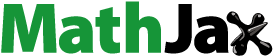
Abstract
Recently, yeast-derived glucan particles (GP) have emerged as novel drug delivery agents that provide for receptor-mediated uptake by phagocytic cells expressing β-glucan receptors. In our previous study, we prepared GP loaded with high payload (40.5 + 1.9%) of rifabutin (RB) nano-particles [(RB-NPs)-GP]. We investigated the anti-mycobacterial efficacy and cellular activation responses within Mycobacterium tuberculosis (M. tuberculosis) infected J774 macrophage cells following exposure to the (RB-NPs)-GP formulation. The exposure was seen to augment a robust innate immune response including the induction of reactive oxygen and nitrogen species, autophagy and apoptosis within M. tuberculosis infected macrophage. Further, the efficacy testing of these particles in murine macrophage exhibited that the (RB-NPs)-GP formulation enhanced the efficacy of RB drug by ∼2.5 fold. The study suggests that the set of innate responses conducive to killing intracellular bacteria evoked by (RB-NPs)-GP play a pivotal role in impeding the intracellular M. tuberculosis survival, resulting in enhanced efficacy of the formulation. Our results establish that the (RB-NPs)-GP formulation not only activate M. tuberculosis infected, immune-suppressed macrophage, but also adds significantly to the efficacy of loaded drug, and thus forms a promising approach that should be explored further as an alternative or adjunct form of TB therapy.
Nano-Rifabutin loaded Glucan microparticles [(RB-NPs)-GP] administered to M. tuberculosis infected macrophage.
(RB-NPs)-GP induces appropriate innate immune responses in host macrophage.
Mycobactericidal Effect of Rifabutin was markedly enhanced by its nano-entrapment in GP.
Intracellular drug delivery supplements the innate response in M. tuberculosis infected macrophage.
Highlights
Introduction
Tuberculosis (TB) is a deadly disease caused by the facultative intracellular pathogen Mycobacterium tuberculosis (M. tuberculosis), which remains a major cause of morbidity and mortality worldwide. Despite intense efforts to mitigate its toll on humanity, <10 million new cases and 2 million deaths have been reported in 2016 [Citation1]. In recent decades, very few anti-TB drugs have been approved for human use including the newest drugs, bedaquiline and delamanid that may be used for TDR-TB [Citation2], both of which have been reported for resistance development [Citation3]. Further, the emergence of multiple- and extensive- drug-resistant TB and co-infection with HIV highlight the necessity of novel anti-TB therapeutics in accordance with our understanding of pathogen and host macrophage biology.
Macrophages are known to phagocytose mycobacteria during the early infection stages. However, M. tuberculosis has evolved a spectrum of strategies to survive and replicate inside the macrophages [Citation4]. Upon internalization by macrophages, M. tuberculosis is enclosed within phagosome, but escapes fusion with the acidic late endosomes and lysosomes, M. tuberculosis arrests phagosomal maturation [Citation5], thus preventing acidification by vesicular proton pumps and lysosomal fusion [Citation6,Citation7] and creating a niche for its survival and replication. The intracellular mycobacterium subverts innate immune responses of the macrophage, inclining the phagocyte to an “alternatively activated state” [Citation8].
Drug delivery systems are potentially capable of affecting activation status of the cells they target. Several reports have shown that the phagocytic uptake of particulate matter is often linked to macrophage activation [Citation9]. Our previous studies have shown that phagocytosis of PLA-microparticles acts as an activation signal and reverses state of alternative activation to classical, mycobactericidal activation [Citation10,Citation11]. Lately, β-Glucan particles (GP) extracted from the cell walls of baker’s yeast are porous, 1–4 μm spherical shells composed primarily of β-1,3/1,6-D-glucan. The hollow cavity of these particles allow adsorption and encapsulation of payload molecules, and the β-1,3 D-glucan moiety on surface permits their recognition by dectin-1 receptors on macrophage [Citation12]. Recently, glucan particle-based delivery systems loaded with anti-tuberculosis drugs have been formulated [Citation13,Citation14]. In this context, we prepared GP containing a payload of nano-embedded rifabutin (RB), an anti-TB drug reported to be effective against MDR-TB [Citation14]. We were able to achieve high RB payload within the polymer matrix, as “Nano-in-Micro” particulate formulation, with increase in drug content up to 40.5% (w/w) RB by spray-drying. However, the efficacy of these GP based systems [Citation13,Citation15], has not been underscored to date in terms of the contribution of delivery system towards the activation status of macrophage. The study, therefore, further explored whether the administration of these particles induce a set of appropriate innate bactericidal events within M. tuberculosis infected macrophage and assessed the anti-mycobacterial efficacy of nano-RB encapsulated GP (RB-NPs)-GP formulation.
Materials and methods
Glucan particles and reagents
Beta-glucan particles (GP) of 1–4 µm diameters were prepared from budding yeast (Saccharomyces cerevisiae) by alkaline and acidic extraction method, as reported earlier [Citation14]. Briefly, 200 g dry yeast (Polo Enterprises, Lucknow, India) was subjected to hot alkali extraction by heating for 1 h at 80°C in 1 M NaOH, to lyse yeast cells and remove mannosylated proteins and lipids trapped within the cell wall. After washing with distilled water, the insoluble sediment was washed with TDW, pH adjusted to 4–5 with HCl and incubated at 55°C for 1 h to remove chitin residues. Thereafter, the yeast wall slurry was washed with triple distilled water (3 times), isopropanol (4 times) and acetone (2 times), and finally spray dried to yield dry beta-l,3/1,6-glucan particles (GP). The structural analysis of these particles by FT-IR and 13 C NMR also verified the primary β-1,3/1,6-glucan structure [Citation14].
