Abstract
Graphene and its derivatives, graphene oxide (GO) and graphene oxide quantum dots (GOQDs), have recently attracted much attention as bioactive factors in differentiating stem cells towards osteoblastic lineage. The stem cells from human exfoliated deciduous teeth (SHEDs) possess the properties of self-renewal, extensive proliferation, and multiple differentiation potential, and have gradually become one of the most promising mesenchymal stem cells (MSCs) in bone tissue engineering. The purpose of this study was to explore the effects of GO and GOQDs on the osteogenic differentiation of SHEDs. In this study, GO and GOQDs facilitated SHED proliferation up to 7 days in vitro at the concentration of 1 μg/ml. Because of their excellent fluorescent properties, GOQD uptake by SHEDs was confirmed and distributed in the SHED cytoplasm. Calcium nodules formation, alkaline phosphatase (ALP) activity, and RNA and protein expression increased significantly in SHEDs treated with osteogenic induction medium containing GOQDs but decreased with osteogenic induction medium containing GO. Interestingly, the Wnt/β-catenin signaling pathway appeared to be involved in osteogenic differentiation of SHEDs induced with GOQDs. In summary, GO and GOQDs at the concentration of 1 μg/ml promoted SHED proliferation. GOQDs induced the osteogenic differentiation of SHEDs, whilst GO slightly inhibited it.
Introduction
Bone defects seriously affect the physical and psychological well-being of patients. In the repair of bone defects, targeting bone regeneration in the sites is a research highlight in bone tissue engineering [Citation1–3].
Bone tissue engineering consists of stem cells [Citation2], bioactive factors [Citation1], and scaffolds [Citation3,Citation4]. According to numerous researchers, the stem cells from human exfoliated deciduous teeth (SHEDs) possess the properties of self-renewal, extensive proliferation, and multiple differentiation potential and conform to ethical concerns, thus gradually becoming one of the most promising mesenchymal stem cells (MSCs) in bone tissue engineering [Citation5–7]. In fact, not only can SHEDs be isolated from primary teeth that are routinely extracted in childhood and generally discarded without any ethical concerns, but also they can be isolated from deciduous caries-affected teeth [Citation7,Citation8]. The main advantages of using SHEDs are that they can be easily obtained and present higher proliferation rates and higher levels of osteocalcin production than dental pulp stem cells (DPSCs) during osteogenic differentiation [Citation9–11]. Numerous studies [Citation12–14] have reported that SHEDs were capable of osteogenic differentiation both in vitro and in vivo. Osteogenic-related RNA and protein expression increased when SHEDs were treated with osteogenic induction medium in vivo [Citation12]. Additionally, SHEDs effectively induced robust bone formation in vivo when they were transplanted into immunocompromised mice [Citation13] or swine [Citation14].
Graphene, a typical sp2 carbon-based material, has drawn attention since 2004 [Citation15]. Due to its potential as a delivery protein or drug molecules [Citation16,Citation17], antibacterial agent [Citation18], or cancer-targeting therapy [Citation19], graphene and its derivative, graphene oxide (GO), have recently attracted attention in the biomedical field. GO is a single-atom-thick and two-dimensional carbon material prepared by the oxidation of graphite from a highly oxidized form of graphite [Citation20]. Moreover, GO has many excellent properties, such as large specific surface area, amphipathy and rich oxygen-containing functional groups [Citation20]. GO, the amphiphilic compound, has abundant functional groups, such as hydroxyl and epoxy groups on the planes and carboxylic acid groups at the edges [Citation20,Citation21], which enhances its interactions with molecules and proteins in osteogenic induction medium through hydrophobic and electrostatic interactions [Citation22], then potentially enhance stem cell osteogenic differentiation [Citation23–25]. Published works have suggested that GO has good biocompatibility. Rosa V et al. showed that DPSCs were anchored to the rough surface provided by GO, as assessed by scanning electron microscopy (SEM) [Citation26]. Moreover, GO at the concentration of 0.1 μg/ml allowed for BMSC attachment and promoted proliferation, whilst GO at 10 μg/ml was cytotoxic [Citation27]. In a review by Mohammadrezaei D et al., up to 50 μg/ml for GO seems to be safest for most cell lines [Citation25]. In addition, GO could induce BMSC and DPSC osteogenic differentiation in vitro [Citation26,Citation27]. GO-coated β-tricalcium phosphate scaffold increased the rate of new bone formation in vivo [Citation28]. Hence, dental materials and bone tissue engineering materials may benefit from these appealing properties of GO.
Recently, nanomaterials, another promising material, have attracted an increasing number of studies focusing on their relationship with MSCs. Nanoparticles can be ingested by cells and then influence cell differentiation. Yi C et al. [Citation29] showed that gold nanoparticles (AuNPs) exhibited a positive influence on MSC osteogenic differentiation by interacting with the cell membrane and binding with proteins in the cytoplasm, whilst Liu D et al. [Citation30] demonstrated that carbon nanotubes (CNTs) inhibited MSC osteogenic differentiation. Moreover, a new type of carbon-based quantum dots, graphene oxide quantum dots (GOQDs), has attracted increasing attention due to their quantum confinement and edge effects [Citation31]. As zero-dimensional stable materials converted from two-dimensional GO sheets, GOQDs not only exhibit the excellent properties of GO but also other new properties, such as optimal stability and good photoluminescence performance [Citation31,Citation32]. Unlike the ability of GO to absorb small molecules and some proteins from an osteogenic induction medium, which further proved its bioactivity, GOQDs can be ingested by cells and then influence cell behaviour [Citation33]. Actually, most reports about GOQDs have been focused on their biomedical applications, for instance, biosensing [Citation34], bioimaging [Citation35], drug delivery [Citation36] and photodynamic therapy [Citation37]. Meanwhile, correlative research found graphene quantum dots’ (GOQs) uptake by MSCs and effectively induced MSC osteogenic differentiation [Citation38]. However, until now, there have been no studies about the interaction between GOQDs and SHEDs.
