 37 °C in a 5% CO2 incubator. Various Au-LTSL-GA.A, at different concentrations (0, 10, 20, 30, 40 and 50 µg/mL), were added into each well and incubated for 24 h after changing the cell culture medium. The same concentrations of GA.A, LTSL-GA.A, Au-LTSL-GA.A were the control groups. The raw NIR irradiation and Au NRs + NIR irradiation were also set as control groups. The blank group was treated with PBS. The cells in some wells were irradiated with an 880 nm laser (0.25 W/cm2) for 5 min after culturing for 2 h. Afterwards, the MTT solution (5 mg/mL, 20 µL) was added to each well and the plate was incubated for another 4 h. Each group was assayed in triplicate, and the IC50 values were obtained from the curves of the mean OD values of the triplicate measurements plotted versus drug concentration. The brightness of each group was obtained by confocal fluorescence microscopy [Citation37]. The cell proliferation was analyzed by real-time cellular analysis (RTCA), using a multielectrode array-based RTCA system (ACEA Biosciences, Inc., San Diego, CA, USA). Cells were plated at a density of 10,000/well with fresh medium and various formulations (GA.A, LTSL-GA.A and Au-LTSL-GA.A) to a final concentration of 30 μg/mL. The raw NIR irradiation and Au NRs + NIR irradiation was also set as control group. The Au-LTSL-GA.A treatment also included a set of different concentrations (10 and 30 μg/mL) and each group was irradiated with an 880 nm laser (0.25 W/cm2) for 5 min after culturing for 2 h. The blank group was PBS. Cells were incubated at 37 °C and 5% CO2 in the RTCA system cradle. The impedance signals were recorded every 10 min until the end of the experiment [Citation38].
Cancer cell live/dead assay by fluorescence microscopy
Calcein-AM/PI co-staining was used to determine the number of live and dead cancer cells, respectively. MCF-7 cells were seeded at a density of 2.0 × 104 cells/well in 48 well plates. The cells were then treated with Au-LTSL-GA.A (30 µg/mL), and the cells were irradiated with 880 nm laser (0.25 W/cm2) for 5 min after incubation for 2 h and then continued to culture for 12 h. The blank group was treated with PBS. Cells treated with NIR irradiation, GA.A (30 μg/mL), Au NRs + NIR irradiation (30 μg/mL), Au-LTSL-GA.A (30 μg/mL) were used as control groups. After removing the culture medium, Calcein-AM (10 ng/mL, 1 ml) and PI (10 ng/mL, 1 ml) were mixed and added into the wells of the culture dishes and incubated for 30 min in the dark. Then, all dishes were washed three times with PBS and observed by confocal laser scanning microscopy (CLSM) [Citation39]. (For Calcein–AM, Ex = 488 nm and Em = 515 nm; PI Ex = 535 nm and Em = 615 nm) [Citation27].
Cancer cell apoptosis study by flow cytometry
MCF-7 cells were grown in 6-well plates overnight at 37 °C and 5% CO2. The cells were then treated with Au-LTSL-GA.A (30 µg/mL), and irradiated with 880 nm laser (0.25 W/cm2) for 5 min after incubation for 2 h and then continued in culture for 12 h. The blank group was treated with PBS. Cells treated with NIR irradiation, GA.A (30 μg/mL), Au NRs + NIR irradiation (30 μg/mL), Au-LTSL-GA.A (30 μg/mL) were used as control groups. After treatment, the cells were collected and washed twice with PBS. Then, the cells were stained with PI and Annexin V–FITC and analyzed using a CytoFLEX analyzer (Beckman Coulter Inc., Brea, CA, USA) [Citation40]. (For PI, Ex = 535 nm and Em = 615 nm; Annexin V–FITC, Ex = 488 nm and Em = 525 nm)
Observation of cancer cell morphology by TEM in anticancer assay
Samples were prepared for TEM analysis following the procedure described for the anticancer activity assays. MCF-7 cells were grown in 6-well plates overnight at 37 °C and 5% CO2. The cells were then treated with Au-LTSL-GA.A (30 µg/mL), and irradiated with 880 nm laser (0.25 W/cm2) for 5 min after incubation for 2 h and then continued in culture for 12 h. The blank group was PBS. Cells treated with GA.A (30 μg/mL) and LTSL-GA.A (30 μg/mL) were used as control groups. After treatment, the cells were collected. The cells were fixed with 1% osmium tetroxide and 2% glutaraldehyde at room temperature and then visualized by TEM [Citation27].
Animal models
BALB/c male nude mice (∼25 g) were purchased from the Model Animal Research Center of Nanjing University and bred in an axenic environment for specific pathogen-free (SPF) animals. All animal experiments were conducted according to the protocols approved by Anhui Agricultural University Laboratory Animal Center (Permit Number: SYXK 2016–007).
In vivo drug toxicity studies
To evaluate the toxicity of Au-LTSL-GA.A + NIR irradiation, the mice were sacrificed after 30 days. The PBS treated mice were used as the blank group. Cells treated with NIR irradiation were used as the control group. The major organs (liver, spleen, kidneys, heart, and lungs) of the mice were collected and stained with hematoxylin and eosin (H&E). Blood samples were also collected, and uric acid, blood glucose, cholesterol, and alanine aminotransferase were measured.