Further, nano-RB encapsulated GP [(RB-NPs)-GP] were prepared by incubating dry glucan particles in 0.2 N HCl with stock RB solution (100 mg RB per ml in 0.2 N HCl), and adding Tris buffer (1 M, pH 8) to precipitate and trap drug RB within GP [Citation14]. The RP-HPLC analysis revealed 40.5 ± 1.9% drug in these particles, indicating a loading ∼405 µg of RB per mg (RB-NPs)-GP formulation]. Particle size distribution was determined by a laser-based particle size analyzer (Mastersizer 2000, Malvern Instruments, UK) at the Pharmaceutics Division, Central Drug Research Institute, Lucknow.
DCFH-DA, Tris-EDTA, culture media and supplements were obtained from Sigma. 4,6-diamidino-2-phenylindole dihydrochloride (DAPI) and acridine orange (AO) were obtained from Himedia.
Cell culture and in vitro infection
J774A.1 mouse Macrophage cell line was purchased from the National Center for Cell Science (NCCS, Pune) and was maintained in DMEM with 10% FCS (complete medium).
M. tuberculosis strain H37Ra was grown to log phase in Sauton’s medium. Prior to infection, the culture was suspended in antibiotic-free DMEM and sonicated by Probe Sonicator (Ultrasonicator) using three pulses, each of 10 s at 10% amplitude to disrupt any bacterial aggregates and obtain a uniform suspension. The bacterial suspension was spun at 400×g for 5 min to remove clumps, of which about 1.5 ml suspension was taken and O.D. was read spectrophotometrically at 600 nm. Adherent J774.1 cells seeded at density of 0.1 × 106 cells/well in 96-well plates were exposed to a multiplicity of infection (MOI) of 10 for 3 h, in humidified CO2 incubator at 37°C and 5% CO2, while a control group received no infection (Group N). The extracellular bacilli were removed by removing the supernatant and washing the adhered cells with HBSS, following which, cells were exposed to (RB-NPs)-GP.
Reactive oxygen species (ROS) generation
The oxidative burst induced by uptake of (RB-NPs)-GP was evaluated by fluorescence imaging and quantitative fluorometric measurements following incubation with a cell-permeable fluorogenic dye 2′,7' –dichlorofluorescein diacetate (DCFH-DA), [Citation16,Citation17]. Adherent J774 cells (1 × 105 per well) infected with M. tuberculosis H37Ra were exposed to 10 µg/ml (RB-NPs)-GP for 24 h. After exposure, cells were incubated with 25 µM DCFH-DA for 30 min at 37°C. The plate was kept on a shaker for 10 min at room temperature in the dark. Fluorescence intensity was measured through fluorescent multi-well microplate reader (Synergy HT BIO-TEK) set at excitation wavelength of 485 nm and emission wavelength of 530 nm.
Measurement of nitric oxide (NO)
Adherent J774 cells infected with M. tuberculosis H37Ra and exposed to 10 µg/ml (RB-NPs)-GP for 24 h were assessed for dissolved nitrite production in culture supernatants using Griess reagent and incubated for 30 min at room temperature in the dark. Accumulated NO2−, a stable end product indicator of NO production in the cell supernatant reacts with Griess reagent to form a purple azo dye and was measured at 540 nm by microplate reader (VersaMax, Molecular Devices) [Citation18,Citation19].
Apoptosis detection
Changes in nuclear morphology
The changes in nuclear morphology induced by 10 µg/ml RB-NPs-GP were analyzed using nuclear fluorescent dye 4′, 6-diamidino-2-phenylindole (DAPI) following a slightly modified protocol [Citation20].
M. tuberculosis infected cells seeded at a density of 0.2 × 106 cells per well in 6-well plates containing polylysine-coated slide, were exposed to 10 µg/ml of RB-NPs-GP for 24 h. Cells were then washed with PBS and fixed in 4% paraformaldehyde for 10 min at room temperature. Subsequently the cells were subjected to permeabilizing buffer (4% paraformaldehyde and 0.5% Triton X-100 in PBS) and incubated with 0.5 µg/ml fluorescent nuclear dye 4',6-diamidino-2-phenylindole dihydrochloride (DAPI) for 5 min [Citation20,Citation21]. After staining, the cells were viewed under fluorescence microscope at 20X magnification.
Measurement of cell death
Propidium iodide (PI) was used to quantify cell death using flow cytometry and fluorescence microscopy. J774A.1 cells were seeded into 6-well plates at a density of 1.6 × 106 cells/well, infected with M. tuberculosis as described previously, and thereafter, incubated with 10 μg and 100 μg/ml of (RB-NPs)-GP for 24 h in 5% CO2 and at 37°C [Citation22]. Subsequently, the cells were washed with 1 × PBS (cold) and then fixed in cold methanol at −20°C for 2 h. Fixed cells were treated with RNase (20 µg/ml) and stained with PI in the dark for 30 min at room temperature. Thereafter, the PI fluorescence of individual nuclei was measured by using flow cytometer (BD FACS Caliber, Becton Dickinson, USA). Data were analyzed through the Cell Quest Pro V 3.2.1 software (Becton Dickinson, USA).
