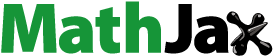
Abstract
The current clinical paradigm for ovarian cancer treatment has a poor prognosis, partially due to the efficacy and toxicity concerns associated with the available chemotherapeutic formulations. To overcome these limitations, we have designed core-shell-structured paclitaxel (PTX) laden solid lipid microparticles (PTX-SLMPs) for intraperitoneal treatment of ovarian cancer. A single-step coaxial electro hydrodynamic atomization (CEHDA) process has been explored to synthesize core-shell structure of PTX-SLMPs with the particle size of 1.76 ± 0.37 µm. Core-shell PTX-SLMPs have high encapsulation efficiency of 94.73% with sustained drug release profile. In vitro evaluation of PTX-SLMPs in SKOV-3 ovarian cancer cells yield significant enhancement in cytotoxicity when compared with Taxol®. In vivo pharmacokinetic study demonstrated slower absorption of PTX into the systemic circulation after intraperitoneal (i.p.) administration of PTX-SLMPs in Wistar rats implying the PTX-SLMPs remained in the peritoneal cavity and released the PTX for prolonged period of time. Through these studies, we have demonstrated the technical potential of core-shell structured PTX-SLMPs, which can enhance passive targeting of PTX to the tumor in the treatment of not only ovarian cancer but also in other peritoneal cancer.
Introduction
Ovarian cancer is the most lethal gynaecologic malignancy and ranks fifth in cancer-related deaths among women in the United States [Citation1]. Ovarian cancer is often diagnosed at a late stage with a high case-to-fatality ratio [Citation2]. The high mortality is associated with its rapid proliferation, frequent acquisition of chemoresistance and widespread metastasis within the peritoneal cavity [Citation3]. Seventy-five percent of patients are present with advanced disease at diagnosis, consequently the 5-year survival is less than 30% [Citation4]. The current first-line treatment of advanced ovarian cancer is cytoreductive surgery followed by administration of paclitaxel (PTX) with other chemotherapeutic agents for six cycles [Citation5,Citation6]. The therapeutic advantage of i.p. chemotherapy has been demonstrated in multiple clinical studies [Citation7,Citation8]. This is due to the unique biology of ovarian cancer where usual high-grade serous ovarian carcinoma originates from the ovarian, tubal and peritoneal surface epithelium and tends to remain in the peritoneal cavity with superficial invasion [Citation9,Citation10]. However, despite its therapeutic potential, widespread acceptance of i.p. chemotherapy in clinical treatment of ovarian cancer is hindered by toxicity, catheter-related complications, and complex administration concerns [Citation11].
PTX, as such has poor aqueous solubility, low bioavailability and is often associated with dose-limiting toxicities and other side effects [Citation12–14]. By loading PTX in different delivery systems, we have demonstrated the improved therapeutic outcome in ovarian cancer cells [Citation15,Citation16]. Solid lipid provides the platform of biocompatibility, stability at room and body temperatures, capability of trapping hydrophobic drugs and preventing them from premature degradation with a hard shell facilitating sustained drug release [Citation17,Citation18]. Solid lipid microparticles (SLMPs) are generally prepared by melt dispersion technique or solvent evaporation methods [Citation19] including spray drying, emulsification and electrospray [Citation17,Citation20]. Among them, the electrospray method exposes the flow of lipid or polymer solution from a needle in an elevated electric field to form a Taylor cone and breaks the jet into micro or nanoparticles [Citation21]. Several delivery systems, including mono-disperse Taxol-loaded poly-caprolactone (PCL) MPs, are fabricated using single axial electrospray with high encapsulation efficiency [Citation22]. This process is able to produce particles in a single step, but the resultant drug release profile is suboptimal due to the lack of a core-shell structure [Citation23]. The coaxial electro hydrodynamic atomization (CEHDA) process has the ability to overcome this limitation by producing multilayer microparticles with a core-shell structure [Citation23]. The CEHDA process not only enables the encapsulation of drugs and proteins with high efficiency but also protects their bioactivities in a well-defined core-shell structure [Citation24]. It is a highly versatile process capable of encapsulating different cargos by a variety of carrier materials [Citation25–27]. Optimizing the process parameters such as flow rate, external voltage and polymer concentration, we are able to encapsulate lipophilic or hydrophilic drugs in microparticles with the size ranging from micron to submicron and the entrapment efficiency of nearly 100% [Citation28]. By controlling the morphology and the thickness of the core-shell structure, it is feasible to achieve controlled drug release and enhance the cellular and tissue uptake [Citation29,Citation30]. Previous reports also suggest the encapsulation of more than one cargo for the applications of combinatory drug delivery and multimodal imaging [Citation31,Citation32]. Scalable production of drug-laden microparticles can also be achieved by using a multiplexed needle assembly [Citation33].
Recently, we succeeded in preparing artemether-loaded and curcumin-loaded core-shell-structured PLGA MPs using CEHDA process for different applications in our lab [Citation23,Citation34]. However, in the present study, we have developed CEHDA technology for scalable production of PTX-laden SLMPs (PTX-SLMPs) with core-shell structure in a single-step process. The core-shell-structured SLMPs have not been worked out before irrespective of its numerous advantages. The drug encapsulation and sustained release properties of PTX-SLMPs have been evaluated. The potential of PTX-SLMPs against SKOV-3 ovarian cancer cell lines has also been assessed. Our in vivo pharmacokinetics studies have demonstrated the potential of PTX-SLMPs in i.p. treatment.
Materials and methods
Materials
PTX was purchased from Hefei Bo Mei Biotechnology Co., Ltd. China. Stearic acid was purchased from Shanghai Titanchem co., ltd (Shanghai, China), cholesterol was purchased from Corden Pharma Switzerland (Liestal, Switzerland), Pluronic F-127 was purchased from Sigma Aldrich Co. (St. Louis, USA), and PEG-2000 was purchased from Sinopharm Chemical Reagent Co., Ltd, China. [3–(4, 5-dimethylthiazol-2-yl)-2, 5-diphenyltetrazolium bromide] cell viability/cytotoxicity assay kit (MTT) was obtained from Beyotime Biotechnology (Jiangsu, China). Fetal bovine serum (FBS), Trypsin-EDTA solution, Roswell Park Memorials Institute (RPMI)-1640 medium and Penicillin-streptomycin solution were supplied by Sangon Biotech (Shanghai, China). Acetone (99.9% high-performance liquid chromatography (HPLC) grade), and acetonitrile (99.9% HPLC grade) was purchased from Sigma Aldrich Chemistry (USA). The water used was pre-treated with the Milli-Q Plus System (Millipore Corporation, Bedford, USA) to obtain ultrapure water.