It is essential to explore better materials for surface modification of scaffolds and guided bone regeneration in bone tissue engineering. As shown previously [Citation26,Citation27,Citation33,Citation34,Citation36,Citation39], both GO and GOQDs have good biocompatibility and the potential of differentiating stem cells towards osteoblastic lineage. However, whether GO or GOQDs could promote SHED osteogenic differentiation, and the different abilities of GO and GOQDs in inducing osteogenic differentiation of SHEDs remain unknown.
In this study, we aimed to explore the effects of GO and GOQDs on the osteogenic differentiation of SHEDs. Initially, we evaluated the proliferation of SHEDs incubated with GO or GOQDs. Then, mineralization, ALP activity, osteogenic-related RNA and proteins were investigated for assessment of the ability of GO and GOQDs in inducing SHED osteogenic differentiation. The hypothesis was that 1 μg/ml GO and GOQDs could promote SHEDs proliferation and that GOQDs would have better ability than GO in inducing SHED osteogenic differentiation.
Materials and methods
Preparation and characterization of the GO and GOQDs
GO was prepared according to the modified Hummer’s method [Citation40]. In detail, 180 ml H2SO4 and 20 ml H3PO4 were mixed, then 2 g of graphite and 9 g of KMnO4 were subsequently added to the mix. The suspension was stirred at 50 °C in a thermostatic water bath for 2 h whilst 40 ml of deionized water was added. After the suspension was cooled to room temperature, it was centrifuged to yield solids. Next, the solids were dissolved in deionized water whilst 4 ml of H2O2 were added. Finally, the suspension was centrifuged, washed with deionized water and hydrochloric acid, and freeze-dried to yield GO.
GOQDs were purchased from Nanjing XFNANO Materials Tech Co., Ltd (Nanjing, China). GOQDs with the concentration of 1 mg/ml were sterilized through a 0.22 μm filter membrane (EMD Millipore, Billerica, MA, USA).
The compositions of GO were characterized by a Laser Micro-Raman Spectrometer (Renishaw InVia, Gloucestershire, UK) with 532 nm laser excitation and Fourier transformation infra-red spectrometry (FTIR; Bruker, Karlsruhe, Germany).
The morphology of GO and GOQDs was characterized by transmission electron microscopy (TEM; FEI Tecnai G2 Spirit, Hillsboro, OR, USA) at an acceleration voltage of 300 KV.
Isolation and characterization of SHEDs
The cells from human exfoliated deciduous teeth were taken from 6- to 10-year-old children whose caries-free deciduous teeth required extraction. Ethics Committee approval was provided by the School of Stomatology, Sun Yat-sen University. Briefly, the teeth were placed in pre-cooled phosphate-buffered saline (PBS, Hyclone, Logan, UT, USA) with 1% penicillin/streptomycin (P/S; Gibco, Thermo Fisher Scientific, Inc., GrandIsland, NY, USA ) and taken to the laboratory within 8 h of extraction. Pulp tissue was extracted with a barbed broach, cut into pieces and digested with 1:1 3 g/l collagenase type I (Sigma-Aldrich, St. Louis, MO, USA) and 4 g/l dispase (Roche, Basel, Switzerland) at 37 °C for 30 min. The pulp cells were then suspended in the primary culture medium, which included Dulbecco’s modified Eagle’s medium (DMEM; Gibco), 20% foetal bovine serum (FBS; Gibco), and 2% penicillin/streptomycin, then cultured in 37 °C and 5% CO2 incubator.
Morphology of primary SHEDs and third-passage (P3) SHEDs was observed by an inverted microscope (Zeiss, Oberkochen, Germany). SHEDs were characterized by flow cytometric analysis for CD34, CD44, CD45, CD73, CD90, and CD105 (BD Pharmingen, San Diego, CA, USA). Flow cytometry was performed with a Beckman Coulter CytoFlex system (Beckman Coulter, Fullerton, CA, USA).
Adipogenic induction medium [DMEM with 10% FBS, 1% P/S, 1 μM dexamethasone (Sigma), 0.2 mM indomethacin (Sigma-Aldrich), 10 μg/ml insulin (Sigma-Aldrich), and 0.5 mM methylisobutylzanthine (Sigma-Aldrich)] was applied to induce the adipogenic differentiation of SHEDs. Twenty-one days later, the cells were fixed with 4% paraformaldehyde for 30 min at room temperature and washed with PBS, after which Oil red O solution was added and incubated for 15 min and then washed thoroughly with PBS for evaluation of the formation of lipid droplets.
SHEDs were passaged for 3 times, and each passage was determined when cells reached 80–90% confluence. Only the third and fourth passages of SHEDs were used for the in vitro experiments. SHEDs were cultured in the culture medium that included DMEM, 10% FBS and 1% P/S.
