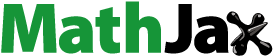
Abstract
The main aim of this study is to synthesize and prepare polyacrylamide (PAM)/polylactic acid (PLA) co-assembled core/shell nanofibers in order to investigate an effective dapsone-loaded capability and dapsone-release in the aqueous medium. Dapsone (4,4-diamino-diphenyl sulfone) has high permeability and low solubility in water. In vitro release testing indicates that maximum incorporation of the dapsone nanoemulsions into core/shell nanofibrous structures were 77.71 after 400 min. Products were characterized with X-Ray Diffraction (XRD), Scanning Electron Microscopy (SEM), Transmission Electron Microscopy (TEM), Fourier Transform Infrared Spectroscopy (FT-IR), Thermo-Gravimetric Analysis (TGA), Dynamic light scattering (DLS) analysis, Contact Angle Measurement (CAM) and nitrogen adsorption [i.e. Brunauer-Emmett-Teller (BET) Surface Area Analysis] techniques. The porosimetric measurements of the nanofibers structures showed that high porosity diameter, adsorption cross-section area, pore volumes and dead volume were obtained as 0.162 nm2, 0.1005 cm3g−1 and 15.693 cm3, respectively. TGA curve of the core/shell nanofibrous structures shows thermal stability between 240 °C and 260 °C.
Graphical Abstract
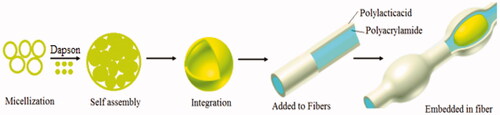
Introduction
Nanotechnology as a new perspective offers considerable ability to control and release hydrophobic and hydrophilic drugs [Citation1,Citation2]. The new and novel drug carriers [Citation3,Citation4] have found wide application as drug delivery systems [Citation5]. A large number of nanocarriers such as molecular imprinted nanopolymers (polymeric gel carriers, nanomicelles) and dynamically PEGylated [Citation6–9], lipids phase (liposomes and niosomes), solid lipid carriers, targeted drug delivery system and nanocapsules [Citation10–12] and nanopolymer carriers increased the in vitro release rate compared to bulk materials [Citation13,Citation14]. In fact, the major advantages of drug delivery nanocarriers such as hydrogels [Citation15], polymeric micelles [Citation16], reservoirs in implant devices [Citation17] and nanofibers [Citation18] are improved drug solubility, stability, performance and efficiency as well as efficacy for a long period of time [Citation19]. Rheological characteristics of PLA make it suitable for the cast and blown film extrusion and fiber spinning [Citation20]. PLA as the compatible and degradable polymer has been developed for many biotechnology applications [Citation21]. This has been solved by employing branching by the treatment of PLA with peroxide or by the introduction of multifunctional initiators or monomers. Several approaches exist to synthesize, design and develop core/shell nanofibers such as hydrothermal [Citation22], electrospinning [Citation23], coaxial electrospinning [Citation24] and microwave phase [Citation25]. The coaxial spinning method used as a modified electrospinning setup where two compounds with different solutions are primarily fed in co-axial capillary channel [Citation26]. Dapsone (4,4′-diaminodiphenylsulfone) as a sulfone class of drugs discovered in the early nineteenth century is a synthetic derivative of diamino-sulfone with anti-inflammatory and anti-bacterial properties [Citation27]. In recent researches, sulphone compounds have attracted considerable attention due to versatile bioactive [Citation28], acidic and anti-inflammatory properties. shows the molecular structure of dapsone. The emulsion method was used to ensure the successful development of a high-quality uniform distribution of drugs [Citation29]. Nanoemulsions as emulsions having droplet sizes between 10 and 200 nm are introduced as systems with high potential for biological delivery and controlled release of drugs. Nanoemulsions as a suitable carrier system resolve the solubility problem of lipophilic drugs with increased energy dissipation associated [Citation30–32]. The size decrease of the dapsone structures with transmutation of microemulsion phase to nanoemulsion phase leads to improvement loading in core/shell nanofibrous structures [Citation33]. Electrospinning technique is widely applicable for generating membranes and nanofibres containing different drugs [Citation34], and for removal or degradation of harmful organic molecules from the wastewater of the pharmaceutical industry [Citation35]. In recent years, many researchers have reported synthesis and usage of many core/shell nanofibers such Fe3O4@SiO2 [Citation36], Bio-Inspired/-Functional Colloidal Core-Shell polymeric [Citation37], poly(vinyl alcohol)/dextran nanofibers [Citation38] and paclitaxel/chitosan co-assembled that have been characterized by the electrospinning technique [Citation39].