Methods
CEHDA process setup
SLMPs were produced by the CEHDA process as shown in . Briefly, the set-up consisted of coaxial needles (inner and outer needles), in which the inner needle (inner diameter: 0.26 mm, outer diameter: 0.51 mm) was assembled in the outer needle (inner diameter: 0.84 mm, outer diameter: 1.27 mm) in an aligned position as shown in . The inner and the outer needles were connected with two different syringes containing the inner and the outer phases. The syringes were attached to a syringe pump (Harvard Apparatus, MA) to obtain constant flow rates. The positive and the negative electrodes of high voltage DC power supply (Gamma High Voltage Research, Inc., FL) were connected to the coaxial needle and the collector, respectively. Both the positive and negative power supplies are also connected to the ground. The vertical distance between the coaxial needle and the collector electrode was 150 mm. The whole process was monitored in real time by a charge-coupled device camera (Allied vision technologies, Inc., MA) equipped with a microscopic lens (Model VT-7DS-2CD, Hangzhou, China).
Figure 1. (a) CEHDA set-up consisting of the coaxial needle, Twin syringe pump, and high-voltage DC power supplies with positive and negative electrodes connected with crocodile clip, Aluminium plate, and microscopic lens combined with CCD camera, computer and light source. (b) Inner and outer needles with a coaxial set-up.
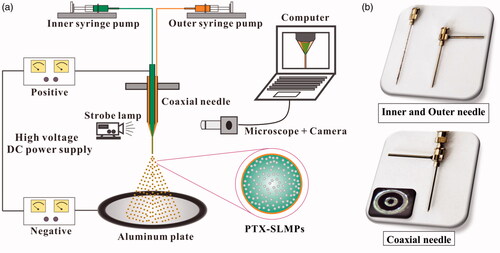
Preparation of the solutions for CEHDA
Acetone was used as the inner and the outer solvents in the process of CEHDA. In the optimized SLMPs fabrication, the solution of acetone containing 4% w/v stearic acid in presence of 2% w/v cholesterol, PEG-2000 and Pluronic F127 (0.5–1% w/v), was used as the outer solvent. 1% w/v stearic acid solution of acetone alone or having PTX (6 mM) was used as the inner phase to prepare blank (SLMPs) and drug-laden particles (PTX-SLMPs), respectively. For confocal and cell uptake studies, additional Nile red fluorescent dye was used in a concentration of 0.01% in the outer solvent containing lipid to obtain fluorescent labelled SLMPs.
Preparation of PTX-SLMPs and their physicochemical characterization
In CEHDA process, the inner and the outer solvent were injected through two separate Teflon tubes connected separately to the syringe pumps maintained at a flow rate of 10 µl min−1. The flow rate was maintained at a ratio of 1:1 for both inner and outer needles. The electric field in the assembly was established between the needle and the collector using a high power DC voltage. The positive electrode of 10 kV was applied on the top of the needles and the negative voltage of 11.5 kV was applied on the ground collector. As a result, stable coaxial liquid Taylor cone jet was formed which breaks under the influence of applied electric field. The jet breaks and during the time of its flight towards the negative electrode, the solvent evaporates. These results into PTX-SLMPs which were collected in a petri dish containing 0.1% (w/v) PVA solution kept on the aluminium plate. These MPs were collected by centrifuge using a TDZ4A-WS Centrifuge (Hefei, China) at 5000 g for 15 min at 4 °C and washed with ultrapure water for three times. The dry particles were collected by lyophilization (LGJ-10 freeze dry system, Beijing, China) using mannite (1% w/v) as the cryoprotectant. The assessments of PTX-SLMPs particle size, size uniformity and its zeta potential were carried out using dynamic light scattering, Zetasizer (Nano series, Nano-ZS90) at 25 °C. Before analysing, the PTX-SLMPs were dispersed in ultrapure water using bath sonication. The analysis was carried out in triplicate.
The size distribution of the PTX-SLMPs was also confirmed by means of an optical microscope (Model DSZ2000X, Chongqing UOP photoelectric technology Co. Ltd, China) and an image-processing program (Image-Pro Plus version 6.0 software, Media Cybernetics, Inc., USA). The diameter determination and the statistical analysis were carried out from various microphotographs.
The formation of PTX-SLMPs was continuously monitored during the fabrication process. Some of the PTX-SLMPs were collected on glass slide and were monitoring using 40x optical lens using Nikon microscope. Finally collected PTX-SLMPs were analysed for their morphological characteristics by scanning electron microscopy (SEM; Leica Stereoscan 410, Wetzlar, Germany). Samples for SEM were prepared by mounting a thin layer of PTX-SLMPs on a copper stud, which was then sputter coated with gold (SCD 050, Leica, Wetzlar, Germany) for 90 s under vacuum. The gold-coated PTX-SLMPs were used for SEM scanning.
SLMPs without PTX were prepared by avoiding the addition of PTX in inner solution of electrospray. The fluorescent labelled SLMPs were prepared by adding Nile red (0.01 wt %) in the outer solution of electrospray. The core-shell structure of the fluorescent labelled SLMPs were validated using confocal microscope (Nikon Instruments Inc., Japan). The fluorescent labelled SLMPs were mounted on the glass slide and were observed using Apo TIRF 60x/1.49 oil under the confocal microscope. The images were processed using software Nikon NIS-Elements C. All experiments were performed in triplicate.
Ultra performance liquid chromatography (UPLC) analysis
A reproducible reverse phase UPLC method was developed for determining the PTX concentration in the PTX-SLMPs with slight modifications [Citation35]. The same method was employed to quantify the amount of PTX in, in vitro dissolution studies samples as well as in the in vivo pharmacokinetics studies for plasma samples. The method used an octadecylsilane column (C18, 2.1 × 100 mm, 2.2 μm) with a water/acetonitrile/methanol gradient mobile phase at a flow rate of 0.3 ml/min, and a detection wavelength of 227 nm while the temperature of the column was maintained at 35 °C.
