Abstract
We describe the ex vivo expansion of haematopoietic stem/progenitor cells (HSPCs) with consideration of their eventual in-vivo niche. We firstly fabricated hierarchically structured scaffolds (lattices derived via three-dimensional plotting combined with electrospun submicron fibers coated with vitronectin to increase cell affinity). We also applied intermittent hydrostatic pressure (IHP) to mimic the physical environment of the in vivo niche. In the absence of mechanical stimuli, the cell phenotype (CD34+, CD34+CD38–) remained excellent in the vitronectin-treated group. Two IHP regimens were tested; optimally, cells were pressurized (20 kPa) for 2 min and then rested for 13 min. On day 7 of culture, the total cell number had increased 21.2-fold and that of CD34+ cells 10.94-fold. CD34+ and CD34+CD38– cells constituted 44.50 and 44.07% of total cells, respectively. Colony-forming counts and the long-term culture-initiating cell assay showed that clonogenic potential was greatly improved under our experimental conditions. Scaffolds with hierarchical structures were valuable in this context. Furthermore, ex vivo expansion of HSPCs was improved by physical stimulation.
Introduction
Haematopoietic stem/progenitor cells (HSPCs) are the forerunners of several types of blood cells and exhibit excellent self-renewal capacities [Citation1–3]. Therefore, HSPC transplantation is widely used to treat various blood and/or immune system-related diseases, such as multiple myeloma and lymphoma [Citation4]. Harvesting of bone marrow (BM) and peripheral blood (PB) (sources of HSPCs) is associated with high-level donor risks. However, it is relatively easy to obtain HSPCs from umbilical cord blood (UCB); such cells proliferate better than BM- and PB-derived cells. As might be expected, the number of cells available from a single umbilical cord is limited [Citation4–7]. Ex vivo expansion of HSPCs is thus essential. HSPCs reside in a complex niche containing secreted factors, adhesion molecules, physical factors and extracellular matrix (ECM) components; the cells engage in cell-to-cell interactions and are exposed to mechanical stimuli. All of these factors affect cell growth, differentiation, and mobilization in a complex manner [Citation8,Citation2]. Earlier studies expanded HSPCs in media containing various cytokines and growth factors characteristic of the in vivo niche [Citation9]. However, although proliferation was robust, differentiation caused phenotypic loss, associated with poor transplantation efficiency and loss of engraftment potential. Co-culture of HSPCs and stromal cells (which neighbour HSPCs in the in vivo BM) supplemented with cytokines has been used to expand HSPCs. However, cross-contamination is possible and long-term engraftment is poor. Thus, many researchers have constructed three-dimensional (3D) environments mimicking the in vivo niches of HSPCs [Citation10–12] in an effort to enhance cell attachment to structural supports and enable cell-cell and cell-matrix interactions, thus improving cellular potential [Citation6,Citation13,Citation14]. Therefore, when functional ex vivo expansion of HSPCs is essential, the 3 D culture conditions should first be considered.
Many 3 D fibrous environments mimicking in vivo cell niches have been reported [Citation6,Citation12–16], but more work is required. Previously, we developed scaffolds with structures similar to that of the ECM via rapid prototyping and electrospinning [Citation17]. We found that these supports greatly increased total nucleated cell (TNC) numbers and that the HSPC phenotype was better-maintained than in 2 D culture. Cells hang from fibres rather than attaching to a substrate over a large area, thus enhancing proliferation. Here, we structurally supplemented our 3 D scaffold and mimicked the physical microenvironment by growing cells in a bioreactor.
Many studies have used various bioreactors focusing on shear force among the physical factors present in the niche. Perfusion chambers [Citation18,Citation19], stirred tanks [Citation20,Citation21], and rotating-wall vessels [Citation22] facilitate cell expansion by providing homogeneous and dynamic environments. However, the limitations have included poor reproducibility and poor clonogenic potential. The present study introduces an adjustable hydrostatic pressure using a bioreactor in view of the fact that there is a shear force as well as a bone marrow pressure in the bone marrow space. This pressure is in the form of hydrostatic pressure, which acts equally on a part of the inner surface of the bone marrow. The hydrostatic pressure values of the marrow in mammals reported in the literature vary from 10.7 to 120 mm Hg and are generally known to be about one-fourth of the systemic blood pressure [Citation23]. These hydrostatic pressures have been reported to cause intracellular biochemical signals and play an important role in the maintenance of cell properties and function [Citation24]. Nevertheless, there are few studies on the application of hydrostatic pressure in bone marrow, and further studies on synergy with 3 D structure have not been conducted. Therefore, this study was intended to expand the HSPCs while maintaining the immunophenotype by applying hydrostatic pressure, one of the important elements of in vivo niche, in the 3 D culture system.