Proliferation assay
SHEDs were plated on 96-well plates at a concentration of 5 × 103 cells/well. GO (1 and 10 μg/ml) or GOQDs (1 and 10 μg/ml) were added and supplied with culture medium. The control group was placed in SHED-treated culture medium. SHED proliferation was tested by means of Cell Counting Kit-8 (CCK-8; Beyotime Institute of Biotechnology, Haimen, China). After the indicated time points for 1, 3, 5, and 7 days of incubation, the culture medium of each well was replaced with 10 μl of CCK-8 solution and 100 μl DMEM, after which the plates were incubated for 1 h at 37 °C in 5% CO2. The OD value of each well was measured by means of a microplate reader (Tecan, Männedorf, Switzerland) at a wavelength of 450 nm.
Confocal laser scanning microscopy (Zeiss) was used to observe the morphology of SHEDs treated with GO or GOQDs. Initially, SHEDs were plated on a laser-scanning confocal petri dish at a concentration of 1 × 104 cells/dish, after SHEDs were attached, then treated with culture-medium-coated GO or GOQDs at the concentration of 1 μg/ml. After 48 h, SHEDs were washed three times with PBS and then imaged.
Alizarin red staining
After cell adhesion, SHEDs were seeded in 6-well plates at a density of 2 × 105 per well, then treated with GO/osteogenic induction medium (OIM) or GOQDs/OIM for 14 days. Thereafter, DMEM with 10% FBS, 1% P/S, 1 μM dexamethasone (Sigma), 10 mM β-glycerophosphate (Sigma-Aldrich) and 50 μM ascorbic acid (Sigma-Aldrich) was denoted as OIM. OIM containing GO or GOQDs was respectively, denoted as GO/OIM or GOQDs/OIM. The control group was SHEDs treated with OIM. Cells were fixed with 4% paraformaldehyde for 30 min at room temperature and washed with PBS, after which they were stained with Alizarin red (Cyagen Biosciences Inc., Guangzhou, China) for 30 min. An inverted microscope (Zeiss) was used to take the microscopic images.
ALP activity assay
After cell adhesion, SHEDs were seeded in 24-well plates at a density of 5 × 104 per well, and the culture medium was placed with OIM including 1 μg/ml GO or GOQDs for 3 and 7 days. The control group was SHEDs treated with OIM. SHEDs were subjected to alkaline phosphatase (ALP) activity assay by means of the ALP assay kit (Nanjing Jiancheng Bioengineering Institute, Nanjing, China) according to the manufacturer’s instructions.
Quantitative real-time reverse transcription polymerase-chain-reaction (qRT-PCR)
After cell adhesion, SHEDs were seeded in 6-well plates at a density of 2 × 105 per well, then treated with GO/OIM or GOQDs/OIM for 14 days. The control group was SHEDs treated with OIM. Total RNA was extracted by Trizol (Invitrogen , Thermo Fisher Scientific, Inc., Waltham, MA, USA), and the concentration was determined by spectrophotometry. cDNA was prepared through reverse transcription (TaKaRa Bio Inc, Shiga, Japan). Gene expression was quantified by qPCR with an SYBR Green kit (Roche, Basel, Switzerland) and gene-specific primers. The primers were synthesized by the Beijing Genomics Institute, China. qRT-PCR primer sequences are shown in . GAPDH was set as the internal control, after which the qRT-PCR, OCN, Runx2, COL I, and β-catenin RNA levels were calculated and compared among the different groups.
Table 1. Primer sequences used in qRT-PCR.
Western blotting
After cell adhesion, SHEDs were seeded in 6-well plates at a density of 2 × 105 per well, then treated with GO/OIM or GOQDs/OIM for 14 days. The control group was SHEDs treated with OIM. Cells underwent lysis in RIPA buffer (KeyGen BioTECH, Nanjing, China) containing 1% protease inhibitor cocktail (CWBIO, Beijing, China) and protein concentration were measured by means of the BCA assay kit (CWBIO). A 40 μg quantity of protein was applied to each lane, separated on Tris-Glycine SDS-PAGE (CWBIO, Beijing, China ), and then transferred to a polyvinylidene difluoride membrane (EMD Millipore, Billerica, MA, USA). Subsequently, the membranes were blocked for 1 h at room temperature by incubation in TBST (10 mM TrisHCl, 50 mM NaCl, 0.25% Tween 20) containing 5% nonfat milk, then incubated with the primary antibodies against osteocalcin (OCN; 1:500, Abcam, Cambridge, MA, USA), runt-related transcription factor-2 (Runx2; 1:1000, Cell Signaling Technology, CST, Danvers, MA, USA), collagen I (COL I; 1:500, Abcam), β-catenin (1:1000; CST), and GAPDH (1:1000; CST) for 18 h at 4 °C. Subsequently, after 1 h of incubation with secondary antibody (1:2000; CST) and analysis with an enhanced chemiluminescent (ECL) detection system (EMD Millipore).
Statistical analysis
All data were expressed as the mean ± standard deviation (mean ± SD), of at least, triplicate determinations. The SPSS 20.0 software package was used for statistical analysis of the data. Results were performed by one-way analysis of variance (ANOVA). Tukey's multiple comparisons test was used for comparison among groups. p < .05 was considered statistically significant.
Results
Characterization of GO and GOQDs
From the Raman spectrum [(a)] of GO, the G and D bands were found to be the most prominent, with the G band (∼1605 cm−1) an ordered sp2 structure, whereas the D (∼1359 cm−1) band was associated with the defects and amorphous structures on the graphene edge. The ID/IG ratio (0.85) exhibited the typical response of GO.