Detection of phagosomal acidification
M. tuberculosis H37Ra infected J774A.1 cells were exposed to GP and RB loaded GP for 24 h and then incubated with 1 μg/ml Acridine Orange (AO) for 15 min to detect acidic vesicular organelles (AVOs) and were visualized after illumination with blue light (488 nm). In AO stained cells, the acidic compartments were seen to fluorescence bright red, the intensity of the fluorescence being proportional to the degree of acidity. The nucleolus and cytoplasm emitted green fluorescence (520 nm) upon excitation with the same wavelength of light. Photomicrographs were obtained with an upright fluorescent microscope [Citation23] at 20X magnification.
The GP exposed cells were also stained with LysoTracker-G dye to detect lysosomal compartments and phagosomal acidification upon lysosome fusion were monitored. After GP exposure for 30 min, 4 h and 24 h cells were washed with PBS and stained with LysoTracker-G (50 nM). After 15 min incubation at 37°C cells were washed with PBS and visualized under fluorescent microscope.
Intracellular anti-mycobacterial efficacy
J774 cells seeded at 1 × 106 cells per well in 12-well tissue culture plates, were infected with M. tuberculosis H37Ra at a MOI of 10:1 (bacteria-to-cell ratio) at 37°C in 5% CO2. Four hours after infection, the infected cells were exposed to an equivalent concentration (10 μg/ml) of blank GP, soluble (pure) RB or (RB-NP)-GP formulation. Thereafter, at 0 h, 48 h and 72 h post exposure, the macrophages were lysed in sterile water containing 0.025% (w/v) Triton X-100. The lysates were serially diluted (10X and 100X), of which 10 μl was plated on Middlebrooks 7H10 agar plates containing oleic acid, dextrose and catalase (OADC) supplements and cycloheximide. The plates were incubated at 37°C for 3 weeks under controlled humidity conditions, after which the colony forming units (CFU) were counted using the formula as given below, so as to determine the intracellular M. tuberculosis survival.
Statistical analysis
The results were represented as the mean ± S.D of three independent experiments which were performed in triplicates. Statistical analysis was performed by one-way ANOVA using Dunnett’s multiple comparison test and also by two-tailed, paired Student’s t-test (*p < 0.01,**p < 0.001, ***p < 0.0001 represent significant difference compared with control).
Results
(RB-NPs)-GP trigger ROS and NO induction within M. tuberculosis infected macrophage
Blank GP as well as (RB-NPs)-GP particles were seen to be rapidly taken up by mouse macrophage phagocytes within 5 min of exposure ().
Figure 1. Phagocytosis of (RB-NPs)-GP by M. tuberculosis infected J774 cells. Representative images of (A) untreated M. tuberculosis infected J774 cells, (B) M. tuberculosis infected cells exposed to 10 μg/ml blank GP and (C) to 10 μg/ml (RB-NPs)-GP, with magnified view of respective regions within yellow panels.
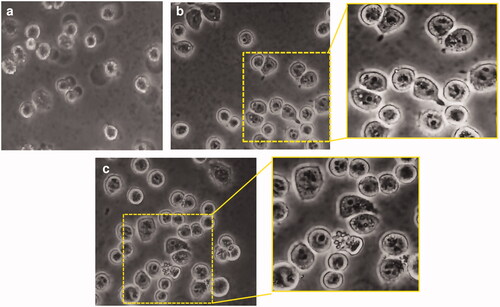
ROS generation was assessed as a consequence of particle uptake, in terms of relative fluorescence units using 10 mM DCFH-DA staining. Significantly high intracellular ROS generation was observed within M. tuberculosis infected cells, after 24 h (p < 0.0001, p < 0.0001) exposure to 10 µg/ml of both blank GP as well as (RB-NPs)-GP (p < 0.0001, p < 0.0001) (). The dose was chosen based on our previous results indicating that 10 μg/ml is the optimal dose that did not show any significant cytotoxicity after 24 h exposure of these particles to mouse macrophage [Citation14,Citation24]. The (RB-NPs)-GP particles were seen to induce significant NO production (p < 0.05) at the 24 h post-exposure time point within M. tuberculosis H37Ra infected macrophage ().
(RB-NPs)-GP trigger acidic vesicular organelles (AVOs) formation and accumulation of lysosomes within M. tuberculosis infected J774 cells
Next, we monitored the formation of acidic vesicular organelles (AVOs) by AO staining within M. tuberculosis infected macrophage, at 24 h post-exposure to (RB-NPs)-GP. Bright red coloured, fluorescent AVOs were readily detected within M. tuberculosis infected mouse macrophage upon exposure to both blank GP and (RB-NPs)-GP ().
Figure 3. Acidic vesicular organelles (AVOs) and Lysosomal activity. (A) Microscopic detection of AVOs. Acridine orange stains nucleus and cytoplasm as green and AVOs as bright red. (a) M. tuberculosis H37Ra infected, untreated control cells (I-CTRL). (b) M. tuberculosis infected cells exposed to 10 μg/ml of blank GP for 24 h, or (c) to (RB-NPs)-GP. (B) Quantitative estimation of lysosomal activity within LysoTracker-G preloaded, M. tuberculosis infected macrophage after 24 h exposure to (RB-NPs)-GP.