Materials and methods
Preparation of three-layered hierarchical scaffolds
Each layer of the hierarchical scaffold features a lattice and a fibrous mesh fabricated via 3 D plotting and electrospinning, respectively. After a lattice is fabricated, a fibrous mesh is electrospun onto the lattice, and then, another lattice is placed. This is repeated to create a three-layered hierarchical scaffold (). The 3 D plotting mixture was 10% (w/v) hydroxyapatite (HA; Sigma-Aldrich , St-Louis, MO, USA) in polycaprolactone (PCL; Polysciences Inc, Northampton, UK). The mixture was melted in a cylinder (∼120 °C) and extruded under a pressure of 650 ± 50 kPa. Polyurethane (PU; Dow Chemical, Midland, MI, USA) was used for electrospinning. PU was dissolved in N,N-dimethylformamide (N,N-DMF; Junsei Chemical, Tokyo, Japan) to 20% (w/v) at room temperature. Under a potential of 15 kV, the distance between the needle tip and the collector was set to 30 cm. The prepared scaffolds were immersed in 70% (v/v) ethanol for 1 h, washed with deionized water, and sterilized under ultraviolet light for 1 h. Scaffold size was adjusted to fit the wells of 48-well commercial culture plates (5 × 5 × 3 mm3) (). The scaffolds were immersed in a vitronectin (VN; Sigma-Aldrich) solution (5 μg/mL) for 2 h at 37 °C; the protein-coated the surfaces. The scaffolds were then washed three times with phosphate-buffered saline (PBS; Sigma-Aldrich). Non-treatment with VN is indicated by use of the descriptor “Non”.
Cell preparation and seeding
Human UCB-HSPCs were purchased from StemCell Technologies (Vancouver, BC, Canada) and cultured in accordance with the manufacturer’s guidelines. Immediately after purchase, 98% of cells expressed CD34, as revealed by flow cytometry. The HSPCs were cultured in StemSpan™ SFEM II serum-free expansion medium (StemCell Technologies, Vancouver, BC, Canada) supplemented with recombinant human stem cell factor (SCF; 50 ng/mL; ProSpec, Rehovot, Israel), recombinant human FMS-related tyrosine kinase 3 ligand (FLT-3l; 50 ng/mL; ProSpec) and recombinant human thrombopoietin (TPO; 20 ng/mL; ProSpec). Each well of a 48-well plate contained a single scaffold, in which 5 × 104 cells were suspended in 500 μL medium. Half of the medium was replaced with fresh medium every other day. All cultures proceeded in a conventional incubator at 37 °C under 5% [v/v] CO2.
Mechanical stimulation
A commercial bioreactor (ACBC-100; AnyCasting , Gimhae, South Korea) was used to deliver IHP. This device, which was also used in other related studies [25,26], consists of a vacuum pump, a chamber in which a culture dish can be placed, and a controller. The controller was designed to control the pressure magnitude and its cycle in a chamber containing 48 wells. During the stimulation, the chamber was kept in a 5% CO2 incubator at 37 °C. After culture stabilization for 48 h, IHP (20 kPa) was applied on each of 3 days for 4 h a day [Citation23]; we collected data on days 1, 4, and 7 and sought to confirm a continued influence of mechanical stimulation even after IHP ceased. We used two frequency patterns. Thus, the group exposed to IHP was divided into two subgroups: P(1/7) and P(2/13). The first number reflects the duration of pressurization and the second the rest period, in a min. The total pressure application times over 4 h were near-identical, although the application patterns differed.