Figure 1. Characterization of GO and GOQDs. (A) Characterization of GO with (a) laser Micro-Raman Spectrometer (b) FTIR spectrometer. (B) Morphology of (a) GO (b) GOQDs with TEM.
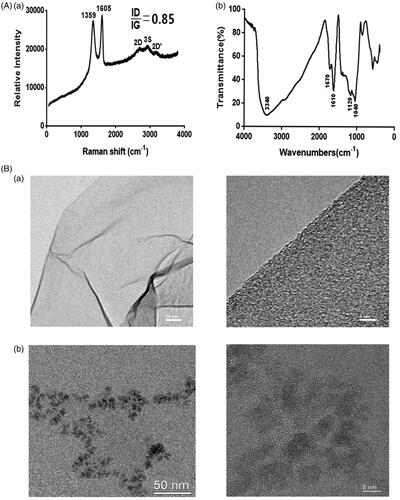
The FTIR of GO is shown in (b). As for GO, the absorption band at 3340 cm−1 was observed due to –OH stretching, 1670 cm−1 (stretching vibrations of C=O), 1610 cm−1 (hydroxyl stretching), and in the region of 1040–1120 cm−1 due to C–O–C and C–O stretching vibrations. Thus, the GO which we prepared showed hydrophilicity.
The morphology of GO and GOQDs was observed by TEM []. The morphology of GO showed two-dimensional irregularly shaped flakes with lateral size over 50 nm whereas GOQDs showed dot sizes with a lateral dimension of less than 10 nm.
Characterization of SHEDs
The major morphology of SHEDs was typically spindle-like or fibroblast-like []. As for the analysis of cell-surface markers, 98.4% of the cells were CD44-positive, 96.1% were CD73-positive, 96.8% were CD90-positive, and 95.4% were CD105-positive, only 0.42% of the cells were CD34-positive and 0.32% were CD45-positive []. This indicated that cells were positive for the expression of mesenchymal stem-cells-related markers and negative for the expression of hematopoietic markers. In addition, after the induction of adipogenic and osteogenic differentiation of SHEDs, clear, distinct calcium deposits and lipid droplets were observed []. The results demonstrated that cells from human exfoliated deciduous teeth were mesenchymal stem cells, which had multiple differentiation potential.
Figure 2. Characterization of SHEDs. (A) (a) 4 days of primary SHEDs (b) 3 days of SHEDs at P3, ×50. (B) Flow cytometry analysis of cell surface marker CD34, CD45, CD44, CD73, CD90, CD105. (C) (a) Cells were treated with osteogenic induction medium after 14 days and stained with Alizarin red, ×50. (b) Cells were treated with adipogenic induction medium after 21 days and stained with oil red O, ×50.
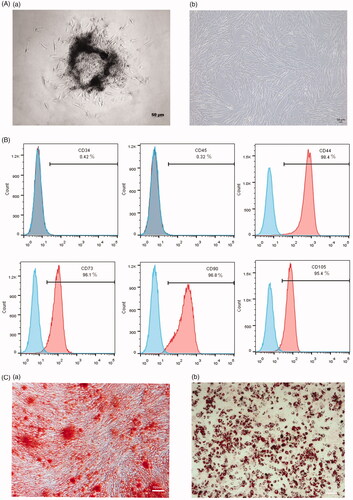
Proliferation of SHEDs
To choose a material with low cytotoxicity, we investigated the proliferation ability of SHEDs by a CCK-8 assay. From the results (), GO and GOQDs showed low cytotoxicity at the concentrations of 1 μg/ml and 10 μg/ml. Moreover, 1 μg/ml GO and GOQDs significantly promoted the proliferation of SHEDs, so we chose 1 μg/ml of GO and GOQDs in the subsequent assays.
Figure 3. The proliferation of SHEDs incubated with different concentrations (1 and 10 ug/mL) of GO and GOQDs after 1, 3, 5, 7 days. *p < .05.
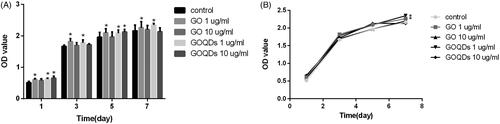
Confocal laser scanning microscopy was used to show the morphology of SHEDs cultured with GO or GOQDs. As shown in , over a period of 48 h, SHEDs exhibited normal morphology in both the GO and GOQD culture media compared with the control cells. Interestingly, after incubation for 48 h, the cells treated with GOQDs emitted blue fluorescence under 405 nm excitation wavelength, whilst there was no fluorescence from the cells of the control group and the cells treated with GO. This indicated that GOQDs can easily penetrate the SHED membrane and enter the cells distributed in the cytoplasm, which then might influence the function and behaviour of SHEDs.
Mineralized matrix nodule formation
The results of Alizarin red S staining after 14 days of osteogenic-induced culturing are shown in . Obviously, there was calcium nodule formation in the three groups with a higher level of mineralization in the GOQDs/OIM group.
Figure 5. SHEDs were cultured in osteogenic induction medium containing GO or GOQDs. (A) Osteogenic differentiation was detected by ALP activity assay kit after 3 and 7 days. (B) Osteogenic differentiation was detected by Alizarin red S staining for 14 days. (a) Optical images (b) Microscopic images, ×50. *p < .05, **p < .01, ***p < .001, ****p < .0001.