Figure 1. 3D molecular structure of dapson (a) and the XRD patterns of the polyacrylamide/polylactic acid co-assembled core/shell nanofiber structures (b).
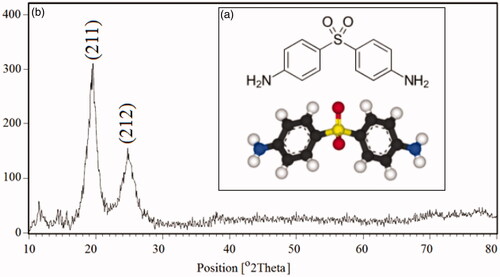
In this research, initially nanoemulsion of dapsone molecules was prepared by the reflux method, then we have proved which polyacrylamide/polylactic acid core/shell nanofibers were able to act as a suitable carrier. The PAM/PLA core/shell nanofibers encapsulated dapsone nanoemulsion in the form of beads, for example, beads and rosary. One of the most important issues in this research was an investigation of the comparing the durability for dapsone loaded in the polyacrylamide/polylactic acid core/shell nanofibers and the nanoemulsion phase. The effects of experimental parameters such as concentration, reaction time and pH as well as device parameters containing the feed rate of the core on the coaxial electrospinning process, the core/shell structure and the fiber morphology were investigated using the SEM, TEM analysis. In recent years, many studies have been conducted on the use of nanofibers as the drug delivery system. present a summary of nanofibers and target drugs researches. We anticipate which dapsone loaded in core/shell nanofibers can be used as a medicine pad for this reason. Since the optimal pH of human skin is 5.5–7.5, we measure pH in the range between 5.5 and 8.0 for all formulations.
Table 1. Review of different core/shell nanofibers for drug delivery.
Experimental
Materials and methods
All reagents were of analytical grade and were used without further purification. Sodium hydroxide (NaOH) was purchased from Merck Company and without any purification. Polylactic acid (PLA) granule, (MW = 60,000 g/mol) with purity >99% and 3 mm nominal granule size powder and polyacrylamide (C3H5NO)n, MW = 40,000 with purity >99.9% were purchased from Sigma-Aldrich Company. Dapsone, as an antibiotic drug in diaminodiphenyl sulfone (DDS) group, was obtained from pharmaceutics laboratories (KMU, Kerman) used in this study. In this work, in the first step, we synthesized and prepared dapsone nanoemulsions using microemulsion formulations. Then, dapsone nanoemulsions were incorporated in PAM/PLA core/shell nanofibers as biodegradable and bioactive compounds. Therefore, this study discussed the synthesis of dapsone nanoemulsions loaded in PAM/PLA core/shell nanofibers in three steps as follows: Step ι: Initially, microemulsion phase of dapsone was prepared in the present surfactant and cosurfactant. This step is carried out to dissolve dapsone (DAP) as hydrophobic drug molecules. Step ιι: Nanoemulsion phase dapsone was formed with external energy and temperature through the reflux technique. The main strategy in this step was sustained release of dapsone as hydrophobic drug and the highest percentage loaded in electrospun fibers. Step ιιι: loading optimization of dapsone nanoemulsion was carried out in PAM/PLA core/shell nanofibers structures. In this step, dapsone nanoemulsion is established as the stable connection with the core surface (Polyacrylamide) as the hydrophilic component.
Preparation of micro and nanoemulsion/dapsone
In a typical and creative method at the first stoichiometric amount of Tween 80, 60, 40 as surfactant phase and Span of 20, 40, 60 as cosurfactant phase dissolved in 20 ml deionized water under vigorous stirring with 300 rpm using a magnetic stirrer at 45 °C for 120 min. Then, oleic acid with different percentage ratio according to was added to the above solution. By adding the surfactant concentration, critical point (CMC) was achieved, and pH was adjusted with HCl 0.1 M and NaOH 2 M between 5.5 and 8.0 ± 0.2 with added dropwise NaOH 2 M. In the next step, to prepare dapsone microemulsions, 80 mg of dapsone (dapsone to surfactant, 5:1) was dissolved in 30 ml of DMF. Then, this solution was added to the above mixture phase subsequently under reflux system at 50 °C for 120 min. Structural characteristics and properties of the nanoemulsions were evaluated using SEM (Scanning electron microscopy), TEM (Transmission electron microscopy), AFM (Atomic force microscopy), FT-IR (Fourier-transform infrared) spectroscopy.