Results
Preparation and characterization of Au-LTSL-GA.A
The self-assembled thermal nanoparticles, Au-LTSL-GA.A, was synthesized in this work. A complete list of the methods used to characterize the new particles is shown in . The novel liposome-nanoparticle has many advantages and was effectively used as an antibacterial and anticancer agent. The UV − vis spectrum presented in reveals the characteristic peak of Au NRs, with characteristic peaks present at ∼600 nm. Despite their small size, Au NRs exhibited a longitudinal surface plasmon resonance band at about 880 nm within the biologically transparent NIR spectral window. The insert image displays a TEM image showing the morphology of Au NRs. The characteristic peaks of GA.A and LTSL are also present in the UV − vis spectrum (), at 249 and 220 nm, respectively. However, UV–vis spectral analysis revealed strong UV absorbance, which was influenced by the LTSL-encapsulated GA.A. For instance, characteristic peaks were displaced after Au NRs were modified on LTSL-GA.A. The characteristic peak of the assembled Au-LTSL-GA.A was located at 286 nm. These results primarily confirmed that the synthesis of the Au-LTSL-GA.A nanoparticle was successfully completed. Additionally, the results of the FTIR spectroscopy analysis conducted to verify the presence of GA.A, LTSL, LTSL-GA.A and Au-LTSL-GA.A () revealed the red-shifting of the characteristic peaks for GA.A and LTSL, which further indicated the successful synthesis of the Au-LTSL-GA.A. The morphological characteristics of these nanoparticles were observed by SEM and TEM (). The TEM image of LTSL shown in reveals that it is monodispersed and varies in size from 200 to 300 nm. Also, as shown in the image of the Au-LTSL-GA.A nanoparticles shown in , the Au NRs are clearly attached to the surface of the liposome, and have a size of about 400 nm. An enlarged image of a single Au-LTSL-GA.A nanoparticle is shown in . The particle size distribution was further evaluated by dynamic light scattering (DLS) analysis. The DLS analysis revealed a particle size that is consistent with the observation by electron microscopy (). The zeta-potential measurements presented in reveal that the Au NRs (+36.8 ± 0.3 mV) are positively charged, while LTSL-GA.A (−38.7 ± 0.5 mV), and Au-LTSL-GA.A (−8.1 ± 0.1 mV) are negatively charged. Thus, the mechanism is that LTSL-GA.A was modified with positively charged Au NRs. The standard curve of GA.A concentration versus the UV-Vis absorbance of GA.A was prepared in order to study the encapsulation rate of Au-LTSL-GA.A. The results revealed that when the temperature reaches 45 °C, the release efficiency of GA.A can reach 96.8%, as shown in and . However, below 45 °C, the release efficiency is poor, indicating that the temperature-sensitive critical point of the Au-LTSL-GA.A is 45 °C. Through the absorption of NIR radiation Au NRs produce significant localized heating, making these specific particles extremely efficient “nano-heaters”. In order to determine the photothermal properties of Au-LTSL-GA.A, the photothermal responses of the Au NRs and Au-LTSL-GA.A particles were evaluated with a particle concentration of 30 μg/mL under irradiation with a NIR laser (880 nm, 250 mW/cm2). PBS was used as a control. Since the manner of heating can influence the response, the temperature of the solution upon irradiation was monitored. The Au-LTSL-GA.A solutions, subjected to 880 nm NIR irradiation for 5 min, showed a rise in temperature from 37 to 54 °C (ΔT = 17 °C), as shown in . Although the temperature will continue to increase as the irradiation time increases, a 5 min irradiation treatment is enough for the Au-LTSL-GA.A to release the encapsulated GA.A. We also investigated the effect of different irradiation times on the release of GA.A from Au-LTSL-GA.A (). The results indicated that as the irradiation time increases, the release of GA.A increases. When the irradiation time reached 5 min, the increase in the release of GA.A slowed down. Accordingly, a 5 min NIR irradiation was selected for the anticancer activity evaluation experiment.
Figure 1. Characterization of the prepared Au-LTSL-GA.A. (A) UV-Vis spectra of Au NRs. The extinction spectrum of Au NRs shows a localized surface plasmon resonance centered at 880 nm, which is within the biologically transparent spectral window. (B) FTIR spectra of LTSL, GA.A, LTSL- GA.A and Au-LTSL-GA.A after removing CTAB. (C) UV-Vis spectra of LTSL, GA.A, LTSL-GA.A and Au-LTSL-GA.A. (E) SEM image of LTSL-GA.A. (D, F) TEM images of Au-LTSL-GA.A. (G) The particle size distribution of LTSL-GA.A and Au-LTSL-GA.A in DLS image. (H) The Zeta potential of Au-LTSL-GA.A.
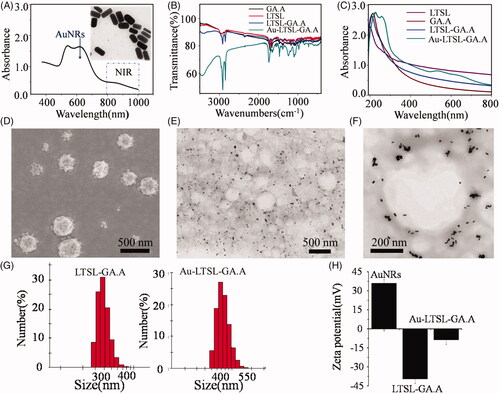
Figure 2. (A) Screening results for drug-resistant M. tetragenus, E. coli, B. subtilis, and S. aureus by CFU method, treated with Au-LTSL-GA.A (50 μg/mL). Error bars represent the standard deviations (n ≥ 3). (B) Photographs of bacterial colonies (E. coli and S. aureus were treated with GA.A, LTSL-GA.A, Au-LTSL-GA.A and Au-LTSL-GA.A + NIR irradiation) formed on LB-agar plates. Bacteria without NPs are blank. Cells were treated with different materials for 2 h in incubation and measured for 5 min upon irradiation (0.25 W/cm2).
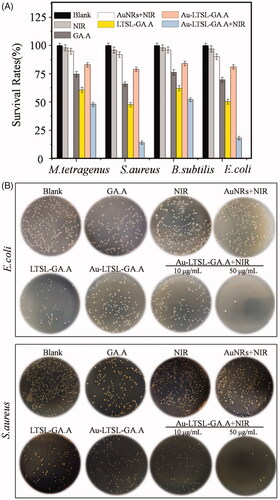
Figure 3. Fluorescence microscopic images of drug-resistant E. coli (A) and S. aureus (B) through the LIVE-DEAD stained assay. Cells treated with GA.A (50 μg/mL), LTSL-GA.A (50 μg/mL), Au-LTSL-GA.A (50 μg/mL) were set as control groups. Cells were treated with different concentration of Au-LTSL-GA.A (30 and 50 μg/mL) for 2 h in incubation and measured for 5 min upon irradiation (0.25 W/cm2). Cells were stained (for 30 min) with SYTO9 and PI for antibacterial activity. Overlay images were compared for the two kinds of cell. Bacteria without NPs were as blank.