Scanning electron microscopy
The hierarchical scaffolds and attached HSPCs were observed using a scanning electron microscope (SEM; Hitachi Science System Ltd., Tokyo, Japan). Scaffolds with attached cells were washed twice in PBS followed by fixation in 4% (v/v) paraformaldehyde (Sigma-Aldrich), dehydrated through a series of ethanol baths (50, 60, 70, 80, 90, and 100% v/v) for 2 min each time, dried under sterile conditions for 1 day, sputter-coated with gold for 100 s and observed using an acceleration voltage of 15 kV.
Cell counting
TNCs were counted after staining with trypan blue. Cells were harvested using Hank’s enzyme-free cell dissociation buffer (Gibco, Carlsbad, CA, USA) on days 1, 4, and 7. A Countess II FL Automated Cell Counter (Thermo Fisher Scientific Inc., Waltham, MA, USA) was used to enumerate cells of diameter 7–20 μm.
Flow cytometry
Among the many markers expressing phenotypes of HSPCs, this study analyzed CD34+ and a primitive marker of CD34+ CD38−- based on the recent studies especially focused on clinical trials [27–30]. For this, HSPCs were incubated with polyethylene (PE)-tagged mouse anti-human CD34 (BD Biosciences, San Jose, CA, USA) and fluorescein isothiocyanate (FITC)-tagged mouse anti-human CD38 (BD Biosciences) antibodies, and an isotype control antibody for compensation. In brief, cells were washed with staining buffer (0.2% bovine serum albumin [BSA] and 0.09% sodium azide [both w/v] in PBS). Non-specific binding was blocked by incubation at 4 °C for 30 min in a buffer with 5% (w/v) BSA and 8 mM EDTA. The cells were then washed, stained with fluorescent dye-conjugated antibodies for 30 min at 4 °C, washed with staining buffer and suspended in 1% (v/v) paraformaldehyde (USB , Fremont, CA, USA). A total of 10,000 events were acquired by an Attune NxT acoustic focusing cytometer (Life Technologies, Carlsbad, CA, USA) and analyzed with the aid of Attune NxT Software (ver. 2.7.0; Life Technologies).
Colony-forming cell assay
Hematopoietic potency was evaluated using the colony-forming cell (CFC) assay. On day 7, HSPCs were suspended in a semi-solid methylcellulose medium (MethoCult GF H4434; StemCell Technologies) and plated (in triplicate) in 35-mm-diameter Petri dishes at 1 × 103 cells/dish, followed by incubation in a conventional incubator (under 5% [v/v] CO2 at 37 °C) for 14 days. CFCs were counted on day 14, and morphologically distinguished: burst-forming unit-erythroids (BFU-E), colony-forming unit-granulocytes/macrophages (CFU-GM), and colony-forming unit-granulocytes/erythroids/macrophages/megakaryocytes (CFU-GEMM).
Long-term culture-initiating cell assay
The clonogenic potential of HSPCs after prolonged incubation was examined with the long-term culture-initiating cell (LTC-IC) assay. Bone marrow stromal cells (line M2-10B4; Korean Cell Line Bank, Seoul, South Korea) treated with mitomycin C (20 μg/mL, 3 h; Sigma-Aldrich) served as the feeder layer [Citation31,Citation32]; these cells were seeded into 35-mm-diameter Petri dishes at 3 × 105 cells/dish. After 24 h, HSPCs (5 × 105 cells) were seeded onto the feeder layer. The medium (2.5 ml) was MyeloCult H5100 (StemCell Technologies) containing 10−6 M hydrocortisone (Sigma-Aldrich). The plates were incubated for 5 weeks in a conventional incubator (under 5% [v/v] CO2 at 37 °C); half of the medium was replaced with fresh medium every week. After 5 weeks, all cells were harvested and seeded into 35-mm-diameter Petri dishes at 5 × 104 cells/dish and cultured for an additional 14 days in H4435 medium (StemCell Technologies). LTC-ICs were counted and the numbers normalized as recommended by StemCell Technologies.
Statistical analyses
One-way analysis of variance was performed with SPSS software (ver. 12.0 K; SPSS Inc., Chicago, IL, USA) using the least significant difference method. All data are expressed as means ± standard deviation (SD); a p values ≤ .05 was considered to reflect statistical significance.