Table 2. Reaction conditions for preparation of micro and nanoemulsion/dapson.
Preparation of PAM/PLA core/shell nanofibers containing dapsone nanoemulsion
PAM/PLA core/shell nanofibers were prepared by the electrospinning method. At first, 5.0 g PAM powder was dissolved into deionized water (25 ml) and stirred at 50 °C for 30 min. Then, 5 ml of dapsone nanoemulsion was added to polyacrylamide solution and pH was adjusted between 6 and 7. To fabricate the shell layer, a stoichiometric amount (10 wt%) of polylactic acid was dissolved in chloroform and put in constant stirring for 60 min at 45 °C. Both of the mixture solutions, namely core (polyacrylamide contains dapsone nanoemulsion) layer and shell (polylactic acid) layer were sonicated for 10 min in 60-watt frequency to obtain a homogeneous mixture before using. Two concentrically arranged capillaries exist in the coaxial spinneret. The inner capillary had inner and outer diameters of 0.35 mm and 0.65 mm, respectively, while the outer capillary had inner and outer diameters of 1.05 mm and 1.20 mm, respectively. Electrospinning device was set up with a high-voltage power supplying generating voltages up to 30 kV, as the source of the electric field. The feed rate of the sprayed polymer on the controller was 1.0 ml/h with electrical potential 15 kV. In the across process, the distance between the tip of the nozzle and the outer surface of the drum was 10 cm. Finally, nanofibers were collected on a sheet of aluminium, the system was allowed to cool to room temperature naturally, and the obtained precipitations were collected. Scheme 1 shows the summary of the experimental method to form polyacrylamide/polylactic acid co-assembled core/shell nanofibers containing dapsone nanoemulsion.
Results and discussion
This work aimed to synthesis, prepare and investigate efficient encapsulation and release study of the dapsone as a lipophilic drug, and the polyacrylamide/polylactic acid co-assembled core/shell nanofibers were selected as a vehicle to protect and deliver nanoemulsion system such as dapsone as a lipophilic drug.
Structural analysis (XRD)
The X-ray diffraction (XRD) as one of the most important techniques is superior in elucidating the three-dimensional atomic structure, phase identification and crystallographic detection of crystalline solids. The XRD patterns of the structures were carried out between (10 < 2θ < 80) and shown in . Two reflection peaks of the XRD pattern for polyacrylamide/polylactic acid co-assembled core/shell nanofibers are well indexed with the calculated cell parameters. The crystalline size and diameter (Dc) of nanofibers can be determined as ∼131–168 nm from the diffraction patterns, from the full width of the half maximum (FWHM) with the help of Debay–Scherer equations (EquationEq. (1–4)):
(1)
(1)
Where
(2)
(2)
for sample
(4)
(4)
for standard use
(5)
(5)
where β is the breadth of the observed diffraction line at its half intensity maximum, K is the so-called shape factor, which usually takes a value of approximately 0.9 assuming the particle shape is spherical.
Morphological structure
Scanning electron microscope’s (SEM) microphotograph of samples was evaluated with SEM (LEO 1455VP, Leo Trading Company, Hong Kong), compeer for the Brookhaven Instruments 90 Plus particle size analyzer at the University of Tehran. shows the SEM image of dapsone microemulsion. As the results show, the morphologies of the microemulsion were cumulative of emulsions with the spherical-like self-assembly. It is anticipated that in critical micelle concentration (CMC), surfactants tail containing dapsone makes organized structures. Polyacrylamide/polylactic acid creates core/shell nanofibers as shown in , and nanofiber structures were synthesized without any tangles and rupture. Polyacrylamide as hydrophilic polymer putting yourself in dapsone microemulsion shows the SEM image of dapsone microemulsion loaded in the polyacrylamide/polylactic acid as core/shell nanofibers. Results show that in some areas of the polyacrylamide/polylactic acid core/shell nanofibers, due to excessive accumulation of dapsone microemulsion, the strings are cut and separated. In this study, the accuracy of the method was repeated using multiple experiments.