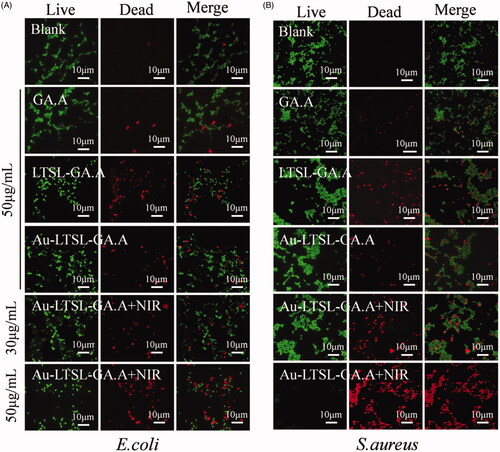
TABLE 1. MIC values (μg/mL) against drug-resistant E. coli and S. aureus.
Antibacterial activity of Au-LTSL-GA.A
GA.A has been reported as an effective antibacterial active compound. However, due to its low water solubility it has extremely low bioavailability. In this study, the polarity of GA.A was successfully changed so as to improve its antibacterial activity, as indicated by the results shown in . The determination of CFU count was also adopted in our study whenever bacterial cell viability was estimated throughout the study. As shown in , the results suggested the group treated with Au-LTSL-GA.A + NIR irradiation exhibited a broad-spectrum antibacterial effect, indicating that the treatment had strong antibacterial activity against drug-resistant E. coli and S. aureus compared with the blank and control treatment. However, NIR and Au NRs + NIR irradiation showed low antibacterial activity for drug-resistant E. coli and S. aureus. A possible reason is that the bacteria had strong heat-resistance activity. Thus, the treatment of the Au NRs + NIR irradiation group will not be performed in the last experiments. The results also showed that raw GA.A was successfully modified to increase its antibacterial activity. This result is also shown in the images of the CFUs (). Additionally, we also found that the antibacterial activity is enhanced as the concentration of the drug is increased. Indeed, when the concentration reached 50 μg/mL, the inhibition of E. coli and S. aureus was already very significant. The MIC values were also determined and are shown in . Specifically, the MIC value of the group treated with Au-LTSL-GA.A + NIR irradiation was 9.3 ± 0.26 μg/mL for E. coli and 7.5 ± 0.62 μg/mL for S. aureus. The MIC value of the group treated with Au-LTSL-GA.A + NIR irradiation against E. coli was lower than that of the groups treated with Au-LTSL-GA.A (27.5 ± 0.83 μg/mL), LTSL-GA.A group (20.6 ± 0.38 μg/mL), and GA.A group (19.9 ± 0.45 μg/mL). The MIC value of the group treated with Au-LTSL-GA.A + NIR irradiation against S. aureus was also lower than that of the groups treated with Au-LTSL-GA.A (36.7 ± 1.09 μg/mL), LTSL-GA.A group (23.1 ± 0.92 μg/mL), and GA.A group (21.9 ± 0.62 μg/mL). These results indicate that the treatment with Au-LTSL-GA.A + NIR has the predominant antibacterial activity. Thus, the Au-LTSL-GA.A + NIR treatment could become a new antibacterial nanoparticle for further antibacterial therapeutic research. Thus, these results additionally revealed that the inhibitory effect on drug-resistance against S. aureus was better than that against E. coli.
Fluorescence assay assesses antibacterial activity
To further assess the antibacterial activity of Au-LTSL-GA.A + NIR irradiation, we used fluorescent staining to evaluate the antibacterial activity by visual observation. Cells were stained with SYTO9 (green) to detect live cells and PI (red) to detect dead cells in order to evaluate the antibacterial activity. After treatment of the cells with different concentration of Au-LTSL-GA.A (30 and 50 μg/mL), incubated for 2 h and measured for 5 min upon irradiation (0.25 W/cm2), no dead cells (red fluorescence) were found in the blank group, as shown in . The control group showed a few dead cells, indicating weak antibacterial activity. However, when the bacterial cells were treated with Au-LTSL-GA.A + NIR irradiation, the number of dead cells was clearly increased. In addition, it was also determined that the number of dead cells was concentration dependent. We also found that the inhibitory effect was stronger against drug-resistant S. aureus than against E. coli as can be seen by comparing the results shown in . Thus, results showed that the antibacterial activity of GA.A was further improved after wrapping in Au-LTSL.