Results
Morphology of HSPCs growing on three-layered hierarchical scaffolds
The representative SEM images in show changes in HSPCs attached to scaffolds, especially to the contained fibres, over time. The numbers of attached HSPCs increased in all groups. Even in the absence of mechanical stimulation, VN treatment (VN) improved proliferation and adhesion. Mechanical stimulation after VN treatment of groups P(1/7) and P(2/13) further enhanced proliferation and adhesion. At day 7 (thus 3 days after mechanical stimulation ceased), the cells were more clustered than immediately after stimulation (day 4), suggesting that the effect of the stimulus persisted even after cessation. The P(1/7) and P(2/13) group patterns differed on day 7; phenotypic analysis by the group was thus required.
Changes in nucleated cell numbers
shows the increases in TNC numbers over time and the fold increases by day 7 compared to day 1. Three-day stimulation was effective immediately, that is, on day 4. Moreover, the effect continued to day 7 but differed by stimulus pattern. The fold increase in TNC numbers was highest (>20.0) in the P(2/13) group, thus significantly greater than that in the P(1/7) group.
HSPC phenotypes during ex vivo expansion
The proportions of cells expressing the HSPC phenotype (CD34+ and CD34+CD38–; a typical and primitive marker, respectively) over time were measured by flow cytometry. The average expression levels just before seeding were 95.0 and 82.4%, respectively. ) shows that phenotypic expression decreased over time. Twenty-four hours after seeding (day 1), VN facilitated phenotypic maintenance. On days 4 and 7, higher proportions of mechanically stimulated cells than controls retained the HSPC phenotype. Particularly, on day 7, P(2/13) cells were significantly better in this context than P(1/7) cells. The total numbers of cells with the desired phenotypes are shown in . shows the average fold increases in cells expressing the desired phenotype on day 7 relative to the day 1 numbers. The beneficial effect of mechanical stimulation is evident, as is the significance of the stimulation pattern; the P(2/13) group exhibited a significantly higher -fold increase than the other groups.
Figure 4. Flow cytometry analysis of HSPCs cultured under different conditions. (A) The typical HSPC marker CD34+. (D) The primitive HSPC marker CD34+CD38− (%). (B) Absolute CD34+ cell numbers. (E) Absolute CD34+CD38− cell numbers. Cells were collected on days 1, 4, and 7. (C) CD34+ cell-fold increases. (F) CD34+CD38− cell-fold increases on day 7. (n = 10). (*p < .05).
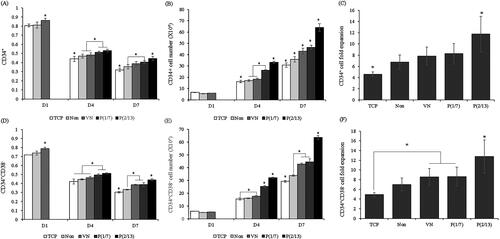
Clonogenic potential of expanded cells
To evaluate colony-forming ability, cells were cultured for 14 days, and the CFC assay was then performed (). The mechanically stimulated groups formed large numbers of colonies (). The P(2/13) group exhibited better colony-forming ability (all three colony types) than the non-stimulated groups. The P(1/7) group also exhibited high colony-forming ability, with the exception of GEMM colonies. Data regarding the number of colonies formed by each group divided by the number of colonies formed by the tissue culture polystyrene (TCP; simple 2D) group are shown in . The P(2/13) group formed almost twice as many colonies as the TCP group. Representative micrographs of each P(2/13) colony type are shown in .
Figure 5. The colony-formation capacity of expanded cells. HSPCs were collected on day 7 and then grown under different culture conditions. (A), (B): BFU-E, CFU-GM, CFU-GEMM, and total colony counts after 14 days of growth. (C) The colony numbers formed by each group divided by the colony numbers of the TCP group. (D) Representative images of P(2/13) BFU-E, CFU-GM, and CFU-GEMM colonies formed after 7 days (n = 3). BFU-E: burst-forming unit-erythroid; CFU-GM: colony-forming unit-granulocyte and macrophage; CFU-GEMM: colony-forming unit-granulocyte, erythrocyte, monocyte, and megakaryocyte (BFU-E and CFU-GEMM, bar = 300 μm; CFU-GM, bar = 400 μm) (*p < .05).