Figure 2. SEM image cumulative of dapsone microemulsion (a), polyacrylamide/polylactic acid core/shell nanofibers (b) dapsone nanoemulsion loaded in polyacrylamide/polylactic acid as core/shell nanofibers (c).
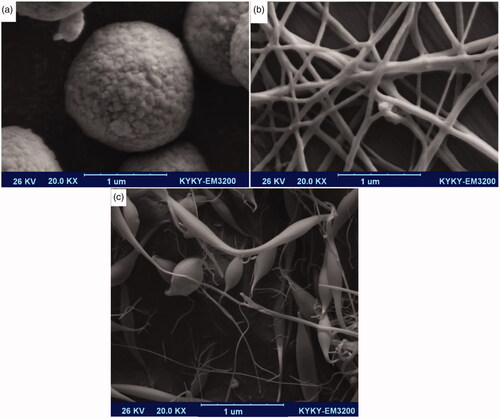
Some of the key objectives of morphological structures are mentioned in the following cases:
Dapsone acts as the lipophilic drug capture in micro and nanoemulsion.
Polyacrylamide acts as the protect coverage against environmental factors in the body such as pH, temperature and other materials that can react with dapsone.
Polyacrylamide/polylactic nanofibers acid acts as a suitable carrier for lipophilic drugs.
The particle size and particle size distribution of the structures can be measured with dynamic light scattering (DLS) analysis [Citation40–44]. In this study size distribution of the dapsone micro and nanoemulsion were determined by laser-light scattering (Mastersizer 2000E, Malvern Instruments, UK) which are shown in . To study the quality, shape, size, density of micro and nanoemulsion as well as loading in nanofibers, we used (transmission electron microscopy) TEM images. In this study, TEM images were obtained on a Philips EM208S transmission electron microscope with an accelerating voltage of 100 kV. The higher concentrations of dapsone molecules in micro and nanoemulsions were self-assembled as spherical-like structures. indicate TEM images of the synthesized dapsone microemulsion and drug loaded on core/shell nanofibers, respectively. The higher density of dapsone micro and nanoemulsion in provide more clarity to display hollow areas.
Thermogravimetric analysis/atomic force microscopy
To investigate the thermal stability of the core/shell nanofibers into the amount of weight change of a material as a function of increasing temperature, thermogravimetric analysis (TGA) was calculated. This analysis was evaluated with the gravimeter S-15000 model under a nitrogen with the heating rate of 10 °C/min from 100 °C up to 400 °C. indicates TGA and DTA (derivative TGA curves for dapsone micro and nanoemulsion loaded in polyacrylamide/polylactic core/shell nanofibers). The degradation behaviors of dapsone in nanofibers indicate a continuous weight loss of 6.06% at the temperatures around 120–130 °C, which is ascribed to the degradation of the surfactant phase in micro and nanoemulsions. After 140 °C, dapsone molecules up to 240 °C will have decomposed completely by a rapid weight loss about 96.16%. Nanofibers improved thermal stability up to 250 °C. The weight loss increases with an increase at the temperature up to 310 °C and the most significant weight loss could be related to the full thermal decompose of polyacrylamide and polylactic. Therefore, it can be concluded that polyacrylamide/polylactic core/shell nanofibers as cover layer carriers delayed thermal decomposite of dapsone micro and nanoemulsions. Atomic force microscopy (AFM) has been calculated with Nano wizard II JPK, Made in Germany. Atomic force microscopy analysis was used to measure the surface properties and surface topography. shows AFM images of dapsone micro and nanoemulsion loaded in the polyacrylamide/polylactic core/shell nanofibers. According to the AFM images, seamless strands of nanofibers are observed having bumps arising from dapsone micro and nanoemulsion.
Contact-angle measurements
Majority of the failures in the new drug development have been attributed to the poor water solubility of the drug. The contact angle for polyacrylamide/polylactic core/shell nanofibers, nanoemulsions and encapsulated structures (the advancing contact angle, or θA) were measured as a function of time for different drop volumes. shows the relationship between advancing contact angles and time for different drop volumes. Contact angle measurements were performed by dripping a drop of water on the surface under a contact angle of 90°. Contact angle measurement shows that the advancing contact angle for dapsone micro and nanoemulsions is ∼24°. Hydrophilic properties of emulsions return to the unique self-assembled structures. With encapsulated micro and nanoemulsions on polyacrylamide/polylactic acid, core/shell nanofibers contact angle increases to 46°. This angle has significantly decreased compared to 94° for polyacrylamide/polylactic acid core/shell nanofibers.