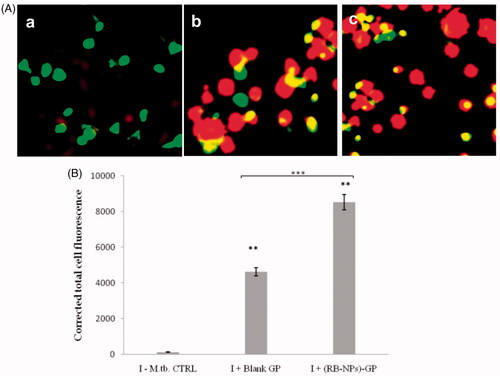
Thereafter, M. tuberculosis infected macrophage exposed to (RB-NPs)-GP for 24 h were stained with an acidic lysosomal tracking dye, LysoTracker-G. The infected cells exhibited a conspicuous increase in green fluorescence intensity (p < 0.05) after (RB-NPs)-GP particle exposure, indicating the activation of lysosome accumulation and phagolysosomal maturation (). The (RB-NPs)-GP particles were seen to induce significantly higher accumulation of lysosomes (p < 0.001) as compared to that by an equivalent weight of blank glucan particles.
(RB-NPs)-GP exposure induces cell death within M. tuberculosis infected macrophages
GP exposure to infected cells caused changes in nuclear morphology such as nuclear fragmentation with abnormal margins and condensed chromatin, visualized as deep blue fluorescence using DAPI staining. The administration of 10 µg/ml (RB-NPs)-GP particles to M. tuberculosis infected cells was found to induce apoptosis as visualized by separated nuclei composed by clusters of blue dots, indicating nuclear fragmentation. The study revealed DNA fragmentation and chromatin condensation and protrusions from the nucleus (nuclear blebs), indicating apoptosis induction upon exposure to (RB-NPs)-GP ().
Figure 4. Cell death induction. (A) Analysis of changes in nuclear morphology. Photomicrographs showing nuclear morphology of J774.1 cells upon DAPI staining of (a) uninfected, untreated control cells, (b) after 24 h exposure of M. tuberculosis infected cells to 10 µg/ml and (c) 100 µg/ml of (RB-NPs)-GP. (RB-NPs)-GP treated cells showed nuclear disintegration and blebbing as indicated by arrows. (B) Quantitative analysis of cell death induction by (RB-NPs)-GP. Flow cytometric data on % apoptotic cells obtained upon PI staining after exposure of M. tuberculosis infected macrophage with 10 µg/ml of blank GP, soluble RB and (RB-NPs)-GP. (***p < 0.0001 as compared to Uninfected, untreated and infected, untreated controls).
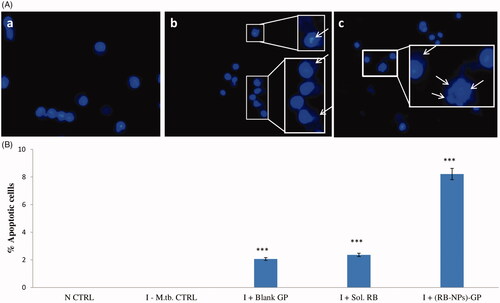
The induction of cell death upon (RB-NPs)-GP exposure to M. tuberculosis infected macrophage was also detected by PI staining followed by flow cytometry mediated quantitative analysis (). The study revealed an increase in the fragmentation and strand breaks of DNA (Sub Go) of M. tuberculosis infected cells by (RB-NPs)-GP, as the percentage of apoptotic cells, significantly increased from negligible (in uninfected, or infected untreated cells) to 8.21% by 10 µg/ml exposure and 38.96 % at 100 µg/ml exposure of (RB-NPs)-GP. Slight cell death was also observed to be induced within the murine macrophage cell line upon exposure to 10 µg/ml concentration of blank GP as well as soluble RB.
(RB-NPs)-GP significantly augment RB efficacy in restricting intracellular M. tuberculosis
While the infected, unexposed cells showed progressive intracellular M. tuberculosis growth () by 48 h (non-significant) and by 72 h (p < 0.05), the blank GP exerted a modest bactericidal activity at both the time points, reducing the CFU counts by 5.2 ± 1.8% (94.7% survival; p < 0.05) by 48 h, and by 10.5 ± 1.0% (89.48% survival; p < 0.05) by 72 h of exposure. The administration of free RB caused 1 log reduction in CFU/ml (20.6 ± 0.57% reduction in CFU; p < 0.01) over 2 days, and 1.45 log reduction in CFU/ml (26.75 + 0.8% reduction in CFU counts; p < 0.01) after 3 days incubation.
Figure 5. Intracellular anti-mycobacterial efficacy of (RB-NPs)-GP. Intracellular CFU counts upon exposure of M. tuberculosis infected cells to 10 µg/ml (RB-NPs)-GP for A. 48 h (grey bars) and B. 72 h (black bars) and comparing it with that at 0 h exposure time point (white bars), and with cells exposed to equivalent concentrations of blank GP and pure, soluble RB. M.tb. CTRL indicates control, untreated cells. C. Growth curve of surviving M. tuberculosis in J774 macrophage exposed to 10 µg/ml of (RB-NPs)-GP, or blank GP or free RB up to 72 h post-infection. (*p < 0.05; **< 0.001; ***< 0.0001 as compared to untreated cells, Student’s t-test).
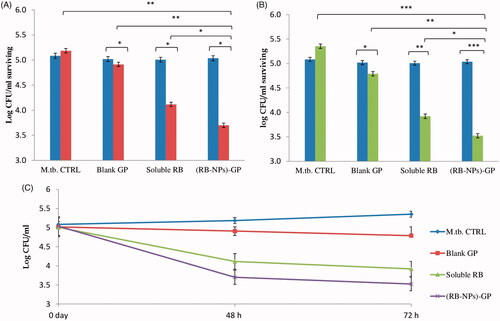
In contrast, (RB-NPs)-GP exerted much higher bactericidal activity (p < 0.05) as compared to both free RB and blank GP. The administration of 10 μg/ml (RB-NPs)-GP, led to 1.5 log reduction in CFU counts (amounting to 28.65 ± 1.9% reduction; p < 0.05) by 48 h, and a 2 log reduction in CFU (65.8 ± 1.2% intracellular survival, or 34.18% reduction; p < 0.001) at the 72 h post-exposure time point.