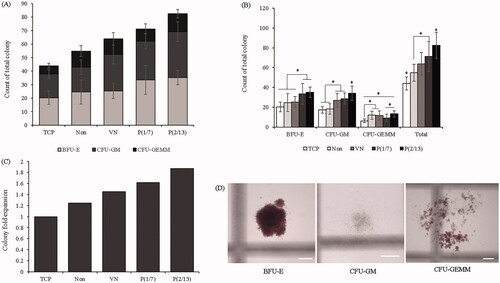
Similar results were also observed using the LTC-IC in . Mechanical stimulation greatly enhanced secondary clonogenic potential, which is critical if HSPCs are functional in vivo, and secondary clonogenic potential was affected by the stimulation pattern. Among the non-stimulated groups, the VN group produced more secondary colonies somewhat better than did the TCP group, but a statistically significant compared to the “Non” group was not attained.
Discussion
When studying cell growth in vitro, the environment should ideally be identical to that in vivo; many studies use biochemical modulators to this end. In recent years, the importance of the physical environment has become recognized [Citation23]. The shape, nature, strength, and physical properties of materials surrounding cells in vivo are important, as are mechanical stimuli imparted voluntarily or involuntarily. We sought to reproduce the in vivo environment of non-adherent HSPCs; the cells were suspended from electrospun submicron fibers and thus not firmly attached to an artificial substrate. We fabricated 3 D lattices by adding HA to PCL, which is commonly used for bone regeneration because of its affinity for bone [Citation33]. The lattices were three-layered, with PU submicron fibers between each layer; PU binds strongly to cells. ECM proteins enhance cell/substrate binding and play important roles in chemical and biological signal transduction [Citation34]. Therefore, the scaffolds were coated with an ECM protein. We tested both VN and fibronectin in this context and selected the former; VN exhibited optimal cellular affinity and enhancement of proliferation (data not shown). However, the optimal protein may vary by cell and substrate type. Also, we applied mechanical stimuli to further mimic the in vivo environment. VN coating increased cell numbers and facilitated phenotypic retention (CD34+, CD34+CD38–) compared to TCP (simple 2D) as control and uncoated (“Non”) groups (). The CFC and LTC-IC assays showed that the clonogenic potential of the VN group was excellent.
Earlier studies also employed submicron fibers. Mousavi et al. [Citation29] reported that fibronectin-coated PCL nanofibres enhanced CD34+ cell proliferation 2.66-fold in simple 2 D culture, and 40-fold in scaffold culture, compared to bare nanofibres. Sabaqhi et al. [Citation13] reported that a 3 D aminated polyethersulphone nanofibre scaffold increased the in vitro proliferation of CD34+ cells 1.8-fold over 10 days compared to a 2 D culture. Thus, HSPC proliferation on nanofibres is significantly better than 2 D proliferation, as we confirmed. However, the cited works did not consider the mechanical environment of the in-vivo niche, only the 3 D environment.
We sought to optimize both the physical and geometric features encountered by HSPCs in vivo. Previous studies also applied mechanical stimulation. Stirred tank, fixed-bed, agitated, and rotating-wall bioreactors have all been used for ex vivo HSPC expansion. Hosseinizand et al. [Citation35] used an agitation bioreactor operating at 40 rpm; total cell proliferation was maximal by day 14. However, engraftment capacity was not confirmed, and total cell fold expansion was disappointing (∼5-fold) despite long-term incubation (14 days). Liu et al. [Citation22], using a rotating-wall vessel, reported increases of 435.5 ± 87.6-fold (total cells), 32.7 ± 15.6-fold (CD34+ cells), and 21.7 ± 4.9-fold (CFU-GM) by day 8. The CD34+ proportion of total cells was small (expansion folding ratio = ∼7.51%). Also, engraftment capacity was not explored. To overcome these limitations, we applied IHP to HSPCs because such cells are exposed to hydrostatic pressure in the BM [Citation23]. The duration was arbitrary (4 h daily on each of 3 successive days). We used two different patterns, as described above. We found that the total cell number increased 21.2-fold, the number of CD34+ cells increased only 10.94-fold, thus by 51.5% (expansion folding ratio) that of all cells. Our methods can be used to enhance ex vivo proliferation and phenotypic maintenance of HSPCs.