Entrapment efficiency and drug loading
The concentration of PTX in PTX-SLMPs was estimated using the UPLC method described above. Briefly, 10 mg PTX-SLMPs were dissolved in acetonitrile and vigorously mixed. The samples were centrifuged at 3000 rpm for 15 min and the solution containing dissolved PTX was analysed by UPLC. Percentage entrapment efficiency was calculated as follows:
PTX loading was calculated as follows:
In vitro dissolution studies
The in vitro dissolution studies were performed at 37 °C by using dialysis bag diffusion method (cut off molecular weight 2000 Da). Taxol® and PTX-SLMPs equivalent to 1 mg of PTX were suspended in a pre-activated dialysis bag. The dialysis bag was placed in 200 ml of PBS having 1% of tween 80 under constant stirring. 1 ml sample was collected at predetermined intervals (0.5, 1, 2, 4, 6, 8, 12, 18, 24, 48, 72, 96, 120, 144 and 168 h). Proper sink condition was maintained by replacing withdrawal sample with an equivalent volume of fresh medium. The amount of PTX released during the in vitro dissolution studies was estimated by the UPLC method described above. The saturation solubility studies were conducted in the release medium of phosphate buffer saline (PBS) having 1% of tween 80 in advance by UPLC method.
The release mechanism of PTX from PTX-SLMPs was evaluated using release models [Citation36]. The zero order rates, the first order rates, Korsmeyer and Peppas model, Higuchi model and the Hixson-Crowell cube root law were determined according to the EquationEqs. (1)(1)
(1) , Equation(2)
(2)
(2) , Equation(3)
(3)
(3) , Equation(4)
(4)
(4) and Equation(5)
(5)
(5) respectively.
(1)
(1)
K0 is zero-order rate constant expressed in units of concentration/time and t is the time.
(2)
(2)
C0 is the initial concentration of PTX and K is first-order constant.
(3)
(3)
Mt/M∞ is fraction of PTX released at time t, k is the rate constant and n is the release exponent.
(4)
(4)
K is the constant reflecting the design variables of the system.
(5)
(5)
Wt is the amount of PTX released in time t, W0 is the initial amount of the PTX in PTX-SLMPs and K is the rate constant for Hixson–Crowell rate equation.
In vitro cellular experiments
SKOV-3 cell culture
SKOV-3 human ovarian cancer cell lines were cultured in RPMI medium with 10% (v/v) FBS and 1% antibiotic solution placed in an incubator (Thermo Scientific, USA) at 37 °C under an atmosphere of 5% CO2 and 90% relative humidity. Cells were then allowed to grow up to 70–80% confluence and plated as per study requirement.
In vitro uptake studies by confocal microscope and flow cytometry
In vitro cellular uptake studies were performed on the monolayer of SKOV-3 cells and evaluated by flow cytometry as well as confocal fluorescence microscopy. The fluorescence-labelled PTX-SLMPs were co-cultured with the SKOV-3 cells in order to study their cellular uptake potential. For the flow cytometric study, SKOV-3 cells were seeded in a 6 well tissue culture plate at a density of 1 × 106 cells/well in RPMI medium. The cells were washed after 24 h and treated with fluorescent labelled PTX-SLMPs and free Nile red at equivalent concentrations. The treated cells were incubated at 37 °C for 4 h. After incubation, the cells were gently washed three times with PBS and were collected by trypsinization. The cells were re-suspended in phenol red free RPMI media. The fluorescent intensity was measured using flow cytometry.
The uptake studies using confocal microscope were carried out after plating the cells in a concentration of 1 × 106 on 15 mm glass bottom cell culture dish. Rest of the procedure of treating cells was the same as stated for flow cytometry uptake studies. The treated cells were fixed using ice cold methanol for 1 h at −80 °C. The nucleus of the cells were stained using 10 µL of 1 mg/mL DAPI, a nucleus staining dye. The cells were observed under confocal microscope (Nikon Instruments Inc., Japan) using 20x objective lens. The images were processed using software Image J. All experiments were performed in triplicate. The untreated cells were used as control.
Cytotoxicity studies on SKOV-3 cells
In vitro cytotoxicity of PTX-SLMPs and Taxol® was evaluated on SKOV-3 ovarian cancer cells by treating the cells at equivalent concentration. The cells were plated in 96-well tissue culture plate at a density of 5000 cells/well and incubated for 24 h. Before the experiment, the cells were washed with PBS. Subsequently, the medium was exchanged with fresh media containing Taxol® and PTX-SLMPs at equivalent concentration in the range of 1 nM–10 µM. The treated cells were incubated for 24, 48 and 72 h followed by the addition of 10 µL of MTT solution (5 mg/mL) to each well. The cells were again incubated for 4 h, and finally, 100 µL DMSO was added to each well to dissolve formazan crystals and was incubated overnight to allow cell lysis at 37 °C. Optical density of each well was measured by a micro-plate spectrophotometer at 470 nm, and accordingly, cell viability was calculated and plotted in the graph against PTX concentration.
Apoptosis assays
The apoptosis analysis was carried out to determine the mode and extent of apoptosis using Annexin V and propidium iodide (PI) dual staining method after the treatment with PTX-SLMPs. SKOV-3 ovarian cancer cell lines seeded in a 6-well plate at a density of 1 × 106, were treated with Taxol® and PTX-SLMPs at an equivalent concentration of 1 µM for 48 h. The 48-h time interval was chosen based on the doubling time of the employed cells. Followed by the treatment, cells were washed with PBS, trypsinized and collected by centrifugation. The apoptosis assay was carried out according to the protocol provided by the company. Briefly, the collected cells were washed with PBS twice and suspended in 400 µL of 1× Annexin V solvent provided by the company. 5 µL of Annexin V-FITC was added to the cells suspension and incubated for 15 min at 2–8 °C. Subsequently, 10 µL of propidium iodide (PI) staining was added to the above solution and again incubated for 5 min at 2–8 °C. The flow cytometry analysis was carried out immediately after addition of PI.