BET analysis
Surface area evaluation and the pore size distribution precision of materials were measured under the nitrogen atmosphere by the Brunauer–Emmett–Teller (BET) method. The N2 adsorption–desorption isotherms of polyacrylamide/polylactic acid core/shell nanofibers were recorded using Belsorp II-BEL equipment (BEL Japan Inc., Osaka, Japan) that is shown in . Adsorption cross-section area, total pore volumes and dead volume were obtained as 0.162 nm2, 0.1005 cm3g−1 and 15.693 cm3 by applying the Barret–Joyner–Halenda (BJH) method, respectively. In addition, the average pore diameter was calculated as 18.63 nm with BET analysis. The polyacrylamide/polylactic acid core/shell nanofibers have the individual hollow spaces that these results show high properties of polyacrylamide/polylactic acid as the suitable carrier for lipophilic drugs.
In-vitro drug release studies
The encapsulation efficiency value was calculated using UV–Vis spectrophotometer at 260 nm in ABS. A percentage of 0.9 in the presence of ethanol 96% and buffer phosphate 7.2 was calculated as ∼99.88%. The drug entrapment efficiency (DEE) of DAP in the core–shell nanofibers were evaluated according to EquationEq. (6)(6)
(6) .
(6)
(6)
where Wt is the total amount of the incorporated material, and Wi is the total quantity of incorporated material added initially during the preparation. In vitro dapsone release from polyacrylamide/polylactic acid core/shell nanofibers was studied with UV spectrometer S-3100 SCINCO. shows the cumulative release profiles of dapsone from micro and nanoemulsions and modified with polyacrylamide/polylactic acid core/shell nanofibers. In vitro release profiles were calculated with 10 ml of phosphate buffer (pH 6.5 and 7.2) in 0–400 min. A number of parameters such as surface erosion, desorption and diffusion of the active agents from the surface of structures in the polymeric substrates have a direct effect on the drug release property. Incorporation of dapsone nanoemulsion into polyacrylamide/polylactic acid core/shell nanofibers may be a long-term promising system for enabling topical delivery of dapsone. Results show that the drug release rate of dapsone from core/shell nanofibers has longer time compared to nanoemulsion; this is due to the creation of a protective layer of polyacrylamide. After 400 min, 80.61% dapsone was released from nanoemulsion structures, while the amount of the drug released for polyacrylamide/polylactic acid core/shell nanofibers modified with nanoemulsion containing dapsone was 77.71% at the same time. The release profile of Dapsone from the designed delivery system follows the zero-order pattern, and dissolution of the Dapsone from pharmaceutical dosage forms, which do not disaggregate and release the drug slowly, can be represented by the following equation (EquationEq. (7))
(7)
(7) :
(7)
(7)
Figure 7. The cumulative release profiles of dapsone from nanoemulsion and core/shell nanofibers modified with nanoemulsion. Percentage of released niosomegel, dapsone solution, noisome and nanofiber niosomes.
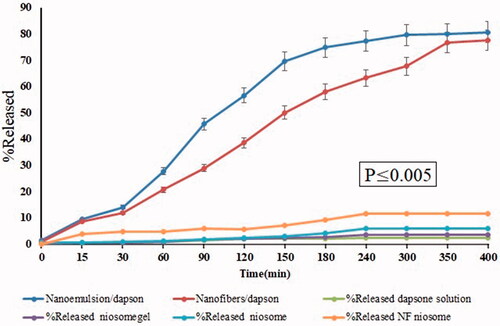
Where, W0 is the initial amount of the drug in the pharmaceutical dosage form, Wt is the amount of drug in the pharmaceutical dosage form at time t, and K is the proportionality constant. We can use the above equation and matrix tablets with low, soluble drugs, coated forms, in the case of some osmotic systems and transdermal systems. For a better understanding and comparison, we have inserted charts for percent released niosomegel, percent released dapsone solution, percent released noisome and percent released nanofiber niosomes as the blank sample. Results show that nanoemulsion and nanofibers modified with nanoemulsion containing dapsone have significant differences on release than other forms, which can be due to a significant increase in particle size absorption.