However, our study had certain limitations. We used UCB cells and commercial cell lines. Therefore, the addition of various cytokines was inevitable, although we employed only three. In clinical practice, it is difficult to ensure that cytokines will produce consistent results when donor- or patient-derived cells are cultivated. In addition, culture scale-up remains a major issue. Clinical practitioners generally consider that at least 2.5 × 106 CD34+ cells/kg are needed for effective treatment. Thus, a patient weighing 60 kg requires at least 1.5 × 108 CD34+ cells, not TNCs [Citation30]. Therefore, culture scale-up is required. It is not easy to process large numbers of cells that may contain only small proportions of CD34+ cells.
Although some issues thus remain, it is encouraging that both IHP and our scaffold facilitated cell growth and phenotypic retention. Various extents and patterns of IHP should be further explored to allow the clinical application. Cells acquired from donors and patients require evaluation, as does scale-up.
Disclosure statement
The authors declare that there is no conflict of interest regarding the publication of this article.
Additional information
Funding
References
- Warr MR, Pietras EM, Passeque E. Mechanisms controlling hematopoietic stem cell function during normal hematopoiesis and hematological malignancies. Wires Syst Biol Med. 2011;6:681–701.
- Mendelson A, Frenette PS. Hematopoietic stem cell niche maintenance during homeostasis and regeneration. Nat Med. 2014;20:833.
- Bryder D, Rossi DJ, Weissman IL. Hematopoietic stem cells: the paradigmatic tissue-specific stem cell. Am J Pathol. 2006;169:338–346.
- Kelly SS, Sola CBS, Lima DM, et al. Ex vivo expansion of cord blood. Bone Marrow Transplant. 2009;44:673.
- Rocha V, Labopin M, Sanz G, et al. Transplants of umbilical-cord blood or bone marrow from unrelated donors in adults with acute leukemia. N Engl J Med. 2004;351:2276–2285.
- Batnyam O, Shimizu H, Saito K, et al. Biohybrid hematopoietic niche for expansion of hematopoietic stem/progenitor cells by using geometrically controlled fibrous layers. RSC Adv. 2015;5:80357–80364.
- Tung SS, Parmar S, Robinson SN, et al. Ex vivo expansion of umbilical cord blood for transplantation. Best Pract Res Clin Haematol. 2010;23:245–257.
- Lane SW, Williams DA, Watt FM. Modulating the stem cell niche for tissue regeneration. Nat Biotechnol. 2014;32:795.
- Eridani S, Mazza U, Massaro P, et al. Cytokine effect on ex vivo expansion of haemopoietic stem cells from different human sources. Biotherapy. 1998;10:295–298.
- Soffer-Tsur N, Peer D, Dvir T. ECM-based macroporous sponges release essential factors to support the growth of hematooietic cells. J Control Release. 2017;257:84–90.
- Severn CE, Macedo H, Eagle MJ, et al. Polyurethane scaffolds seeded with CD34+ cells maintain early stem cells whilst also facilitating prolonged egress of haematopoietic progenitors. Sci Rep. 2016;6:32149.
- Sabaqhi F, Shamsasenjan K, Movasaqhpour AA, et al. Evaluation of human cord blood CD34+ hematopoietic stem cell differentiation to megakaryocyte on aminated PES nanofiber scaffold compare to 2-D culture system. Artif Cells Nanomed Biotechnol. 2016;44:1062–1068.
- Chua KN, Chai C, Lee PC, et al. Functional nanofiber scaffolds with different spacers modulate adhesion and expansion of cryopreserved umbilical cord blood hematopoietic stem/progenitor cells. Exp Hematol. 2007;35:771–781.
- Ferreira MSV, Jahnen-Dechent W, Labude N, et al. Cord blood-hematopoietic stem cell expansion in 3D fibrin scaffolds with stromal support. Biomaterials. 2012;33:6987–6997.
- Mousavi SH, Abroun S, Soleimani M, et al. 3-Dimensional nano-fibre scaffold for ex vivo expansion of cord blood haematopoietic stem cells. Artif Cells Nanomed Biotechnol. 2018;46:740–748.
- Li Y, Ma T, Kniss DA, et al. Human cord cell hematopoiesis in three-dimensional nonwoven fibrous matrices: In vitro simulation of the marrow microenvironment. J Hematother Stem Cell Res. 2001;10:355–368.