In vivo pharmacokinetic studies
Female wistar rats of 3 weeks were purchased from Beijing Vital River Laboratory Animal Technology Co., Ltd. (Beijing, China) and kept in the Animal Care Centre Facility of University of Science and Technology of China (USTC). Rats were housed in well-ventilated cages at room temperature (25 ± 3 °C) and 40–60% relative humidity. Approval from the Local Animal Ethics Committee of USTC (under the Protocol No: U USTCACUC1702045) was sought. The protocols were followed for the commencement of the studies.
A night before the experiments, the animals were divided into three groups (i.e. Group I, Group II and Group III) and the animals were kept on fasting. The next day, Group I received Taxol® with PTX dose equivalent to 10 mg/kg by i.v. injection via tail vein. Whereas Group II and Group III received Taxol® and PTX-SLMPs dispersed in 1 ml of 1% tween 80 with PTX dose equivalent to 10 mg/kg by i.p. injection using angiocatheter (18-gauge, 1.3 mm) respectively. At predetermined time intervals, blood samples were collected in a pre-heparinized tube till 24 h of treatment from retro orbital plexus. Plasma was separated immediately from blood samples by centrifugation at 2500 rpm for 10 min. The samples were stored at −80 °C until analysis. PTX was extracted from plasma samples by protein precipitation method using 2 ml of ethyl acetate as precipitant. The extraction was repeated three times and the organic phase was evaporated to dryness under nitrogen. The residue was reconstituted with 50 µL of mobile phase and the PTX was quantified using UPLC method described above.
The data were subjected to non-compartmental pharmacokinetics analysis using WinNonlin (version 5.1, Pharsight Corporation, Mountain View, USA) analysis. Area under curve and the area under moment curve (AUMC) were calculated using the trapezoid rule for plasma samples. The mean residence time (MRT) for plasma concentration was calculated as AUMC plasma/AUC plasma, while residence time of PTX in peritoneum was calculated as ratios of AUC peritoneum to AUC plasma.
Statistical analysis
All the experiments were carried out in triplicate and the results were expressed as mean ± standard deviation (SD). Statistical analysis of data was carried out using software Graph pad prism 5. p values smaller than 0.05 was regarded significant.
Result and discussion
Fabrication of PTX-SLMPs using CEHDA process
The schematic diagram represented in denotes the system of CEHDA platform and coaxial needle, respectively. PTX-SLMPs prepared by CEHDA process have several advantages over single axial electrospray. In single axial electrospray, when the liquid jet breaks under the influence of applied electric field, there is rapid evaporation of the solvent. The high molecular weight drug dissolved in it, has poor diffusion rate and remains on the surface of the particles. This shortcoming can be easily overcome in coaxial process [Citation37]. In CEHDA, the shell and the core solvents were injected from the outer and the inner coaxial needles respectively, resulting in better drug stability, more or complete drug encapsulation and superior control of release kinetics compared to single axial electrospray technique [Citation38]. The CEHDA process also has the ability of utilizing two different solvent and different polymers or lipids in the inner and the outer phases providing the diversity of producing microparticles with desired characteristics.
The process parameters, such as the flow rates, the applied voltage and the distance between the electrodes have played significant roles in the preparation of the microparticles. We have previously reported the different flow modes affected by the process parameters [Citation23]. Other than the process parameters, the solution properties such as viscosity, conductivity and surface tension, and the solute properties such as solubility and molecular weight of drug were also imperative in the process [Citation21,Citation39]. The concentration of solid lipid in the process plays critical role in the formation of stable coaxial liquid cone jet. Size, morphology and uniformity of SLMPs were also affected by concentration of solid lipid. Higher concentration of solid lipid not only changes the viscosity of the solvent but also results in premature solidification of the solvent at the end of coaxial needle. This solidification of lipid obstructs the flow of solvent from the needle. The lower concentration of solid lipid results in the formation of fragile particles. Therefore, the concentration has been optimized carefully for the preparation of SLMPs. We have used Pluronic F-127 as a surfactant in our formulation which can reduce the surface tension of acetone enhancing the bending instability [Citation40]. The surfactant also ensures droplet stability until stable particles formation.
In our experiment the optimum process parameters, in which we obtained the stable cone jet mode () for the preparation of highly encapsulated PTX-SLMPs, were identified [Citation41]. The influence of the flow rate and voltage was also studied and optimized. It was observed that the diameters of the particles were directly proportional to the outer flow rate and increases with the increase in flow rate (Supplementary Figure S1). However, the diameters of the particles were inversely proportional to the voltage and decreases with the increase in the voltage (Supplementary Figure 2). The process was continuously monitored by observing the particles collected on glass slide during the experiment. The PTX-SLMPs have been characterized for their particle size, PDI and zeta potential. The particle size observed using DLS was found to be 1.76 ± 0.37 µm with observed PDI 0.21 ± 0.2 µm (). The zeta potential of the particles was −19.54 ± 0.61 mV. The percentage encapsulation efficiency of PTX in PTX-SLMPs was found to be as high as 94.73%. The drug-loading efficiency was found to be 9.85 ± 2.9%. The sensitive and reproducible UPLC method was employed for the estimation of PTX in PTX-SLPMs as described above. The optimized process resulted in high encapsulation of PTX in the core with protected shells in our process.
Figure 2. The stable cone-jet mode and the PTX-SLMPs producing CEHDA process. (a) The morphology of the coaxial cone and the coaxial jet; (b) Size distribution of PTX-SLMPs; (c) Confocal fluorescence microscopic image showing the core-shell structure of the fluroescent-labelled PTX-SLMPs; (d) SEM image of PTX-SLMPs prepared by CEHDA process.
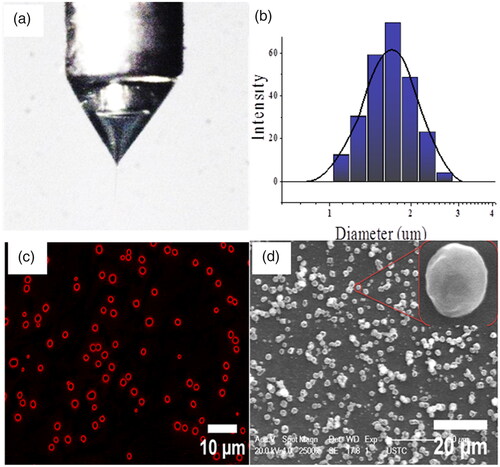
The core-shell structures of fluorescent-labelled PTX-SLMPs were evaluated and confirmed using confocal fluorescence images. The Nile red fluorescent dye added in the outer solvent during the fabrication of PTX-SLMPs was clearly visible in as a shell.