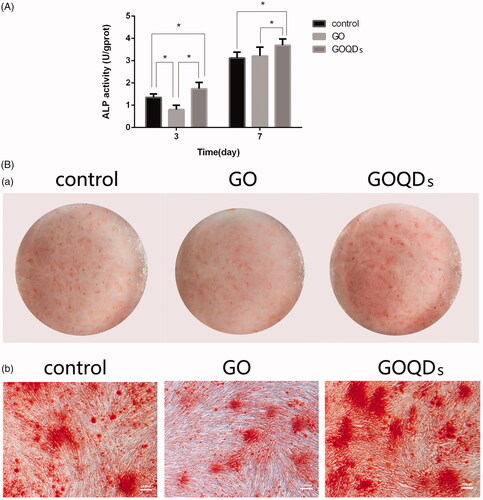
ALP activity
ALP activity is showed in . During the 7 days, GOQDs/OIM showed a stronger SHED-induced osteogenic differentiation ability, as compared with the control group, in which ALP activity increased significantly, whilst during the first 3 days, compared with the control group, GO/OIM significantly inhibited the osteogenic differentiation of SHEDs. However, five days later, there were no significant differences in ALP activity between the GO/OIM group and the control group, indicating that GO could neither affect the ALP generation nor further induce osteogenic differentiation in the early days.
qRT-PCR analysis of osteogenic-related mRNA
To investigate the osteogenic differentiation of SHEDs induced by GO or GOQDs, we studied osteogenic-related gene expression. Moreover, we analyzed Wnt signaling pathway-related RNA. From the results shown in , the expression of OCN, Runx2, COL I, and β-catenin was distinctly higher in the GOQDs/OIM group than in the control group after both 7 and 14 days of osteogenic-induced culturing. However, the expression of those RNAs was lower in the GO/OIM group than in the control group, but had no statistically significant difference.
Western blot analysis of osteogenic-related protein
In addition to assessing the ability of GO and GOQDs to induce SHED osteogenic differentiation, we analyzed the protein expression after 14 days of differentiated cells. Notably, the expression of OCN, Runx2 and COL I was distinctly higher in the GOQDs/OIM group as compared with the control group, whilst the expression of those proteins was lower in the GO/OIM group. In addition, the results showed that the expression of β-catenin was the same as that of the osteogenic-related protein ().
Discussion
In recent years, the exploration of promising materials for surface modification of scaffolds or guided bone regeneration has become a major research focus in the field of bone tissue engineering. In this study, we proposed to gain new insights by studying the cellular behaviours of SHEDs towards GO and GOQDs to explore better biomaterials to induce stem cell osteogenic differentiation.
GO can be obtained by chemical exfoliation of graphite, which introduces oxygen functional groups [Citation20,Citation40]. The compositions of GO were characterized by Raman spectroscopy []. The D peak was due to the defects and amorphous structures on the graphene edge, whilst the G peak was an ordered sp2 structure [Citation41]. Moreover, the 0.85 ID/IG ratio is consistent with the values reported in the literature for graphene oxide [Citation42]. The FTIR of GO results [ (b)] suggests that graphite became part of GO containing oxygen-containing functional groups, such as –OH, C–O, C = O, and C–O–C, so that GO can readily form colloidal suspensions in triple-distilled water. The hydrophilicity of GO could connect with many osteogenic-related factors that may influence stem cell differentiation [Citation21–23]. The TEM images [] showed that GO and GOQDs contained a good crystal graphene structure and that GO showed two-dimensional irregularly shaped flakes with lateral size over 50 nm, whereas GOQDs showed dots with sizes under 10 nm. Different sizes might have different effects of GO and GOQDs on the osteogenesis of SHEDs.
Currently, autologous stem cell transplantation [Citation43], a new regenerative treatment in bone tissue engineering techniques, requires promising therapeutic stem cells. SHEDs, compared with BMSCs and DPSCs, possess the properties of being easily obtained, harmless to the human body, having a low risk of immune rejection and cross-infection, and having higher osteogenic and neurogenic differentiation capacity [Citation11,Citation44–46]. Further, they have been recognized as promising stem cells for regenerative medicine. In this study, we successfully separated and cultured SHEDs and observed their proliferation and differentiation towards GO and GOQDs.
SHEDs were capable of proliferating and maintaining normal cell morphology on GO and GOQDs ( and ). As shown in , GO and GOQDs at the concentration of up to 10 μg/ml had no effect on cell viability. As for the proliferation of SHEDs, cells cultured with 1 μg/ml of GO or GOQDs were significantly more numerous after 7 days compared with the control group. These results indicated that 1 μg/ml GO and GOQDs was beneficial for proliferation, because SHED growth was improved. However, there were some differences from early work, Wei C et al. [Citation27] demonstrated that 1 μg/ml of GO could not promote BMSC proliferation as compared with the control group, possibly because the SHEDs had a higher proliferative capability than BMSCs.
Moreover, the photoluminescence performance of GOQDs allowed for their uptake by SHEDs, being monitored with fluorescence microscopy. As shown in , after SHEDs were incubated with GOQDs for 48 h, the cells were labeled in blue, whilst SHEDs incubated with GO or in the absence of both did not show any fluorescence. It was shown that GOQDs could distribute homogeneously throughout the cell body whilst GO could not. Nanoparticles could be ingested by cells and then influence cell differentiation.