Conclusion
In this study, dapsone molecules were entrapped in the polyacrylamide/polylactic acid co-assembled core/shell nanofibers and physicochemical properties investigated. The results indicate that the polyacrylamide/polylactic acid core/shell nanofibers structures are synthesized with high purity. Morphological structures of the nanofibers show beads-like structures for dapsone loaded in polyacrylamide/polylactic acid. The polyacrylamide/polylactic core/shell nanofibers act as suitable carriers related to an increase in thermal stability of dapsone micro and nanoemulsions. The rate of drug release polyacrylamide/polylactic core/shell nanofibers and nanoemulsion structures after 400 min was 77.71% and 80.61%, respectively.
Compliance with ethics requirements
This article does not contain any studies with human or animal subjects.
Acknowledgements
Authors are grateful of Pharmaceutics Research Center, Institute of Neuropharmacology, Kerman University of Medical Sciences, Kerman, Iran.
Disclosure statement
All authors declare that they have no competing or no conflict of interests.
References
- Adarsh NN, Kumar DK, Dastidar P. Zn(II) metal-organic frameworks (MOFs) derived from a bis-pyridyl-bis-urea ligand: effects of crystallization solvents on the structures and anion binding properties. CrystEngComm. 2008;10:1565–1573.
- Moore AN, Lopez Silva TL, Carrejo NC, et al. Nanofibrous peptide hydrogel elicits angiogenesis and neurogenesis without drugs, proteins, or cells. Biomaterials. 2018;161:154–163.
- Malafaya PB, Silva GA, Reis RL. Natural–origin polymers as carriers and scaffolds for biomolecules and cell delivery in tissue engineering applications. Adv Drug Del Rev. 2007;59:207–233.
- Singh RP, Gangadharappa H, Mruthunjaya K. Phospholipids: unique carriers for drug delivery systems. J Drug Del Sci Technol. 2017;39:166–179.
- Delgado-Rosales EE, Quintanar-Guerrero D, Piñón-Segundo E, et al. Novel drug delivery systems based on the encapsulation of superparamagnetic nanoparticles into lipid nanocomposites. J Drug Del Sci Technol. 2018;46:259–267.
- Sheykhaghaei G, Sadr MH, Khanahmadzadeh S. Synthesis and characterization of core–shell magnetic molecularly imprinted polymer nanoparticles for selective extraction of tizanidine in human plasma. Bull Mater Sci. 2016;39:647–653.
- Liu S, Pan J, Liu J, et al. Dynamically PEGylated and borate-coordination-polymer-coated polydopamine nanoparticles for synergetic tumor-targeted, chemo-photothermal combination therapy. Nano Micro Small. 2018;14(13):1703968.
- Peng Y, Nie J, Cheng W, et al.A multifunctional nanoplatform for cancer chemo-photothermal synergistic therapy and overcoming multidrug resistance. Biomater Sci. 2018;6(5):1084–1098.
- Zeng X, Tao W, Mei L, et al.Cholic acid-functionalized nanoparticles of star-shaped PLGA-vitamin E TPGS copolymer for docetaxel delivery to cervical cancer. Biomaterials. 2013;34(25):6058–6067.
- Mehnert W, Mäder K. Solid lipid nanoparticles: production, characterization and applications. Adv Drug Del Rev. 2012;64:83–101.
- Liu G, Tsai H, Zeng X, et al. Phosphorylcholine-based stealthy nanocapsules enabling tumor microenvironment-responsive doxorubicin release for tumor suppression. Theranostics. 2017;7(5):1192–1203.
- Zhu D, Tao W, Zhang H, et al.Docetaxel (DTX)-loaded polydopamine-modified TPGS-PLA nanoparticles as a targeted drug delivery system for the treatment of liver cancer. Acta biomaterialia. 2016;30:144–154.
- He Y, Zhang H, Li Y, et al. Synergistic proton transfer through nanofibrous composite membranes by suitably combining proton carriers from the nanofiber mat and pore-filling matrix. J Mater Chem A. 2015;3:21832–21841.
- Gao N, Chen Z, Xiao X, et al.. Surface modification of paclitaxel-loaded tri-block copolymer PLGA-b-PEG-b-PLGA nanoparticles with protamine for liver cancer therapy. J Nanopart Res. 2015;17(8):347.