Discussion
Early induction of innate immunity to M. tuberculosis infection within host cells plays an important role in restricting the intracellular survival and proliferation of mycobacteria [Citation25,Citation26] and therefore, has been reported to be critical for determining disease outcome [Citation27]. The present study explored whether the administration of the Nano-Rifabutin loaded Glucan Particles (RB-NPs)-GP, prepared in our previous study [Citation14] would induce sufficient immunity so as to drive the immunosuppressed macrophage towards the development of an appropriate innate immune response within the phagocytes.
Microscopic examination of macrophages exposed to (RB-NPs)-GP revealed the presence of internalized particles within macrophages (). Phagocytosis of particles has been construed as an activation signal, depending on particle size, surface properties and chemical composition of the particles [Citation9,Citation28,Citation29]. The uptake of these particles by uninfected and infected macrophage was followed by rapid induction of ROS upon exposure (). Significantly high levels of ROS were generated in uninfected (p < 0.0001) and infected cells (p < 0.0001) at 24 h of exposure of (RB-NPs)-GP. Particle exposure was also seen to induce high amounts of NO (p < 0.05) within M. tuberculosis infected cells (), indicating macrophage activation by the exposure of (RB-NPs)-GP formulation. Several investigators have correlated induction of NO production with the control of mycobacterial infection [Citation30] and macrophage activation [Citation31]. Activated macrophages have been shown to inhibit M. tuberculosis growth and kill at least 50% of intracellular mycobacteria via NOS2-dependent mechanisms [Citation32]. High concentrations of NO are also reported to reversibly inhibit aerobic respiration and thus, control bacterial growth [Citation33].
NADPH oxidase activation upon phagocytosis and respiratory burst transfer electrons to O2, producing superoxide (O2-) in the phagosome, which forms hydrogen peroxide (H2O2) and reacts with it, generating different bactericidal ROS species [Citation34]. Mice deficient in Phox have been shown to be partially inhibited in their capability to suppress the growth of M. tuberculosis, before the onset of specific immunity [Citation35], suggesting the role for ROS in M. tuberculosis control, during early infection. On the other hand, iNOS deficient mice are reported to be highly susceptible to M. tuberculosis infection [Citation36]. Thus, the significant induction of ROS and NO, upon exposure to RB-NPs-GP particles is promising, since it indicates the activation of infected macrophage by these particles. While virulent M. tuberculosis has mechanisms to scavenge ROS produced by host macrophage [Citation33], the sustained induction of ROS and NO within infected macrophage could be detrimental to bacterial survival.
M. tuberculosis is known to avoid its killing by manipulating and arresting phagosome maturation. (RB-NPs)-GP uptake was seen to induce lysosome accumulation and AVO formation within M. tuberculosis infected macrophage (). While the blank GP administration induced vigorous lysosomal activity after 24 h exposure within M. tuberculosis infected cells, RB incorporation in GP was found to significantly increase the lysosomal activity as compared to blank GP (p < 0.0001), indicating enhanced phagosomal maturation by (RB-NPs)-GP (). The increased detection of AVOs viz. autophagic vacuoles and lysosomes indicates enhanced phagosomal acidification within M. tuberculosis infected cells upon exposure to (RB-NPs)-GP. The drop in pH of vesicular organelles from neutral (∼6.4, visualized as green) to acidic (red colour), and accumulation of lysosomes after 24 h exposure to (RB-NPs)-GP suggests the recruitment of vacuolar H+-ATPases on phagosome membrane, and progression of maturation arrested early phagosomes to late endosome or phagolysosome (PL) stage [Citation37,Citation38]. The fusion of autophagosomes with lysosomes to form auto-phagolysosomes as acidic vesicular organelles (AVOs) is one of the characteristic features of autophagy, an important immune effector that overcomes mycobacterial phagosome maturation block, allows phagosome-lysosome fusion and inhibits intracellular survival of M. tuberculosis [Citation39–41]. This study suggested that (RB-NPs)-GP might activate antimicrobial autophagy within M. tuberculosis infected macrophage. This observation shall be explored further, and experiments are planned to be conducted in this direction. Nevertheless, it is encouraging to observe the appearance of AVOs indicating phagosomal maturation along with other features of classical activation in infected macrophages upon exposure with these particles.
The induction of apoptosis within M. tuberculosis infected macrophage is also expected to provide an effective protection against the M. tuberculosis bacilli, known to survive and replicate inside the macrophage [Citation42–44]. The fragmented and condensed nuclei observed by DAPI and PI staining () indicated significant induction of apoptosis [Citation45] by (RB-NPs)-GP particles within M. tuberculosis infected macrophage after 24 h exposure. PI staining allowed the detection of sub-G0 (<2N) population of cells, indicating the occurrence of DNA fragmentation. Significant DNA fragmentation and strand break formation was revealed in M. tuberculosis infected cells after 24 h exposure to (RB-NPs)-GP. The particles were found to induce apoptosis in a caspase 3 dependent manner (data not shown). On the other hand, both blank GP as well as pure, soluble RB (10 µg/ml) were seen to induce non-significant induction of apoptosis in a few infected cells. Slight cell death induction by 10 µg/ml blank GP seems to be synergistic with that by soluble RB, leading to highly significant induction of cell death by (RB-NPs)-GP.