Anticancer activity of Au-LTSL-GA.A
GA.A exhibited excellent antitumour activity, but it also has low bioavailability due to its low water solubility. After GA.A was encapsulated by LTSL and transformed into a water-soluble material, the surface of the liposome was modified with Au NRs to obtain self-assembled thermal nanoparticles. The antitumour activity of the novel nanoparticles was evaluated using the MTT assay. The antitumour activity of Au-LTSL-GA.A + NIR irradiation on BGC-823, A549, Hela, Hs68 and MCF-7 cells was evaluated using the MTT cytotoxicity assay. As shown in , treatment with Au-LTSL-GA.A + NIR irradiation exhibited a broad spectrum of inhibitory activity on the tested cells, with IC50 values that were significantly lower than treated with Au NRs + NIR irradiation, GA.A, and Au-LTSL-GA.A. The cytotoxicity of the Au-LTSL-GA.A + NIR irradiation treatment increased ∼2–5 times compared to treatment with raw GA.A and Au-LTSL-GA.A. The anticancer activity of Au-LTSL-GA.A + NIR irradiation (12.1 ± 1.3 μg/mL) was almost similar to that of cisplatin (11.8 ± 3.5 μg/mL) on MCF-7 cell. The explanation for this result is that NIR irradiation cleaves LTSL, leading to the targeted release of GA.A, and the combined photothermal therapy from Au NRs thereby increasing the anti-tumour activity. The anticancer activity of these treatments on MCF-7 cells is also shown in . Treatment of MCF-7 cells with Au-LTSL-GA.A slowly decreased the viability of the cells with increasing Au-LTSL-GA.A concentration. However, the survival rate of MCF-7 cells that were treated with Au-LTSL-GA.A, and then irradiated with NIR (0.25 W/cm2) for 5 min rapidly decreased with increasing Au-LTSL-GA.A concentration. This result suggested that treatment with Au-LTSL-GA.A and irradiation with NIR induced apoptosis in MCF-7. The bright cell images of cells treated with different concentrations (10, 20, and 30 µg/mL) of Au-LTSL-GA.A and irradiated with NIR (0.25 W/cm2) for 5 min are shown in . These images showed that the cells in the blank group were intact. However, cells treated with Au-LTSL-GA.A appeared apoptotic and the number of apoptotic cells increased with increasing concentration of Au-LTSL-GA.A. These results are consistent with the MTT assay results described above. To further investigate the antiproliferative effect of Au-LTSL-GA.A + NIR irradiation, RTCA was performed in this study (). Cells treated with GA.A, LTSL-GA.A, or Au-LTSL-GA.A was used as control groups. Adhesion was then monitored as a function of time by real-time cell electronic sensing (RT-CES). Analysis of cell adhesion revealed the extent of cell viability, and the results were broadly similar to those obtained by the MTT assay. These results also demonstrated that LTSL-GA.A had better antitumour activity than raw GA.A. Moreover, after liposomes were modified by Au NRs and irradiated with NIR (0.25 W/cm2), the anticancer activity was further improved. The mechanism responsible for the enhancement of the anticancer activity was to increase the intracellular release rate of GA.A
Figure 4. The anticancer activity of Au-LTSL-GA.A evaluated through MTT (A) and RTCA (B) assay for MCF-7 cells. Cells treated with NIR irradiation, GA.A (30 μg/mL), LTSL-GA.A (30 μg/mL), Au NRs + NIR irradiation (30 μg/mL) and Au-LTSL-GA.A (30 μg/mL) were set as control groups. Cells were also treated with of Au-LTSL-GA.A (30 µg/mL) for 2 h in incubation and measured for 5 min upon irradiation (0.25 W/cm2). (C) The bright cell images of each of the above groups.
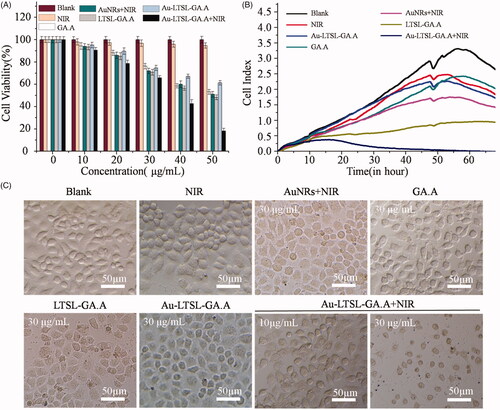
TABLE 2. IC50 (μg/mL) values of the GA.A, Au NRs + NIR irradiation, Au-LTSL-GA.A, and Au-LTSL-GA.A + NIR against cell lines.
Anticancer activity evaluation by a fluorescence assay
In order to more intuitively understand the process of apoptosis, the antitumour effect of the Au-LTSL-GA.A + NIR irradiation treatment on MCF-7 cells was evaluated using a LIVE/DEAD fluorescence assay. As shown in , all the cells in the blank group showed the green fluorescence of Calcein-AM, indicating that they were living cells. A number of cells in the groups treated with NIR irradiation, GA.A (30 μg/mL), Au NRs + NIR irradiation, or Au-LTSL-GA.A (30 μg/mL) showed the red fluorescence staining, which is indicative of dead cells. There were more apoptotic cells in the groups treated with Au NRs + NIR irradiation and Au-LTSL-GA.A compared with those in the group treated with raw GA.A and NIR irradiation. Instead, the groups that were treated with different concentrations of Au-LTSL-GA.A + NIR irradiation clearly showed a large number of apoptotic cells. The number of live cells gradually decreased with increasing concentration of Au-LTSL-GA.A. Indeed, at a concentration of 30 μg/mL, almost all cells in the field of view were apoptotic. These results confirmed that treatment with Au-LTSL-GA.A + NIR can effectively promote tumour cell apoptosis. Au-LTSL-GA.A + NIR irradiation exhibited high anticancer activity against MCF-7 cells combined phototherapy sensitization property of Au NRs with antitumour activity of GA.A.
Figure 5. Fluorescence microscopic images of MCF-7 cells incubated with different treatment and subsequent brief staining. The blank group was PBS. Cells treated with NIR irradiation, GA.A (30 μg/mL), Au NRs + NIR irradiation (30 μg/mL), Au-LTSL-GA.A (30 μg/mL) were set as control groups. Cells were treated with Au-LTSL-GA.A (30 μg/mL) for 2 h in incubation and measured for 5 min upon irradiation (0.25 W/cm2). Cells were stained (for 30 min) with Calcein-AM and PI for anticancer activity.
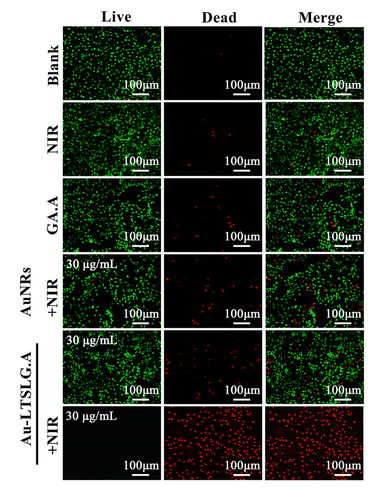
Apoptotic cell death analysis
Quantitative analysis of apoptotic cells was performed by flow cytometry analysis using the Annexin-FITC apoptosis detection kit in MCF-7 cells. The apoptosis ratio for the blank group, NIR irradiation group, and GA.A (30 μg/mL) group was 1.46, 5.68 and 9.59%, respectively. The Au NRs + NIR irradiation and Au-LTSL-GA.A (30 μg/mL) showed high anticancer activity with an apoptosis ratio of 23.53 and 36.87%. However, the group treated with Au-LTSL-GA.A (30 μg/mL) and measured for 5 min upon NIR irradiation (0.25 W/cm2) showed an apoptosis ratio of 66.86%. The enhanced apoptotic effect of Au-LTSL-GA.A on MCF-7 cells confirmed the effectiveness of the anticancer photochemotherapy (. Moreover, the flow cytometry analysis results showed that the Au-LTSL-GAA + NIR irradiation treatment induced cell apoptosis.