- Kang YG, Shin JW, Park SH, et al. A three-dimensional hierarchical scaffold fabricated by a combined rapid prototyping technique and electrospinning process to expand hematopoietic stem/progenitor cells. Biotechnol Lett. 2016;38:175–181.
- Palsson BO, Paek SH, Schwartz RM, et al. Expansion of human bone marrow progenitor cells in a high cell density continuous perfusion system. Nat Biotechnol. 1993;11:368.
- Koller MR, Bender JG, Miller WM, et al. Expansion of primitive human hematopoietic progenitors in a perfusion bioreactor system with IL-3, IL-6, and stem cell factor. Nat Biotechnol. 1993;11:358.
- Sardonini CA, Wu YJ. Expansion and differentiation of human hematopoietic cells from static cultures through small-scale bioreactors. Biotechnol Prog. 1993;9:131–137.
- Li Q, Liu Q, Cai H, et al. A comparative gene-expression analysis of CD34+ hematopoietic stem and progenitor cells grown in static and stirred culture systems. Cell Mol Biol Lett. 2006;11:475.
- Liu Y, Liu T, Fan X, et al. Ex vivo expansion of hematopoietic stem cells derived from umbilical cord blood in rotating wall vessel. J Biotechnol. 2006;124:592–601.
- Gurkan UA, Akkus O. The mechanical environment of bone marrow: a review. Ann Biomed Eng. 2008;36:1978–1991.
- Guilak F, Cohen DM, Estes BT, et al. Control of stem cell fate by physical interactions with the extracellular matrix. Cell Stem Cell. 2009;5:17–26.
- Kim DH, Kim SH, Heo SJ, et al. Enhanced differentiation of mesenchymal stem cells into NP-like cells via 3D co-culturing with mechanical stimulation. J Biosci Bioeng. 2009;108:63–67.
- Jeong JY, Kim DH, Park SH, et al. Effects of PDMS surface patterns on differentiation and proliferation of MSCs under intermittent hydrostatic pressure. Tissue Eng Regen Med. 2010;7:283–290.
- Huang X, Zhu B, Wang X, et al. Three-dimensional co-culture of mesenchymal stromal cells and differentiated osteoblasts on human bio-derived bone scaffolds supports active multi-lineage hematopoiesis in vitro: functional implication of the biomimetic HSC niche. Int J Mol Med. 2016;38:1141–1151.
- Mousavi SH, Abroun S, Soleimani M, et al. Expansion of human cord blood hematopoietic stem/progenitor cells in three-dimensional nanoscaffold coated with fibronectin. Int J Hematol-Oncol Stem Cell Res. 2015;9:72.
- Huang X, Li C, Zhu B, et al. Co-cultured hBMSCs and HIVECs on human bio-derived bone scaffolds provide support for the long-term ex vivo culture of HSC/HPCs. J Biomed Mater Res. 2016;104:1221–1230.
- Fong CY, Gauthaman K, Cheyyatraivendran S, et al. Human umbilical cord Wharton’s jelly stem cells and its conditioned medium support hematopoietic stem cell expansion ex vivo. J Cell Biochem. 2012;113:658–668.
- Kang YG, Jeong JY, Lee TH, et al. Synergistic integration of mesenchymal stem cells and hydrostatic pressure in the expansion and maintenance of human hematopoietic/progenitor cells. Stem Cells Int. 2018;2018:1.
- Ponchio L, Duma L, Oliviero B, et al. Mitomycin C as an alternative to irradiation to inhibit the feeder layer growth in long-term culture assays. Cytotherapy. 2000;2:281–286.
- Shor L, Guceri S, Wen X, et al. Fabrication of three-dimensional polycaprolactone/hydroxyapatite tissue scaffolds and osteoblast-scaffold interaction in vitro. Biomaterials. 2007;28:5291–5297.
- Kurth I, Franke K, Pompe T, et al. Extracellular matrix functionalized microcavities to control hematopoietic stem and progenitor cell fate. Macromol Biosci. 2011;11:739–747.
- Hosseinizand H, Ebrahimi M, Abdekhodaie MJ. Agitation increases expansion of cord blood hematopoietic cells and promotes their differentiation into myeloid lineage. Cytotechnol. 2016;68:969–978.