The (RB-NPs)-GP formulation showing various indicators of appropriate immune responses were then tested for in vitro anti-mycobacterial efficacy. The administration of 10 µg/ml RB-(NPs)-GP formulation was seen to meticulously restrict intracellular M. tuberculosis growth within 48 h (p < 0.001) and 72 h (p < 0.0001) of exposure, leading to significantly higher CFU reduction (p < 0.05) than that by an equivalent concentration (10 μg/ml) of free RB. It should also be noted that since the (RB-NPs)-GP contain 40.5% drug (and ∼59.5% polymer), the drug present in 10 μg/ml (RB-NPs)-GP suspension is merely 4.05 μg/ml (14). Thus, the (RB-NPs)-GP formulation containing RB content of only 4.05 μg/ml was found to be significantly more effective (p < 0.05; p < 0.05) against the intracellular, replicating bacteria than soluble RB at more than two times higher concentration (10 μg/ml). It is also worth appreciating that exposure to an equivalent weight of blank GP (10 μg/ml) also resulted in a significant reduction in CFU counts (p < 0.05) within 48 h. Thus, our findings clearly demonstrate a significant enhancement of anti-mycobacterial efficacy of RB, upon its entrapment within GP as (RB-NPs)-GP. These results establish a critical role of GP encapsulation in potentiating the therapeutic efficacy of RB in reducing intracellular M. tuberculosis survival or killing of M. tuberculosis In another similar study blank PLGA microparticles were seen to induce significant M. tuberculosis killing via autophagy and NF-kB mediated mechanism [Citation46].
The enhancement of antimycobacterial efficacy by particulate formulations is in accordance with previous reports such as for RIF loaded GP [Citation13] or PLA microparticles [Citation47]. β-(1,3)-D-glucans are polysaccharides, recognized as a biological response modifier [Citation48–50]. A comparison between nano-RB encapsulated GP [(RB-NPs)-GP] and RIF loaded GP formulations for M. tuberculosis survival in cell lysates after 72 h exposure, exhibited 98.7% reduction in CFU count by (RB-NPs)-GP formulation, as compared to 80–90% reduction by RIF loaded GP formulations [Citation13]. The higher therapeutic efficacy of (RB-NPs)-GP can be attributed to the higher drug loading (40.5 ± 1.9%) obtained by spray drying as compared to 10%–30% loading in RIF loaded GP [Citation13], This is also reflected by the drastically high drug content/glucan particle (∼34.3 pg RB per particle (14) as compared to 0.2 pg RIF per particle in GP formulations. The high drug loading per glucan particle implies that a low number of (RB-NPs)-GP particles would deliver higher drug amounts within the macrophage. Of note, in contrast to previous report [Citation13], the decreasing trends in CFU counts as observed from 0 h to 72 h by our formulation, indicate that the intracellular sustained drug release from these particles is sufficiently high (above the MIC value, at least up to 72 h). Such particles with high drug loading and sustained release are expected to maintain intracellular drug concentrations within the therapeutic window and thus promote mycobacterial killing over a long time period, thereby displaying a potential to address the noncompliance issues leading to drug resistance.
This study is in agreement with other previous studies demonstrating the induction of macrophage activation and anti-microbial signalling within phagocytic cells, in response to administration of drug-loaded microparticles [Citation10,Citation51,Citation52]. The recognition of (1 → 3)(1 → 6)-β-D-glucan by PRRs (Dectin-1, CR3 and TLR2/TLR6) on the phagocyte surface is known to trigger downstream signals, including induction of pro-inflammatory cytokines [Citation53]. This study led to the following major findings. Firstly, the enhanced activation of a spectrum of appropriate bactericidal, innate responses by these particles within infected macrophage, and secondly, a meticulous decline in intracellular M. tuberculosis survival indicating a significant improvement in the mycobactericidal efficacy of RB drug. This can be expected if the intracellular release of drug NPs from this formulation maintains intracellular drug concentrations within the therapeutic window for prolonged periods, resulting in enhanced killing of intracellular, replicating M. tuberculosis within macrophage. Thus it appears that besides their role as drug delivery system, the RB-loaded GP formulation confers additional advantage in the context of TB therapy, activating the crucial regulators of bactericidal responses within M. tuberculosis containing macrophage and thereby resulting in enhanced protection against M. tuberculosis.
Conclusions
We conclude that the mycobactericidal activity of drug RB is markedly and significantly enhanced upon its nano-entrapment within glucan particles as the (RB-NPs)-GP formulation, while the blank GP exhibited a moderate mycobactericidal activity as compared to control, untreated cells. Our results reveal a critical role of this nano-encapsulation in not only reducing the dose of the loaded anti-TB drug but also in promoting the establishment of an appropriate immune response and thereby, markedly further increasing its M. tuberculosis killing efficiency. These findings, therefore, surmise that targeting macrophage through (RB-NPs)-GP might be an effective immuno-therapeutic approach in evoking host immunity and restricting M. tuberculosis growth.
Acknowledgements
The authors like to gratefully acknowledge Dr. Amit Misra and Dr. K.K. Srivastava, Council of Science and Industrial Research-Central Drug Research Institute (CSIR-CDRI), Lucknow for providing access to highly sophisticated instruments and for access to Mycobacterial Culture Laboratory. Authors are thankful to DST for infrastructural support to the Department of Bio-sciences, Integral University under FIST program. We also acknowledge Integral University, Lucknow for providing the facilities to carry out this study. The manuscript communication number (MCN) of this article is IU/R&D/2018-MCN000369 as provided by the Research & Development Office, Integral University, Lucknow.