Figure 6. MCF-7 cells treated with Au-LTSL-GA.A (30 μg/mL) for 2 h in incubation and measured for 5 min upon irradiation (0.25 W/cm2), were analyzed using flow cytometry by Annexin V-FITC/PI staining after 12 h culture. Cells treated with NIR irradiation, GA.A (30 μg/mL), Au NRs + NIR irradiation (30 μg/mL) and Au-LTSL-GA.A (30 μg/mL) were set as control groups. The blank group was PBS.
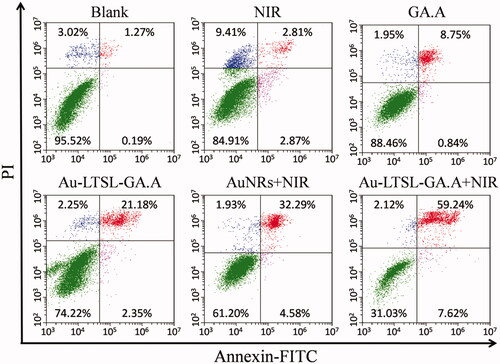
Discussion
In this paper, we defined the concentration used in the experiments based on the whole nanoparticles. The amounts of GA.A in GA.A alone, LTSL-GA.A and Au-LTSL-GA.A, are different. The content of GA.A in the same concentration of these materials is Au-LTSL-GA.A < LTSL-GA.A < GA.A due to the Au NRs loaded the LTSL. The same concentration of LTSL-GA.A and Au-LTSL-GA.A showed similar or better activity than raw GA.A in antibacterial and anticancer assays. However the GA.A was used in a small amount. These results suggested LTSL to encapsulate GA.A, which successfully transforms the polarity of GA.A and enhanced the activity of GA.A. We defined the concentration used in the experiments such as 50 μg/mL and didn’t violate the authenticity of the experiments. The experiments results are credible. The multifunctional Au-LTSL-GA.A, a self-assembled thermal nanomaterial, was used in antibacterial and anticancer applications in combination with near-infrared (NIR) irradiation. The Au NRs, LTSL and GA.A are also very important components for potential nanomedicine (Au-LTSL-GA.A).
The results of the antibacterial activity experiments suggested Au-LTSL-GA.A + NIR irradiation had a broad-spectrum antibacterial effect, which exerted a strong antibacterial activity against drug-resistant E. coli and S. aureus. The raw GA.A was modified to enhance its antibacterial activity. However, Au NRs irradiation exhibited poor antibacterial activity. The reason is that bacteria have high heat-resistance. Au-LTSL-GA.A and LTSL-GA.A were thermosensitive liposome and taken up by cells not externally heated, and it is not possible to release GA.A quickly. Therefore, the activity is low in a short period of time, and it is difficult to achieve the purpose of antibacterial effect. But the antibacterial effect of LTSL-GA.A is better than the raw, poorly polar GA.A. The content of GA.A is Au-LTSL-GA.A < GA.A due to the Au NRs loaded the LTSL. So the same concentrations of Au-LTSL-GA.A and GA.A showed a similar antibacterial activity. The MIC values for the group treated with Au-LTSL-GA.A + NIR irradiation were 9.3 ± 0.26 μg/mL for E. coli and 7.5 ± 0.62 μg/mL for S. aureus.
The mechanism of antibacterial mode of action of the Au-LTSL-GA.A + NIR treatment is still unclear and needs to be evaluated. Dramatic changes in bacterial cell morphology may be a major cause of cell death in the antibacterial assay. SEM and TEM are the main techniques to examine the morphological changes of cells. Several studies have proposed that the antibacterial mechanism of the drug involved the damage of the cell membrane and cell wall [Citation41,Citation42]. Therefore, this study initially determined the inhibitory mechanism by examining the changes in cell morphology after the treatment with Au-LTSL-GA.A + NIR irradiation.
SEM and TEM examinations were performed to observe the changes in the bacterial cell morphology. The destruction of the bacterial membrane was observed by SEM, as shown in . The drug-resistant E. coli and S. aureus without any treatment showed intact cell morphology. The enlarged region in the blank group indicated that E. coli and S. aureus cells had rod and spherical shape, respectively, with smooth and intact cell membranes. Instead, the control groups (GA.A, LTSL-GA.A, or Au-LTSL-GA.A) showed almost completely different cell morphology, less wrinkled cells, and less broken cells. The enlarged region in the control groups indicated that E. coli and S. aureus were rod and spherical shaped, respectively, with damaged membranes. The bacteria cells treated with Au-LTSL-GA.A + NIR irradiation had a large area of cell damage. The enlarged region in this group treated with Au-LTSL-GA.A + NIR irradiation showed cells with no defined morphology. These results suggested that the antibacterial activity of raw GA.A, LTSL-GA.A and Au-LTSL-GA.A was weak, whereas the antibacterial activity of Au-LTSL-GA.A + NIR was clearly strong, and its antibacterial effect was better. The loss of bacterial cell morphology induced by treatment with Au-LTSL-GA.A + NIR irradiation could be one of the mechanisms of cell death.
Figure 7. Morphological changes of drug-resistant E. coil (A) and S. aureus (B) through the SEM observed. Cells treated with Au-LTSL-GA.A (50 μg/mL) for 2 h incubation and measured for 5 min upon irradiation (0.25 W/cm2). Cells treated with GA.A (50 μg/mL), LTSL-GA.A (50 μg/mL), Au-LTSL-GA.A (50 μg/mL) were set as control groups. Bacteria without NPs were as blank. The sections in white circles will be enlarged.