Next, we used Alizarin red S staining to evaluate the mineralized nodules formed by osteoblasts derived from SHEDs. As shown in , the mineralized matrix nodule formation was increased after GOQDs were added to the OIM whilst it was decreased in the GO/OIM group. However, it requires confirmation whether more cells enhanced mineralization in the GOQDs/OIM group or GOQDs indeed upregulate the osteogenic-related RNA and proteins and then promoted mineralized matrix formation. The ALP activity assay was used to evaluate the impact of GO and GOQDs exposure on the differentiation of SHEDs. ALP is a phenotypic marker for the early osteogenic differentiation stage [Citation47]. As shown in , the ALP activity of SHEDs cultured in GO/OIM or GOQDs/OIM increased from day 3 to day 7. Moreover, there was a significant difference between the two biomaterials whereby ALP activity increased significantly in the GOQDs/OIM group compared with the GO/OIM group, suggesting that SHEDs incubated in GOQDs might have greater osteogenic ability than GO. However, this finding differed from those of early established studies (Rosa V et al. [Citation26] and Wei C et al. [Citation27]), which reported that GO could induce MSC and BMSC osteogenic differentiation. Some reasons that might cause the differences in GO characterization include concentration [Citation27], synthetic method [Citation26] and oxidation state [Citation25,Citation48]. In this study, because of the differences in synthesis, the ID/IG ratio of GO we prepared was 0.85, which was lower than that of the micro-sized GO published by Kang et al. [Citation49], indicating different degrees of oxidation that may cause the differences in ability to induce osteogenic differentiation of stem cells. Moreover, Wei C et al. [Citation27] reported that 0.1 μg/mL GO promoted BMSC osteogenesis. However, in this study, we used 1 μg/ml GO, which was a higher concentration, to induce SHED osteogenic differentiation. The difference of concentrations might cause different results.
To further confirm this conclusion, we studied several gene expressions. As we know, Runx2 is a key transcription factor regulating genes that encode for proteins involved in the osteogenic differentiation and bone formation [Citation50,Citation51]. In addition, bone extracellular matrix proteins such as OCN and specific collagen such as COL I, which is synthesized and secreted by osteoblasts, play an essential role in the mineralization of bone tissue [Citation51,Citation52]. Usually, COL I is a marker expressed in early stages of osteoblastic differentiation, whilst Runx2 and OCN are markers expressed in late stages [Citation50–53]. According to the results shown in , the aforementioned conclusion—that, compared with GO, GOQDs significantly improved the osteogenic differentiation of SHEDs — was confirmed. The expression of OCN, Runx2 and COL I RNA in SHEDs cultured with GOQDs significantly increased on day 7 and day 14, especially Runx2 RNA on day 7, whilst those RNAs in SHEDs cultured with GO slightly decreased on day 7 and day 14 but without a statistically significant difference, expect OCN RNA on day 7. Interestingly, the expression of β-catenin RNA, one of the key components of the Wnt/β-catenin signaling pathway, was up-regulated after SHEDs were treated with GOQDs. It is usually known that the Wnt/β-catenin pathway plays an important role in homeostasis and osteogenesis [Citation54,Citation55]. Jiang S et al. [Citation56] reported that SHEDs could differentiate into osteoblasts and odontoblasts and suppress the adipogenic differentiation through Wnt signaling pathways. Results from Western blot were in agreement with the results from qRT-PCR. The protein expressions of OCN, Runx2, COL I and β-catenin were distinctly higher in the GOQDs/OIM group as compared with the GO/OIM group. It seems that GOQDs up-regulated the expression of bone formation RNA and protein and appeared to be related to the Wnt/β-catenin signaling pathway, which needs further study.
Hence, our in vitro results have demonstrated that GO and GOQDs at the concentration of 1 μg/ml promoted SHED proliferation and GOQDs showed advantages over GO in inducing SHED osteogenesis. However, more research is required to confirm whether this occurs through the mechanism whereby GOQDs are taken up by SHEDs so that the protein level of β-catenin in the cytoplasm was up-regulated and the Wnt/β-catenin signaling pathway was activated, then induced SHED osteogenic differentiation with a stronger ability to induce osteogenesis than GO.
Conclusions
In this study, we compared GO with GOQDs to study their effects on the osteogenic differentiation of SHEDs. Both GO and GOQDs facilitated SHED proliferation up to 7 days in vitro at the concentration of 1 μg/ml. Due to their photoluminescence properties, GOQDs were distributed homogeneously in the cytoplasm of SHEDs. In inducing SHEDs osteogenesis, GOQDs showed advantages over GO. Mineralization and ALP activity increased significantly in SHEDs treated with osteogenic induction medium containing GOQDs, whilst they decreased in the group treated with osteogenic induction medium containing GO. Moreover, the RNA and protein expression of OCN, Runx2, COL I and β-catenin in SHEDs were distinctly higher in the GOQDs group than in the GO group. All these results demonstrate GOQDs as a potential biomaterial for improving bone regeneration.
Disclosure statement
The authors have no competing interests to declare.
Additional information
Funding
References
- Henkel J, Woodruff MA, Epari DR, et al. Bone regeneration based on tissue engineering conceptions – a 21st century perspective. Bone Res. 2013;1:216–248.
- Ma L, Aijima R, Hoshino Y, et al. Transplantation of mesenchymal stem cells ameliorates secondary osteoporosis through interleukin-17-impaired functions of recipient bone marrow mesenchymal stem cells in MRL/lpr mice. Stem Cell Res Ther. 2015;6:104.
- Akar B, Tatara AM, Sutradhar A, et al. Large animal models of an in vivo bioreactor for engineering vascularized bone. Tissue Eng Part B Rev. 2018;24:317–325.