Disclosure statement
No potential conflict of interest was reported by the authors.
Additional information
Funding
References
- World Health Organization Global Tuberculosis Control Report. 2017. Available from: https://www.who.int/gho/tb/epidemic/cases_deaths/en/
- D'Ambrosio L, Centis R, Tiberi S, et al. Delamanid and bedaquiline to treat multidrug-resistant and extensively drug-resistant tuberculosis in children: a systematic review. J Thorac Dis. 2017;9:2093–2101.
- Hoffmann H, Kohl TA, Hofmann-Thiel S, et al. Delamanid and bedaquiline resistance in Mycobacterium tuberculosis ancestral Beijing genotype causing extensively drug-resistant tuberculosis in a Tibetan refugee. Am J Respir Crit Care Med. 2016;193:337–340.
- Ehrt S, Schnappinger D. Mycobacterial survival strategies in the phagosome: defense against host stresses. Cell Microbiol. 2009;11:1170–1178.
- Flannagan RS, Cosio G, Grinstein S. Antimicrobial mechanisms of phagocytes and bacterial evasion strategies. Nat Rev Microbiol. 2009;7:355–366.
- Sturgill-Koszycki S, Schlesinger PH, Chakraborty P, et al. Lack of acidification in Mycobacterium phagosomes produced by exclusion of the vesicular proton-ATPase. Science. 1994;263:678–681.
- Hestvik ALK, Hmama Z, Av-Gay Y. Mycobacterial manipulation of the host cell. FEMS Microbiol Rev. 2005;29:1041–1050.
- Kahnert A, Seiler P, Stein M, et al. Alternative activation deprives macrophages of a coordinated defense program to Mycobacterium tuberculosis. Eur J Immunol. 2006;36:631–647.
- Prior S, Gander B, Blarer N, et al. In vitro phagocytosis and monocyte–macrophage activation with poly (lactide) and poly (lactide-co-glycolide) microspheres. Eur J Pharm Sci. 2002;15:197–207.
- Sharma R, Muttil P, Yadav AB, et al. Uptake of inhalable microparticles affects defense responses of macrophages infected with Mycobacterium tuberculosis H37Ra. J Antimicr Chemother. 2007;59:499–506.
- Yadav AB, Misra A. Enhancement of apoptosis of THP-1 cells infected with Mycobacterium tuberculosis by inhalable microparticles and relevance to bactericidal activity. Antimicrob Agents Chemother. 2007;51:3740–3742.
- Soto ER, Caras AC, Kut LC, et al. Glucan particles for macrophage targeted delivery of nanoparticles. J. Drug Deliv. 2012;2012:1.
- Soto ER, Kim YS, Lee J, et al. Glucan particle encapsulated rifampicin for targeted delivery to macrophages. Polymers. 2010;2:681–689.
- Upadhyay TK, Fatima N, Sharma D, et al. Preparation and characterization of beta-glucan particles containing a payload of nano-embedded rifabutin for enhanced targeted delivery to macrophages. Excli J. 2017;7:210–228.
- Soto ER, O’Connell O, Dikengil F, et al. Targeted delivery of glucan particle encapsulated gallium nanoparticles inhibits HIV growth in human macrophages. J Drug Deliv. 2016;2016:1.
- Bass DA, Parce JW, Dechatelet LR, et al. Flow cytometric studies of oxidative product formation by neutrophils: a graded response to membrane stimulation. J Immunol. 1983;130:1910–1917.
- Scott JA, Homcy CJ, Khaw BA, et al. Quantitation of intracellular oxidation in a renal epithelial cell line. Free Radic Biol Med. 1988;4:79–83.
- Jamaati H, Mortaz E, Pajouhi Z, et al. Nitric oxide in the pathogenesis and treatment of tuberculosis. Front Microbiol. 2017;8:2008–2008.
- Jang SA, Park SK, Lim JD, et al. The comparative immunomodulatory effects of β-glucans from yeast, bacteria, and mushroom on the function of macrophages. Jfn. 2009;14:102–108.
- Ahamad MS, Siddiqui S, Jafri A, et al. Induction of apoptosis and anti-proliferative activity of naringenin in human epidermoid carcinoma cell through ROS generation and cell cycle arrest. PLoS One. 2014;9:e110003.
- Lewandowska U, Szewczyk K, Owczarek K, et al. Flavanols from evening primrose (Oenothera paradoxa) defatted seeds inhibit prostate cells invasiveness and cause changes in Bcl-2/Bax mRNA ratio. J Agric Food Chem. 2013;61:2987–2998.
- Kumari A, Kakkar P. Lupeol protects against acetaminophen-induced oxidative stress and cell death in rat primary hepatocytes. Food Chem Toxicol. 2012;50:1781–1789.
- Veena VK, Popavath RN, Kennedy K, et al. In vitro antiproliferative, pro-apoptotic, antimetastatic and anti-inflammatory potential of 2,4-diacetylphloroglucinol (DAPG) by Pseudomonas aeruginosa strain FP10. Apoptosis. 2015;20:1281–1295.
- Fatima N, Upadhyay T, Sharma D, et al. Particulate beta-glucan induces early and late phagosomal maturation in murine macrophages. Front Biosci (Elite Ed). 2017;9:129–140.