The surface morphology was further confirmed by scanning electron microscopy (SEM) as shown in . The images revealed that the PTX-SLMPs were not very spherical in morphology, but the size obtained was analogous with Dynamic Light Scattering data. The PTX-SLMPs does not have smooth surface as it was evident by the SEM. This might be attributed with the rapid evaporation of the solvent during the process, leaving behind the rigid solid particles.
In vitro dissolution profile
The CEHDA process allows the formation of PTX-SLMPs with controlled release profile, which implies that desired pre-programmed release profiles can be achieved according to the need of patient [Citation42]. The thickness of the core or shell can be adjusted according to the desired need. In vitro dissolution profile of PTX from Taxol® and PTX-SLMPs were performed using the dialysis bag method and the results have been illustrated in . represents the release of PTX within 24 h. The release medium was PBS with 1% tween 80. The saturation solubility of free PTX in the medium determined by UPLC method was found to be 0.91 ± 0.17 µg/mL. The in vitro study demonstrated that the rate of release of PTX from Taxol® was much greater than PTX-SLMPs at every time point of sampling. PTX-SLMPs release was much sustained in comparison with Taxol®. The release mechanism was studied by applying the kinetic models including zero-order rates, the first order rates, Korsmeyer and Peppas model, Higuchi model and the Hixson-Crowell cube root law revealed the best linearity with Korsmeyer and Peppas model followed by zero-order release rate. The r2 value was most eminent for Korsmeyer and Peppas model and was 0.984 for PTX-SLMPs with n value of 0.577. The result indicates the non-fickien release kinetics having combined effect of diffusion and erosion for PTX-SLMPs. The release of Taxol® was completed very quickly within 12 h having cumulative release of PTX 97 ± 2.7%, whereas PTX from PTX-SLMPs was even less than 35%. The release study has been carried out till 168 h for the complete release of PTX from PTX-SLMPs. The slow and sustained release from PTX-SLMPs may be attributed to the core-shell structure of the particles which allowed slow diffusion of PTX from the core resulting in sustained release.
In vitro cell studies
In vitro cytotoxicity on SKOV-3 ovarian cancer cells
The cytotoxicity of PTX-SLMPs was evaluated in SKOV-3 ovarian cancer cell lines by MTT assay. The results were compared with cytotoxicity caused by Taxol® at the equivalent concentration of PTX (1 nM–10 µM). The cytotoxicity results have been illustrated in . The results propose time and dose-dependent inhibition of SKOV-3 cells for both Taxol® and PTX-SLMPs. After treatment at 24 h, the cytotoxicity caused by PTX-SLMPs was not much different with Taxol®, which might be due to sustained release of PTX from PTX-SLMPs. However, at 48 h and 72 h, PTX-SLMPs were significantly potent and possess significantly high anti-proliferative activity in comparison to Taxol®. The IC50 values of Taxol® and PTX-SLMPs calculated based on probit analysis method have been illustrated in . The significant (p < .001) decrease in IC50 value suggest the higher internalization of PTX-SLMPs by the cells in comparison to Taxol®, which in turns provide higher availability of PTX inside the cells and thus the higher cytotoxic effect. The SLMPs without PTX did not show any significant toxicity against the cells and the results were aligned with control (untreated cells). It might be attributed with the non-toxic excipients that were employed in the preparation and generally recognized as safe [Citation43,Citation44]. The high encapsulation and loading efficiency of the particles was also one of the reasons for employing minimum amount of solid lipid for delivering the required dose. Previous reports have also justified the safety of lipid in cells, which have tolerate 7.5 mg/mL of solid lipid [Citation45].
Figure 4. Percentage cell viability assay of SKOV-3 cells at (a) 24 h, (b) 48 h and (c) 72 h after the treatment with Taxol® and PTX-SLMPs when analysed by MTT cell proliferation assay. *p < .05 compared to group treated with Taxol®.
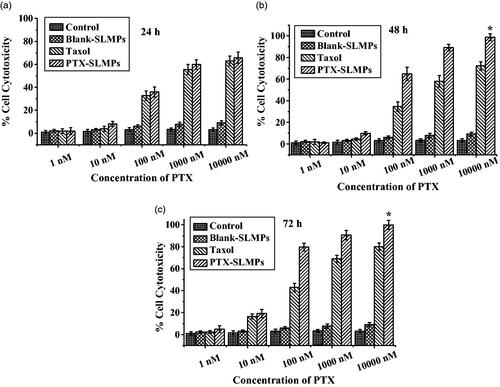
Table 1. IC50 Value of taxol® and PTX-SLMPs at (a) 24 h, (b) 48 h and (c) 72 h calculated based on MTT assay (n = 3, # p < .001 taxol®/PTX-SLMPs).
SKOV-3 ovarian cancer cell uptake by confocal microscopy and flow cytometry
The uptake of the fluorescent labelled SLMPs by SKOV-3 ovarian cancer cells in comparison with Nile red has been evaluated using both confocal microscope and flow cytometry after incubation of 4 h at equivalent concentration. The confocal images of uptake fluorescent-labelled SLMPs by cells have been shown in , which shows substantial uptake of SLMPs. Nucleus staining dye, DAPI was added to identify localization of fluorescent labelled SLMPs. The uptake studies using FACS also confirm the significantly higher uptake of fluorescent-labelled SLMPs compared to plain Nile red, which has negligible uptake. Data have been represented in . The uptake of fluorescent-labelled PTX-SLMPs was significantly (p < .05) higher, which might be attributed to the higher uptake of particles mediated by endocytosis pathways including phagocytosis and macropinocytosis [Citation46]. Contrary to nanoparticles, phagocytosis has been established as main mechanism involved for cellular uptake of microparticles [Citation47]. The rough surface of PTX-SLMPs prepared using CEHDA process might also have played an important role in higher uptake. As it has been well established that the particles with rough surface have faster cellular uptake than smooth particles or plain compounds [Citation48]. The superior efficacy of the PTX-SLMPs demonstrates the effectiveness of this approach.