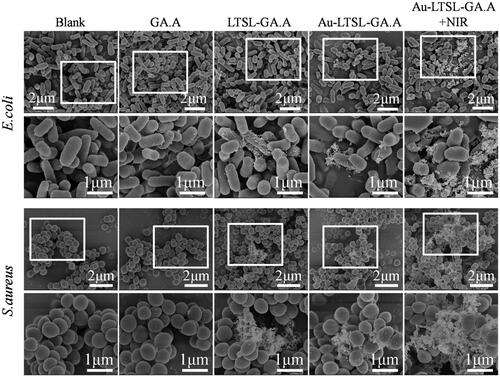
The Au-LTSL-GA.A nanoparticles could penetrate the bacterial cell membrane and wall, then taken up by the cell. We performed TEM analysis of bacterial sections (E. coli and S. aureus) and examined the distribution of Au-LTSL-GA.A nanoparticles inside the bacterial cell to further understand the underlying antibacterial mechanism. The images of ultrathin sections from the blank group shown in reveal that the cells have an intact morphology, the cell wall and cell membrane are not damaged, and the intracellular nucleic acid region is also intact. The cells that were treated with GA.A also exhibited a similar morphology as those from the blank group. In addition, the nanoparticles were observed in the images. In comparison, the cells that were treated with Au-LTSL-GA.A or Au-LTSL-GA.A + NIR irradiation exhibited different morphology from those in the blank and GA.A groups. The morphology of the cells was irregular, cells appeared broken and voids appeared inside the cells. However, the cell damage in the group treated with Au-LTSL-GA.A + NIR irradiation was more pronounced than that in the group treated with Au-LTSL-GA.A. Additionally, it was also found that in the group treated with Au-LTSL-GA.A + NIR irradiation, the number of damaged cells increased with the increase of the concentration of Au-LTSL-GA.A. Moreover, the images demonstrate the presence of Au NRs inside the cells, as indicated by the red arrow. These findings confirm that the Au-LTSL-GA.A can enter the bacterial cells and release GA.A where it exerts its bacteriostatic action. Loss of bacterial cell morphology induced by Au-LTSL-GA.A + NIR irradiation was evaluated by SEM and TEM examinations. The results suggested that treatment with Au-LTSL-GA.A + NIR irradiation led to the uptake of the nanoparticles by the cells, and once inside, the cells destroy the cell integrity. This should be one of the mechanisms of cell death.
Figure 8. Morphological changes of drug-resistant E. coil (A) and S. aureus (B) through TEM observation. Cells treated with GA.A (50 μg/mL), LTSL-GA.A (50 μg/mL), Au-LTSL-GA.A (50 μg/mL) were set as control groups. Cells were treated with different concentration of Au-LTSL-GA.A (30 and 50 μg/mL) for 2 h in incubation and measured for 5 min upon irradiation (0.25 W/cm2). Bacteria without NPs were as blank. The red arrows point to the NPs.
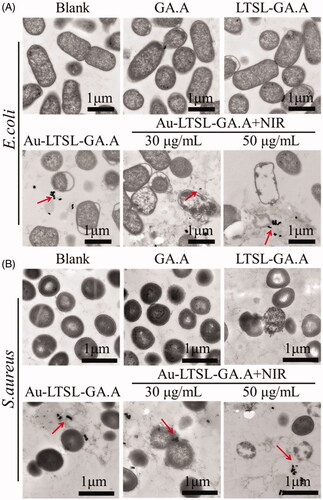
In the anticancer activity experiments, the Au-LTSL-GA.A + NIR irradiation treatment exhibited strong anticancer activity against MCF-7 cells. The IC50 value of the Au-LTSL-GA.A + NIR irradiation treatment (12.1 ± 1.3 μg/mL) was similar to that of cisplatin for MCF-7 cell. The results of the fluorescence assay confirmed that the Au-LTSL-GA.A + NIR irradiation treatment can effectively promote tumour apoptosis. Flow cytometry analysis also showed that the Au-LTSL-GA.A + NIR irradiation treatment induced cell apoptosis. The TEM images of MCF-7 cells demonstrated the presence of Au-LTSL-GA.A nanoparticles inside the cells, which confirmed the uptake of Au-LTSL-GA.A by MCF-7 cells and subsequent disruption of cancer cell morphology. The evaluation of the potential in vivo toxicity of NIR irradiation and Au-LTSL-GA.A showed no toxicity in mice.
Although the anticancer activity of the treatment with Au-LTSL-GA.A + NIR irradiation was shown by the in vitro experiments, they did not allow the visualization of cellular compartments at the nanoscale to demonstrate the uptake of the nanoparticles by tumour cells. TEM imaging can provide nanoscale resolution images of ultrathin sections of tumour cells. As shown in , the morphology of the cells in the blank group was intact and the internal structure was clear, indicating that they were viable functioning cells with no signs of apoptosis. The cells of the control group treated with GA.A showed similar intact morphology, which suggested the raw GA.A had weak anticancer activity against MCF-7 cells. However, the cells of the group treated with Au-LTSL-GA.A + NIR had a different morphology, some cells appeared impaired, while others were damaged, or disintegrated, and their cytoplasm leaked. Moreover, the Au-LTSL-GA.A nanoparticle could be found inside the cell, thus confirming the uptake of Au-LTSL-GA.A by MCF-7 cells.
Figure 9. TEM images of MCF-7 cells treated with Au-LTSL-GA.A were analyzed at a concentration of 30 µg/mL measured for 5 min upon irradiation (0.25 W/cm2). Red squares and red arrows indicate Au-LTSL-GA.A. Assay treated with PBS is the blank. The GA.A (30 µg/mL) and LTSL-GA.A (30 µg/mL) were set as the control group.