- Van Crevel R, Ottenhoff TH, van der Meer JW. Innate immunity to Mycobacterium tuberculosis. Clin Microbiol Rev. 2002;15:294–309.
- Weiss G, Schaible UE. Macrophage defense mechanisms against intracellular bacteria. Immunol Rev. 2015;264:182–203.
- Lerner TR, Borel S, Gutierrez MG. The innate immune response in human tuberculosis. Cell Microbiol. 2015;17:1277–1285.
- Attwood EM, Weich DJV, Oosthuizen JMC. Influence of carbon particles on superoxide and hydrogen peroxide radical release during the killing of Mycobacterium bovis by alveolar macrophages. Tuber Lung Dis. 1996;77:462–467.
- Yadav AB, Sharma R, Muttil P, et al. Inhalable microparticles containing isoniazid and rifabutin target macrophages and “stimulate the phagocyte” to achieve high efficacy. Indian J Exp Biol. 2009;47:469–474.
- MacMicking JD, North RJ, LaCourse R, et al. Identification of nitric oxide synthase as a protective locus against tuberculosis. Proc Natl Acad Sci USA. 1997;94:5243–5248.
- Bredt DS, Snyder SH. Nitric oxide: a physiologic messenger molecule. Annu Rev Biochem. 1994;63:175–195.
- Chan J, Xing Y, Magliozzo RS, et al. Killing of virulent Mycobacterium tuberculosis by reactive nitrogen intermediates produced by activated murine macrophages. J Exp Med. 1992;175:1111–1122.
- Voskuil MI, Bartek IL, Visconti K, et al. The response of Mycobacterium tuberculosis to reactive oxygen and nitrogen species. Front Microbiol. 2011;2:105.
- Robinson JM. Phagocytic leukocytes and reactive oxygen species. Histochem Cell Biol. 2009;131:465–469.
- Cooper AM, Segal BH, Frank AA, et al. Transient loss of resistance to pulmonary tuberculosis in p47(phox-/-) mice. Infect Immun. 2000;68:1231–1234.
- Yang CS, Shin DM, Kim HK, et al. NADPH Oxidase 2 interaction with TLR2 is required for efficient innate immune responses to Mycobacteria via Cathelicidin expression. J Immunol. 2009;182:3696–3705.
- Vergne I, Chua J, Singh SB, et al. Cell biology of Mycobacterium tuberculosis phagosome. Annu Rev Cell Dev Biol. 2004;20:367–394.
- Rohde K, Yates RM, Purdy GE, et al. Mycobacterium tuberculosis and the environment within the phagosome. Immunol Rev. 2007;219:37–54.
- Gutierrez MG, Master SS, Singh SB, et al. Autophagy is a defense mechanism inhibiting BCG and Mycobacterium tuberculosis survival in infected macrophages. Cell. 2004;119:753–766.
- Deretic V. Autophagy in innate and adaptive immunity. Trends Immunol. 2005;26:523–528.
- Deretic V. Autophagy as an innate immunity paradigm: expanding the scope and repertoire of pattern recognition receptors. Curr Opin Immunol. 2012;24:21–31.
- Gan H, Lee J, Ren F, et al. Mycobacterium tuberculosis blocks cross linking of annexin-1 and apoptotic envelope formation on infected macrophages to maintain virulence. Nat Immunol. 2008;9:1189–1197.
- Behar SM, Divangahi M, Remold HG. Evasion of innate immunity by Mycobacterium tuberculosis: is death an exit strategy? Nat Rev Microbiol. 2010;8:668–674.
- Gil D, Garcia LF, Rojas M. Modulation of macrophage apoptosis by antimycobacterial therapy: physiological role of apoptosis in the control of Mycobacterium tuberculosis. Tox Appl Pharmacol. 2003;190:111–119.
- Saraste A, Pulkki K. Morphologic and biochemical hallmarks of apoptosis. Cardiovasc Res. 2000;45:528–537.
- Lawlor C, O’Connor G, O’Leary S, et al. Treatment of Mycobacterium tuberculosis-infected macrophages with poly (lactic-co-glycolic acid) microparticles drives NF kappa B and autophagy dependent bacillary killing. PLoS One. 2016;11:e0149167.
- Kalluru R, Fenaroli F, Westmoreland D, et al. Polylactide-co-glycolide-rifampicin-nanoparticles efficiently clear Mycobacterium bovis BCG infection in macrophages and remain membrane-bound in phago-lysosomes. J Cell Sci. 2013;126:3043–3054.
- Akramiene D, Kondrotas A, Didziapetriene J, et al. Effects of beta-glucans on the immune system. Medicina (Kaunas). 2007;43:597–606.
- Chan GCF, Chan WK, Y Sze DM. The effects of beta-glucan on human immune and cancer cells. J Hematol Oncol. 2009;2:25.
- Novak M, Vetvicka V. Glucans as biological response modifiers. Emiddt. 2009;9:67–75.
- Hunter KW, Gault RA, Berner MD. Preparation of microparticulate beta-glucan from Saccharomyces cerevisiae for use in immune potentiation. Lett Appl Microbiol. 2002;35:267–271.
- Tabata Y, Ikada Y. Macrophage phagocytosis of biodegradable microspheres composed of L‐lactic acid/glycolic acid homo‐and copolymers. J Biomed Mater Res. 1988;22:837–858.
- Schepetkin IA, Quinn MT. Botanical polysaccharides: macrophage immunomodulation and therapeutic potential. Int J Immunopharmacol. 2006;6:317–333.