Figure 5. (a) Confocal fluorescence image of single XY optical section of SKOV-3 cells. (i) Image reveals the uptake of fluorescent labelled PTX-SLMPs; (ii) Cells treated with plain Nile red at equivalent concentrations. Blue florescent signals were arising from DAPI, a nucleus staining dye. (b) Fluorescence activated cell shorter (FACS) images of SKOV-3 cells after the treatment with fluorescent-labelled PTX-SLMPs and plain Nile red. (i) Cells without treatment (control); (ii) Cells incubated with Nile red at equivalent concentrations; (iii) Cells incubated with fluorescent labelled PTX-SLMPs. (c) The mean fluorescent intensity of control (cells without treatment), plain nile red and fluorescent-labelled SLMPs. *p < .05 compared to plain nile red.
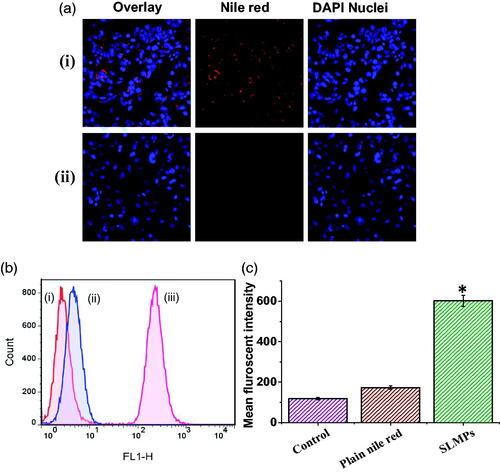
Apoptosis
The dual staining method was used to examine the potential of PTX-SLMPs in comparison with Taxol®. PTX can initiate apoptosis through multiple mechanisms including checkpoint of mitotic spindle assembly, aberrant activation of cyclin-dependent kinases [Citation49]. After analysing the treated cells with flow cytometry, results have been depicted as the dot plot in . The results demonstrated that the Taxol® and PTX-SLMPs both were efficient in inducing apoptosis after the treatment to the SKOV-3 cells; however, PTX-SLMPs have enhanced apoptotic potential. The cells undergoing early apoptosis were found to be 3.98%, whereas late apoptotic cells were 6.23% after the treatment with Taxol® and cells undergoing early apoptosis were found to be 11.2%, whereas late apoptotic cells were 10.5% after the treatment with PTX-SLMPs. Obstinate results were observed for necrosis, in which Taxol® stipulated greater necrosis effect of about 3.03% than PTX-SLNPs which showed 1.55%, which might be due to toxic excitements of Taxol®. The result indicates higher uptake of PTX-SLMPs allowed higher concentration of PTX within the cells, which resulted in its enhanced apoptogenic mechanisms [Citation49,Citation50].
In vitro pharmacokinetics
represents the plasma concentration–time profile of PTX after the i.v. and i.p. administration of Taxol® along with administration of PTX-SLMPs in wistar rats. The Taxol® was also administered by i.v. for comparative study, whereas PTX-SLMPs were only administered by i.p. to avoid any complication regarding its size. Rats were administered with 10 mg/kg of equivalent PTX dose for both i.v. and i.p. dosing. PTX-SLMPs were composed of naturally occurring lipids and hence were biocompatible, which can be easily metabolized by the metabolic enzymes present in the body including various fluids [Citation17]. Any toxicity or inflammation was also not observed during the experiment and the PTX-SLMPs were well tolerated. The pharmacokinetic parameters have been summarized in . The peak plasma concentration (Cmax: 43.83 ± 12.48 µg/mL) for i.v. administered Taxol® was recorded at the first sampling in 0.25 h. Subsequently, Taxol® was rapidly cleared from the blood suggesting that PTX from Taxol® was extensively distributed into tissues outside the plasma compartment during the clearance process [Citation51]. However, after i.p. administration, PTX-SLMPs showed enhanced resident time within the peritoneum in comparison with Taxol® which was also evident by the significant (p < .05) difference in mean residence time (MRT) of PTX from PTX-SLMPs group. This enhanced residence of PTX-SLMPs can be attributed to its size and sustain release of PTX. PTX-SLMPs have particle size of ∼ 2 µm which cannot be easily removed by lymphatic ducts, whereas smaller size cremophor micelles of Taxol® can be drained into the lymphatics. The release of PTX from Taxol® was also much high than the PTX from PTX-SLMPs as observed from the in vitro release studies. This allows a high concentration of free PTX from Taxol® to be absorbed from the peritoneum into the systemic circulation. PTX-SLMPs group exhibited Cmax 0.29 ± 0.04 µg/mL whereas Cmax 1.72 ± 0.28 µg/mL was observed for i.p. administered Taxol® group. The Tmax was shifted from 1 h of Taxol® group to 2 h of PTX-SLMPs group. This difference in Cmax and the shift in Tmax might be attributed to the amount of free PTX absorbed into the systemic circulation from Taxol®, whereas PTX-SLMPs have sustained release property and were greater in size that cannot be transported across the peritoneum [Citation52,Citation53]. The absorption into the systemic circulation occurs only after PTX will release from the PTX-SLMPs. It can be well observed from the plasma concentration profiles that sustain release of PTX from PTX-SLMPs results in the decreased systemic absorption with significant decrease in AUC in comparison to Taxol® given after IP administration [Citation54].
Table 2. Pharmacokinetic study results of taxol® administered by i.v. with taxol® and PTX-SLMPs after i.p. administration at the dose of 10 mg per kg body weight.
As PTX does not solubilize in water and have high molecular weight, it could be a preferred drug for i.p. administration. PTX encapsulation in PTX-SLMPs also results in a desired decreased systemic drug level of PTX which can minimize systemic side effects and also more amount of PTX will be available for passive targeting to the tumour. The results clearly suggest that PTX-SLMPs have enhanced the retention of PTX by sustaining its release which reached slowly into the systemic circulation compared to Taxol®.