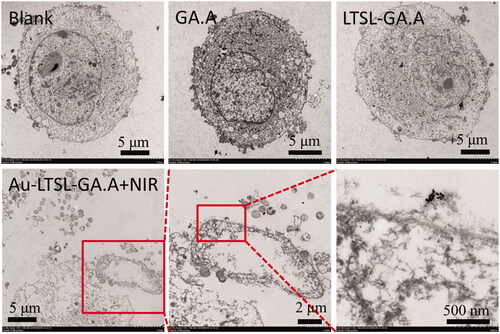
The potential in vivo toxicity is usually a significant concern for the clinical application of photochemotherapy drugs. To evaluate the toxicity of the Au-LTSL-GA.A + NIR irradiation treatment, blood biochemical and immunohistochemistry assays were performed to examine possible in vivo toxicity in mice after treatment. As shown in , the levels of cholesterol, blood glucose, uric acid, and alanine aminotransferase in the NIR irradiation and Au-LTSL-GA.A + NIR irradiation groups were similar to those found in the blank group. They all showed healthy blood biochemical indicators. This result was also confirmed by H&E staining of major organs. The blank, NIR irradiation and Au-LTSL-GA.A + NIR treatment groups showed similar histological features, with no obvious impairment or inflammation (). These results indicated the safety of the use of the Au-LTSL-GA.A + NIR treatment in mice.
Conclusion
In this study a multifunctional Au-LTSL-GA.A nanoparticle was synthesized and characterized. In the novel Au-LTSL-GA.A nanoparticles the thermosensitive liposome (LTSL) is mainly used to encapsulate GA.A, which successfully transforms the polarity of the GA.A and improves its water solubility. Through the modification of Au NRs and in combination with NIR irradiation, a self-assembled thermal nanomaterial with high activity was prepared. The designed Au-LTSL-GA.A nanoparticle is used as a nano-photosensitizer to achieve synergistic photochemotherapy based on the phototherapy sensitization property of Au nanorods (NRs) and antitumour activity of GA.A. Characterization experiments confirmed that the LTSL successfully encapsulated GA.A. The TEM analysis revealed that LTSL was monodispersed and varied in size from 200 to 300 nm. TEM examination of the Au-LTSL-GA.A revealed that the Au NRs were clearly attached to the surface of the liposome and the size was about 350 nm. The results of the antibacterial experiments suggested that the treatment with Au-LTSL-GA.A + NIR irradiation exhibited a broad-spectrum antibacterial effect, which exerted a strong antibacterial activity against drug-resistant E. coli and S. aureus. In the anticancer activity experiments, the Au-LTSL-GA.A + NIR irradiation treatment exhibited strong anticancer activity against MCF-7 cells. In addition, the evaluation of the potential in vivo toxicity of NIR irradiation and Au-LTSL-GA.A showed no toxicity in mice. Finally, the treatment with the newly prepared nanoparticles, Au-LTSL-GA.A in combination with NIR irradiation, exhibited excellent antibacterial (E. coli and S. aureus) and anticancer (MCF-7 cell) activities. Accordingly, the Au-LTSL-GA.A nanoparticles have a wide range of potential industrial and clinical applications as a new generation of powerful antibacterial and anticancer agents that can be successfully targeted to organs or tissues of interest.
Supplemental Material
Download ()Disclosure statement
The authors declare no competing financial interest.
Correction Statement
This article was originally published with errors, which have now been corrected in the online version. Please see Correction (http://dx.doi.org/10.1080/21691401.2023.2245267)
Additional information
Funding
References
- Paterson RR. Ganoderma - a therapeutic fungal biofactory. Phytochemistry. 2006;67:1985–2001.
- Xu JW, Zhao W, Zhong JJ. Biotechnological production and application of ganoderic acids. Appl Microbiol Biotechnol. 2010;87:457–466.
- Da J, Cheng CR, Yao S, et al. A reproducible analytical system based on the multi-component analysis of triterpene acids in Ganoderma lucidum. Phytochemistry. 2015;114:146–154.
- Zhao XR, Huo XK, Dong PP, et al. Inhibitory Effects of Highly Oxygenated Lanostane Derivatives from the Fungus Ganoderma lucidum on P-Glycoprotein and α-Glucosidase. J Nat Prod. 2015;78:1868–1876.
- Bishop KS, Kao CH, Xu Y, et al. From 2000 years of Ganoderma lucidum to recent developments in nutraceuticals. Phytochemistry 2015;114:56–65.
- Shi L, Ren A, Mu D, et al. Current progress in the study on biosynthesis and regulation of ganoderic acids. Appl Microbiol Biotechnol. 2010;88:1243–1251.
- Guo XY, Han J, Ye M, et al. Identification of major compounds in rat bile after oral administration of total triterpenoids of Ganoderma lucidum by high-performance liquid chromatography with electrospray ionization tandem mass spectrometry. J Pharmaceutical Biomed. 2012;63:29–39.
- Zhang DH, Jiang LX, Li N, et al. Overexpression of the squalene epoxidase gene alone and in combination with the 3-hydroxy-3-methylglutaryl coenzyme A gene increases ganoderic acid production in Ganoderma lingzhi. J Agric Food Chem. 2017;65:4683–4690.
- Zhao X, Luo G, Bai C, et al. Juglone thermosensitive liposomes: preparation, characterization, in vitro release and hyperthermia cell evaluation. Int J Food Eng. 2016;12:429–438.
- Kim HR, You DG, Park SJ, et al. MRI Monitoring of tumour-selective anticancer drug delivery with stable thermosensitive liposomes triggered by high-intensity focused ultrasound. Mol Pharmaceutics. 2016;13:1528–1539.
- Tagami T, Ernsting MJ, Li SD. Efficient tumour regression by a single and low dose treatment with a novel and enhanced formulation of thermosensitive liposomal doxorubicin. J Control Release. 2011;152:303–309.
- Allen TM, Cullis PR. Drug delivery systems: entering the mainstream. Science. 2004;303:1818–1822.
- Sun D, Li N, Zhang W, et al. Quercetin-loaded PLGA nanoparticles: a highly effective antibacterial agent in vitro and anti-infection application in vivo. J Nanopart Res. 2016;18:3.
- Hansen MB, Gaal EV, Minten I, et al. Constrained and UV-activatable cell-penetrating peptides for intracellular delivery of liposomes. J Control Release. 2012;164:87–94.