- Gan D, Liu M, Xu T, et al. Chitosan/biphasic calcium phosphate scaffolds functionalized with BMP-2-encapsulated nanoparticles and RGD for bone regeneration. J Biomed Mater Res A. 2018;106(10):2613–2624.
- Zhang N, Chen B, Wang W, et al. Isolation, characterization and multi-lineage differentiation of stem cells from human exfoliated deciduous teeth. Mol Med Rep. 2016;14:95–102.
- Alipour R, Adib M, Karimi MM, et al. Comparing the immunoregulatory effects of stem cells from human exfoliated deciduous teeth and bone marrow-derived mesenchymal stem cells. Iranlan J Allergy Asthma and Immunol. 2013;12:331–344.
- Miura M, Gronthos S, Zhao M, et al. SHEDs – stem cells from human exfoliated deciduous teeth. J Dent Res. 2003;82:B305.
- Werle SB, Lindemann D, Steffens D, et al. Carious deciduous teeth are a potential source for dental pulp stem cells. Clin Oral Investig. 2016;20:75–81.
- Nakamura S, Yamada Y, Katagiri W, et al. Stem cell proliferation pathways comparison between human exfoliated deciduous teeth and dental pulp stem cells by gene expression profile from promising dental pulp. J Endodont. 2009;35:1536–1542.
- Koyam N, Okubo Y, Nakao K, et al. Evaluation of pluripotency in human dental pulp cells. J Oral Maxillofac Surg. 2009;67:501–506.
- Rosa V, Dubey N, Islam I, et al. Pluripotency of stem cells from human exfoliated deciduous teeth for tissue engineering. Stem Cells Int. 2016;2016:5957806.
- Li B, Qu C, Chen C, et al. Basic fibroblast growth factor inhibits osteogenic differentiation of stem cells from human exfoliated deciduous teeth through ERK signaling. Oral Diseases. 2012;18:285–292.
- Seo BM, Sonoyama W, Yamaza T, et al. SHED repair critical-size calvarial defects in mice. Oral Dis. 2008;14:428–434.
- Zheng Y, Liu Y, Zhang CM, et al. Stem cells from deciduous tooth repair mandibular defect in swine. J Dent Res. 2009;88:249–254.
- Novoselov KS, Geim AK, Morozov SV, et al. Electric field effect in atomically thin carbon films. Science. 2004;306:666–669.
- Tran AV, Shim K, Vo TT, et al. Targeted and controlled drug delivery by multifunctional mesoporous silica nanoparticles with internal fluorescent conjugates and external polydopamine and graphene oxide layers. Acta Biomater. 2018;74:397–413.
- La W, Jin M, Park S, et al. Delivery of bone morphogenetic protein-2 and substance P using graphene oxide for bone regeneration. Int J Nano. 2014;91:107–116.
- Hu W, Peng C, Luo W, et al. Graphene-based antibacterial paper. ACS Nano. 2010;4:4317–4323.
- Yang K, Zhang S, Zhang G, et al. Graphene in mice: ultrahigh in vivo tumor uptake and efficient photothermal therapy. Nano Lett. 2010;10:3318–3323.
- Dreyer DR, Park S, Bielawski CW, et al. The chemistry of graphene oxide. Chem Soc Rev. 2010;39:228–240.
- Li D, Kaner RB. Materials science. Graphene-based materials. Science. 2008;320:1170–1171.
- Hong J, Shah NJ, Drake AC, et al. Graphene multilayers as gates for multi-week sequential release of proteins from surfaces. ACS Nano. 2012;6:81–88.
- Lee WC, Lim CH, Shi H, et al. Origin of enhanced stem cell growth and differentiation on graphene and graphene oxide. ACS Nano. 2011;5:7334–7341.
- Menaa F, Abdelghani A, Menaa B. Graphene nanomaterials as biocompatible and conductive scaffolds for stem cells: impact for tissue engineering and regenerative medicine. J Tissue Eng Regen Med. 2015;9:1321–1338.
- Mohammadrezaei D, Golzar H, Rezai RM, et al. In vitro effect of graphene structures as an osteoinductive factor in bone tissue engineering: a systematic review. J Biomed Mater Res. 2018;106:2284–2343.
- Rosa V, Xie H, Dubey N, et al. Graphene oxide-based substrate: physical and surface characterization, cytocompatibility and differentiation potential of dental pulp stem cells. Dent Mater. 2016;32:1019–1025.
- Wei C, Liu Z, Jiang F, et al. Cellular behaviours of bone marrow-derived mesenchymal stem cells towards pristine graphene oxide nanosheets. Cell Prolif. 2017;50:e123675. .
- Wu C, Xia L, Han P, et al. Graphene-oxide-modified beta-tricalcium phosphate bioceramics stimulate in vitro and in vivo osteogenesis. Carbon. 2015;93:116–129.
- Yi C, Liu D, Fong C, et al. Gold nanoparticles promote osteogenic differentiation of mesenchymal stem cells through p38 MAPK pathway. ACS Nano. 2010;4:6439–6448.
- Liu D, Yi C, Zhang D, et al. Inhibition of proliferation and differentiation of mesenchymal stern cells by carboxylated carbon nanotubes. ACS Nano. 2010;4:2185–2195.
- Li P, Di Stasio F, Eda G, et al. Luminescent properties of a water-soluble conjugated polymer incorporating graphene-oxide quantum dots. Chemphyschem. 2015;16:1258–1262.