Conclusions
The CEHDA process has unlimited potential in the field of drug delivery. It overcomes the limitation of conventional methods and assertions reproducibility with controlled particle size. Drugs having different physicochemical properties can be encapsulated with high entrapment efficiency. In our work, we have successfully developed core-shell structured PTX-SLMPs having high encapsulation efficiency and sustained release profiles. To the best of our knowledge, we are the first to report core-shell-structured SLMPs. PTX typically diffuses from the core to the shell and then to the release medium resulting in sustained release. The in vitro cytotoxicity studies demonstrated the PTX-SLMPs have great potential with higher toxicity towards SKOV-3 ovarian cancer cell lines and also induces the apoptotic pathways. The PTX-SLMPs with particle size 1–2 µm were well engulfed by the cells which enhanced killing efficiency of PTX. Our findings provide the sufficient evidence for the potential of CEHDA method in the fabrication of delivery system for PTX which can be exploited not only in ovarian cancer but also in several intra-abdominal malignancies, such as stomach, colon, pancreas and rectal cancer but further investigation of their role in the care of patients might be needed.
Supplimentary_file.docx
Download ()Acknowledgements
The authors acknowledge Prof Yucai Wang from the School of Life Science, University of Science and Technology of China for facilitating unconditionally for various cell experiments. The authors PD and MD were grateful to State Administration of Foreign Experts Affairs-Foreign Talented Youth Introduction Plan grant number WQ20180103 and WQ20180102 respectively.
Disclosure statement
No potential conflict of interest was reported by the authors.
Additional information
Funding
References
- Ovarian Cancer Overview. American Cancer Society. 2016.
- Cannistra SA. Cancer of the ovary. N Engl J Med. 2004;351:2519–2529.
- Lengyel E. Ovarian cancer development and metastasis. Am J Pathol. 2010;177:1053–1064.
- Siegel RL, Miller KD, Jemal A. Cancer Statistics, 2017. CA Cancer J Clin. 2017;67:7–30.
- du Bois A, Luck HJ, Meier W. A randomized clinical trial of cisplatin/paclitaxel versus carboplatin/paclitaxel as first-line treatment of ovarian cancer [Clinical Trial Clinical Trial, Phase III Multicenter Study Randomized Controlled Trial Research Support, Non-U.S. Gov't]. J Natl Cancer Inst. 2003;95:1320–1329.
- Metzger-Filho O, Moulin C, DʼHondt V. First-line systemic treatment of ovarian cancer: a critical review of available evidence and expectations for future directions [Review]. Curr Opin Oncol. 2010;22:513–520.
- Howell SB. Pharmacologic principles of intraperitoneal chemotherapy for the treatment of ovarian cancer. Int J Gynecol Cancer. 2008;18:20–25.
- Chan DL, Morris DL, Rao A. Intraperitoneal chemotherapy in ovarian cancer: a review of tolerance and efficacy. Cancer Manage Res. 2012;4:413–422.
- Auersperg N, Edelson MI, Mok SC, et al. The biology of ovarian cancer. Semin Oncol. 1998;25:281–304.
- Fathalla MF. Factors in the causation and incidence of ovarian cancer. Obstetrical Gynecological Survey. 1972;27:751–768.
- Landrum LM, Gold MA, Moore KN, et al. Intraperitoneal chemotherapy for patients with advanced epithelial ovarian cancer: a review of complications and completion rates. Gynecologic Oncology. 2008;108:342–347.
- Kampan NC, Madondo MT, McNally OM, et al. Paclitaxel and Its evolving role in the management of ovarian cancer. BioMed Res Int. 2015;2015:1.
- Sharma S, Verma A, Teja BV, et al. Development of stabilized Paclitaxel nanocrystals: in-vitro and in-vivo efficacy studies. Eur J Pharm Sci. 2015;69:51–60.
- Tanenbaum LM, Mantzavinou A, Subramanyam KS, et al. Ovarian cancer spheroid shrinkage following continuous exposure to cisplatin is a function of spheroid diameter. Gynecol Oncol. 2017;146:161–169.
- Sun J, Yin M, Zhu S, et al. Ultrasound-mediated destruction of oxygen and paclitaxel loaded lipid microbubbles for combination therapy in hypoxic ovarian cancer cells. Ultrasonics Sonochemistry. 2016;28:319–326.
- Liu L, Chang S, Sun J, et al. Ultrasound-mediated destruction of paclitaxel and oxygen loaded lipid microbubbles for combination therapy in ovarian cancer xenografts. Cancer Lett. 2015;361:147–154.
- Scalia S, Young PM, Traini D. Solid lipid microparticles as an approach to drug delivery. Expert Opin Drug Deliv. 2015;12:583–599.
- Dwivedi P, Khatik R, Khandelwal K, et al. Pharmacokinetics study of arteether loaded solid lipid nanoparticles: an improved oral bioavailability in rats. Int J Pharm 2014;466:321–327.
- Pilaniya U, Pilaniya K, Chandrawanshi HK, et al. Formulation and evaluation of verapamil hydrochloride loaded solid lipid microparticles. Pharmazie. 2011;66:24–30.
- Passerini N, Qi S, Albertini B, et al. Solid lipid microparticles produced by spray congealing: influence of the atomizer on microparticle characteristics and mathematical modeling of the drug release. J Pharm Sci. 2010;99:916–931.
- Bussano R, Chirio D, Costa L, et al. Preparation and characterization of insulin-loaded lipid-based microspheres generated by electrospray. J Dispers Sci Technol. 2011;32:1524–1530.
- Ding L, Lee T, Wang C-H. Fabrication of monodispersed Taxol-loaded particles using electrohydrodynamic atomization. J Control Release. 2005;102:395–413.
- Yuan S, Lei F, Liu Z, et al. Coaxial electrospray of curcumin-loaded microparticles for sustained drug release. PLoS One. 2015;10:e0132609.
- Zhang L, Huang J, Si T, et al. Coaxial electrospray of microparticles and nanoparticles for biomedical applications. Expert Rev Med Devices. 2012;9:595–612.
- Chen J, Cui Y, Xu X, et al. Direct and effective preparation of core-shell PCL/PEG nanoparticles based on shell insertion strategy by using coaxial electrospray. Colloids Surf A Physicochem Eng Asp. 2018;547:1–7.