- Kono K, Ozawa T, Yoshida T, et al. Highly temperature-sensitive liposomes based on a thermosensitive block copolymer for tumour-specific chemotherapy. Biomaterials 2010;31:7096–7105.
- Yang Y, Yang Y, Xie X, et al. PEGylated liposomes with NGR ligand and heat-activable cell-penetrating peptide-doxorubicin conjugate for tumour-specific therapy. Biomaterials. 2014;35:4368–4381.
- Dromi S, Frenkel V, Luk A, et al. Pulsed-high intensity focused ultrasound and low temperature-sensitive liposomes for enhanced targeted drug delivery and antitumour effect. J Pharm Sci. 2007;13:2722–2727.
- Banno B, Ickenstein LM, Chiu GN, et al. The functional roles of poly(ethylene glycol)-lipid and lysolipid in the drug retention and release from lysolipid-containing thermosensitive liposomes in vitro and in vivo. J Pharm Sci. 2010;99:2295–2308.
- Kuai R, Yuan W, Qin Y, et al. Efficient delivery of payload into tumour cells in a controlled manner by TAT and thiolytic cleavable PEG co-modified liposomes. Mol Pharmaceutics. 2010;7:1816–1826.
- Tagami T, Foltz WD, Ernsting MJ, et al. MRI monitoring of intratumoural drug delivery and prediction of the therapeutic effect with a multifunctional thermosensitive liposome. Biomaterials 2011;32:6570–6578.
- Needham D, Anyarambhatla G, Kong G, et al. A new temperature-sensitive liposome for use with mild hyperthermia: characterization and testing in a human tumour xenograft model. Cancer Res. 2000;60:1197–1201.
- Koning GA, Eggermont AMM, Lindner LH, et al. Hyperthermia and Thermosensitive Liposomes for Improved Delivery of Chemotherapeutic Drugs to Solid Tumours. Pharm Res. 2010;27:1750–1754.
- Hossann M, Wang T, Wiggenhorn M, et al. Size of thermosensitive liposomes influences content release. J Control Release. 2010;147:436–443.
- Alahmady ZS, Aljamal WT, Bossche JV, et al. Lipid-peptide vesicle nanoscale hybrids for triggered drug release by mild hyperthermia in vitro and in vivo. Acs Nano. 2012;6:9335–9346.
- Lozano N, Al-Jamal WT, Taruttis A, et al. Liposome-gold nanorod hybrids for high-resolution visualization deep in tissues. J Am Chem Soc. 2012;134:13256–13258.
- Yang L, Tseng YT, Suo G, et al. Photothermal therapeutic response of cancer cells to aptamer–gold nanoparticle-hybridized graphene oxide under NIR illumination. Acs Appl Mater Interfaces. 2015;7:5097–5106.
- Pan L, Liu J, Shi J. Intranuclear photosensitizer delivery and photosensitization for enhanced photodynamic therapy with ultralow irradiance. Adv Funct Mater. 2014;24:7318–7327.
- Chen Z, Zhao P, Luo Z, et al. Cancer cell membrane-biomimetic nanoparticles for homologous-targeting dual-modal imaging and photothermal therapy. Acs Nano. 2016;10:10049–10057.
- Sun D, Li N, Zhang W, et al. Design of PLGA-functionalized quercetin nanoparticles for potential use in Alzheimer’s disease. Coll Surf B. 2016;148:116–129.
- Sun D, Zhang W, Mou Z, et al. Transcriptome analysis reveals silver nanoparticle-decorated quercetin antibacterial molecular mechanism. Acs Appl Mater Interfaces. 2017;9:10047–10060.
- Sun D, Zhang W, Li N, et al. Silver nanoparticles-quercetin conjugation to siRNA against drug-resistant Bacillus subtilis for effective gene silencing: in vitro and in vivo. Mat Sci Eng C-Mate. 2016;63:522–534.
- Velema WA, van der Berg JP, Szymanski W, et al. Orthogonal control of antibacterial activity with light. ACS Chem Biol. 2014;9:1969–1974.
- Gunputh UF, Le H, Handy RD, et al. Anodised TiO 2 nanotubes as a scaffold for antibacterial silver nanoparticles on titanium implant. Mat Sci Eng C-Mate. 2018;91:638–644.
- Choi I, Lee J, Kim W, et al. On-demand modulation of bacterial cell fates on multifunctional dynamic substrates. Acs Appl Mater Interfaces. 2018;10:4324–4332.
- Sun D, Zhang W, Lv M, et al. Antibacterial activity of ruthenium(II) polypyridyl complex manipulated by membrane permeability and cell morphology. Bioorg Med Chem Lett. 2015;25:2068–2073.
- Takao S, Sekizawa O, Samjeské G, et al. Observation of degradation of Pt and carbon support in polymer electrolyte fuel cell using combined nano-XAFS and TEM techniques. Acs Appl Mater Interfaces. 2018;10:27734–27744.
- Sun D, Mou Z, Li N, et al. Anti-tumour activity and mechanism of apoptosis of A549 induced by ruthenium complex. J Biol Inorg Chem. 2016;21:1–12.
- Yang X, Zhang W, Zhao Z, et al. Quercetin loading CdSe/ZnS nanoparticles as efficient antibacterial and anticancer materials. J Inorg Biochem. 2017;167:36–48.
- Yang ZY, Li H, Zeng YP, et al. Photosensitizer-loaded branched polyethylenimine-PEGylated ceria nanoparticles for imaging-guided synchronous photochemotherapy. Acs Appl Mater Interfaces. 2015;7:24218–24228.
- Andel EV, Bus ID, Tijhaar EJ, et al. Highly specific binding on antifouling zwitterionic polymer-coated microbeads as measured by flow cytometry. Acs Appl Mater Interfaces. 2017;9:38211–38221.
- Guo X, Liu J, Xiao B. Evaluation of the damage of cell wall and cell membrane for various extracellular polymeric substance extractions of activated sludge. J Biotechnol. 2014;188:130–135.
- Kong M, Chen XG, Liu CS, et al. Antibacterial mechanism of chitosan microspheres in a solid dispersing system against E. coli. Coll Surf B. 2008;65:197–202.