- Shi Y, Pramanik A, Tchounwou C, et al. Multifunctional biocompatible graphene oxide quantum dots decorated magnetic nanoplatform for efficient capture and two-photon imaging of rare tumor cells. ACS Appl Mater Interfaces. 2015;7:10935–10943.
- Ouyang S, Hu X, Zhou Q. Envelopment-internalization synergistic effects and metabolic mechanisms of graphene oxide on single-cell chlorella vulgaris are dependent on the nanomaterial particle size. ACS Appl Mater Interfaces. 2015;7:18104–18112.
- Zhu X, Wu G, Lu N, et al. A miniaturized electrochemical toxicity biosensor based on graphene oxide quantum dots/carboxylated carbon nanotubes for assessment of priority pollutants. J Hazard Mater. 2017;324:272–280.
- Murugesan B, Sonamuthu J, Pandiyan N, et al. Photoluminescent reduced graphene oxide quantum dots from latex of Calotropis gigantea for metal sensing, radical scavenging, cytotoxicity, and bioimaging in Artemia salina: a greener route. J Photochem Photobiol B. 2018;178:371–379.
- Ji X, Xu B, Yao M, et al. Graphene oxide quantum dots disrupt autophagic flux by inhibiting lysosome activity in GC-2 and TM4 cell lines. Toxicology. 2016;374:10–17.
- Choi SY, Baek SH, Chang SJ, et al. Synthesis of upconversion nanoparticles conjugated with graphene oxide quantum dots and their use against cancer cell imaging and photodynamic therapy. Biosens Bioelectron. 2017;93:267–273.
- Qiu J, Li D, Mou X, et al. Effects of graphene quantum dots on the self-renewal and differentiation of mesenchymal stem cells. Adv Healthc Mater. 2016;5:702–710.
- Singh Z. Applications and toxicity of graphene family nanomaterials and their composites. Nanotechnol Sci Appl. 2016;9:15–28.
- Perera SD, Mariano RG, Khiem V, et al. Hydrothermal synthesis of graphene-TiO2 nanotube composites with enhanced photocatalytic activity. ACS Catal. 2012;2:949–956.
- Kudin KN, Ozbas B, Schniepp HC, et al. Raman spectra of graphite oxide and functionalized graphene sheets. Nano Lett. 2008;8:36–41.
- Xie H, Chua M, Islam I, et al. CVD-grown monolayer graphene inducesos osteogenic but not odontoblastic differentiation of dental pulp stem cells. Dent Mater. 2017;33:13–21.
- Park K, Koak J, Kim S, et al. Wettability and cellular response of UV light irradiated anodized titanium surface. J Adv Prosthodont. 2011;3:63–68.
- Ishiy FA, Fanganiello RD, Griesi-Oliveira K, et al. Improvement of in vitro osteogenic potential through differentiation of induced pluripotent stem cells from human exfoliated dental tissue towards mesenchymal-like stem cells. Stem Cells Int. 2015;2015:249098.
- Suchánek J, Suchánková KT, Řeháček V, et al. Proliferative capacity and phenotypical alteration of multipotent ecto-mesenchymal stem cells from human exfoliated deciduous teeth cultured in xenogeneic and allogeneic media. Folia Biol (Praha). 2016;62:1–14.
- Nishino Y, Yamada Y, Ebisawa K, et al. Stem cells from human exfoliated deciduous teeth (SHEDs) enhance wound healing and the possibility of novel cell therapy. Cytotherapy. 2011;13:598–605.
- Garcia AJ, Reyes CD. Bio-adhesive surfaces to promote osteoblast differentiation and bone formation. J Dent Res. 2005;84:407–413.
- Zhang W, Yan L, Li M, et al. Deciphering the underlying mechanisms of oxidation-state dependent cytotoxicity of graphene oxide on mammalian cells. Toxicol Lett. 2015;237:61–71.
- Kang ES, Song I, Kim DS, et al. Size-dependent effects of graphene oxide on the osteogenesis of human adipose-derived mesenchymal stem cells[J]. Colloids Surf B Biointerfaces. 2018;169:20–29.
- Dalle CL, Innamorati G, Valenti MT. Transcription factor Runx2 and its application to bone tissue engineering. Stem Cell Rev. 2012;8:891–897.
- Pan K, Sun Q, Zhang J, et al. Multilineage differentiation of dental follicle cells and the roles of Runx2 over-expression in enhancing osteoblast/cementoblast-related gene expression in dental follicle cells. Cell Prolif. 2010;43:219–228.
- Kuo MY, Lan WH, Lin SK, et al. Collagen gene expression in human dental pulp cell cultures. Arch Oral Biol. 1992;37:945–952.
- Rahnama M, Jastrzębska-Jamrogiewicz I, Jamrogiewicz R, et al. Analysis of the influence of hormone replacement therapy on osteocalcin gene expression in postmenopausal women. Biomed Res Int. 2015;2015:1.
- Bakopoulou A, Leyhausen G, Volk J, et al. Wnt/beta-catenin signaling regulates dental pulp stem cells' responses to pulp injury by resinous monomers. Dent Mater. 2015;31:542–555.
- Yin X, Li J, Salmon B, et al. Wnt signaling and its contribution to craniofacial tissue homeostasis. J Dent Res. 2015;94:1487–1494.
- Jiang S, Chen G, Feng L, et al. Disruption of kif3a results in defective osteoblastic differentiation in dental mesenchymal stem/precursor cells via the Wnt signaling pathway. Mol Med Rep. 2016;14:1891–1900.