- Matsuura T, Maruyama T. Calcium phosphate-polymer hybrid microparticles having functionalized surfaces prepared by a coaxially electrospray technique. Colloids Surf A Physicochem Eng Asp. 2017;526:64–69.
- Soares RMD, Siqueira NM, Prabhakaram MP, et al. Electrospinning and electrospray of bio-based and natural polymers for biomaterials development. Mater Sci Eng: C. 2018;92:969–982.
- Cao L, Luo J, Tu K, et al. Generation of nano-sized core–shell particles using a coaxial tri-capillary electrospray-template removal method. Colloids Surf B: Biointerfaces. 2014;115:212–218.
- Mai Z, Chen J, He T, et al. Electrospray biodegradable microcapsules loaded with curcumin for drug delivery systems with high bioactivity [10.1039/C6RA25314H]. RSC Adv. 2017;7:1724–1734.
- Gao Y, Zhao D, Chang M-W, et al. Optimising the shell thickness-to-radius ratio for the fabrication of oil-encapsulated polymeric microspheres. Chem Eng J. 2016; 284:963–971.
- Zhang C, Yao Z-C, Ding Q, et al. Tri-needle coaxial electrospray engineering of magnetic polymer yolk–shell particles possessing dual-imaging modality, multiagent compartments, and trigger release potential. ACS Appl Mater Interfaces. 2017;9:21485–21495.
- Xing Z, Zhang C, Zhao C, et al. Targeting oxidative stress using tri-needle electrospray engineered Ganoderma lucidum polysaccharide-loaded porous yolk-shell particles. Eur J Pharm Sci. 2018;125:64–73.
- Almeria B, Fahmy TM, Gomez A. A multiplexed electrospray process for single-step synthesis of stabilized polymer particles for drug delivery. J Control Release. 2011;154:203–210.
- Mangrio FA, Dwivedi P, Han S, et al. Characteristics of artemether-loaded poly(lactic-co-glycolic) acid microparticles fabricated by coaxial electrospray: validation of enhanced encapsulation efficiency and bioavailability. Mol Pharma. 2017;14: 4725–4733.
- Alvi KA, Wang S, Tous G. New and rapid ultra‐performance liquid chromatography assay of paclitaxel. J Liq Chromatogr Relate Technol. 2008;31:941–949.
- Dwivedi P, Kansal S, Sharma M, et al. Exploiting 4-sulphate N-acetyl galactosamine decorated gelatin nanoparticles for effective targeting to professional phagocytes in vitro and in vivo. J Drug Target. 2012;20:883–896.
- Davoodi P, Feng F, Xu Q, et al. Coaxial electrohydrodynamic atomization: microparticles for drug delivery applications. J Control Release. 2015;205:70–82.
- Chakraborty S, Liao IC, Adler A, et al. Electrohydrodynamics: A facile technique to fabricate drug delivery systems. Adv Drug Deliv Rev. 2009;61:1043–1054.
- Luo CJ, Edirisinghe M. Core-liquid-induced transition from coaxial electrospray to electrospinning of low-viscosity poly(lactide-co-glycolide) sheath solution. Macromolecules. 2014;47:7930–7938.
- Dai WG, Dong LC. Characterization of physiochemical and biological properties of an insulin/lauryl sulfate complex formed by hydrophobic ion pairing. Int J Pharm. 2007;336:58–66.
- Nie H, Dong Z, Arifin DY, et al. Core/shell microspheres via coaxial electrohydrodynamic atomization for sequential and parallel release of drugs. J Biomed Mater Res A. 2010;95:709–716.
- Singh MN, Hemant KSY, Ram M, et al. Microencapsulation: a promising technique for controlled drug delivery. Res Pharm Sci. 2010;5:65–77.
- Kumar S, Randhawa JK. Solid lipid nanoparticles of stearic acid for the drug delivery of paliperidone [10.1039/C5RA10642G]. RSC Adv. 2015;5:68743–68750.
- Doktorovová S, Kovačević AB, Garcia ML, et al. Preclinical safety of solid lipid nanoparticles and nanostructured lipid carriers: current evidence from in vitro and in vivo evaluation. Eur J Pharm Biopharm. 2016;108:235–252.
- Soddu E, Rassu G, Cossu M, et al. The effect of formulative parameters on the size and physical stability of SLN based on “green” components. Pharm Dev Technol. 2016;21:98–107.
- Kou L, Sun J, Zhai Y, et al. The endocytosis and intracellular fate of nanomedicines: implication for rational design. Asian J Pharm Sci. 2013;8:1–10.
- Lawlor C, Kelly C, O’Leary S, et al. Cellular targeting and trafficking of drug delivery systems for the prevention and treatment of MTb. Tuberculosis. 2011;91:93–97.
- Hu X, Hu J, Tian J, et al. Polyprodrug amphiphiles: hierarchical assemblies for shape-regulated cellular internalization, trafficking, and drug delivery. J Am Chem Soc. 2013;135:17617–17629.
- Wang TH, Wang HS, Soong YK. Paclitaxel-induced cell death: where the cell cycle and apoptosis come together. Cancer. 2000;88:2619–2628.
- Das GC, Holiday D, Gallardo R, et al. Taxol-induced cell cycle arrest and apoptosis: dose-response relationship in lung cancer cells of different wild-type p53 status and under isogenic condition. Cancer Lett. 2001;165:147–153.
- Peltier S, Oger JM, Lagarce F, et al. Enhanced oral paclitaxel bioavailability after administration of paclitaxel-loaded lipid nanocapsules. Pharm Res. 2006;23:1243–1250.
- Tsai M, Lu Z, Wang J, et al. Effects of carrier on disposition and antitumor activity of intraperitoneal paclitaxel. Pharm Res. 2007;24:1691–1701.
- Dwivedi P, Yuan S, Han S, et al. Core-shell microencapsulation of curcumin in PLGA microparticles: programmed for application in ovarian cancer therapy. Artif Cells Nanomed Biotechnol. 2018;9:1–11.
- Fu Q, Hargrove D, Lu X. Improving paclitaxel pharmacokinetics by using tumor-specific mesoporous silica nanoparticles with intraperitoneal delivery. Nanomedicine. 2016;12:1951–1959.