- Hirakura T, Yasugi K, Nemoto T, et al. Hybrid hyaluronan hydrogel encapsulating nanogel as a protein nanocarrier: new system for sustained delivery of protein with a chaperone-like function. J Control Rel. 2010;142:483–489.
- Lim E-K, Sajomsang W, Choi Y, et al. Chitosan-based intelligent theragnosis nanocomposites enable pH-sensitive drug release with MR-guided imaging for cancer therapy. Nanoscale Res Lett. 2013;8:467.
- Varner SE, Dejuan E, Barnes AC, et al., Inventors; Johns Hopkins University. Reservoir device for intraocular drug delivery. United States patent US7883717B2. 2011 February 8..
- Luo Y, Shen H, Fang Y, et al. Enhanced proliferation and osteogenic differentiation of mesenchymal stem cells on graphene oxide-incorporated electrospun poly (lactic-co-glycolic acid) nanofibrous mats. ACS Appl Mater Interfaces. 2015;7: 6331–6339.
- Khadka P, Ro J, Kim H, et al. Pharmaceutical particle technologies: an approach to improve drug solubility, dissolution and bioavailability. Asian J Pharma Sci. 2014;9:304–316.
- Al-Itry R, Lamnawar K, Maazouz A. Rheological, morphological, and interfacial properties of compatibilized PLA/PBAT blends. Rheol Acta. 2014;53:501–517.
- Csikós Á, Faludi G, Domján A, et al. Modification of interfacial adhesion with a functionalized polymer in PLA/wood composites. Euro Polym J. 2015;68:592–600.
- Kong J, Liu Z, Yang Z, et al. Carbon/SnO 2/carbon core/shell/shell hybrid nanofibers: tailored nanostructure for the anode of lithium ion batteries with high reversibility and rate capacity. Nanoscale. 2012;4:525–530.
- Kumar M, Klimke S, Preiss A, et al. Electrospinning synthesis and characterization of PLA-PEG-MNPs composite fibrous membranes. Hyperfine Interactions. 2017;238:66.
- Zamani M, Prabhakaran MP, Ramakrishna S. Advances in drug delivery via electrospun and electrosprayed nanomaterials. Int J Nanomed. 2013;8:2997.
- Zhao X-Y, Zhu Y-J, Qi C, et al. Hierarchical hollow hydroxyapatite microspheres: microwave‐assisted rapid synthesis by using pyridoxal‐5′‐phosphate as a phosphorus source and application in drug delivery. Chem Asian J. 2013;8:1313–1320.
- Qin Z, Zhang P, Wu Z, et al. Coaxial electrospinning for flexible uniform white-light-emitting porous fibrous membrane. Mater Des. 2018;147:175–181.
- Essousi H, Barhoumi H. Electroanalytical application of molecular imprinted polyaniline matrix for dapsone determination in real pharmaceutical samples. Electroanal Chem. 2018;818:131–139.
- Lee KH, Park JH, Kim DH, et al. Dapsone as a potential treatment option for Henoch-Schönlein Purpura (HSP). Medical Hypotheses. 2017;108:42–45.
- Koroleva M, Nagovitsina T, Yurtov E. Nanoemulsions stabilized by non-ionic surfactants: stability and degradation mechanisms. Phys Chem Chem Phys. 2018;20:10369–10377.
- Hussain A, Samad A, Singh SK, et al. Nanoemulsion gel-based topical delivery of an antifungal drug: in vitro activity and in vivo evaluation. Drug Deliv. 2016;23:642–657.
- Patel RB, Patel MR, Thakore SD, et al. Nanoemulsion as a valuable nanostructure platform for pharmaceutical drug delivery. In Nano-and Microscale Drug Delivery Systems. Design and Fabrication; 2017. p. 321–341.
- Jaiswal M, Dudhe R, Sharma PK. Nanoemulsion: an advanced mode of drug delivery system. 3 Biotech. 2015;5:123–127.
- de Almeida Borges VR, et al. Nanoemulsion containing dapsone for topical administration: a study of in vitro release and epidermal permeation. Int J Nanomed. 2013;8:535.
- Wang B, Wang Y, Yin T, et al. Applications of electrospinning technique in drug delivery. Chem Eng. 2010;197:1315–1338.
- Baruah S, Dutta J. Nanotechnology applications in pollution sensing and degradation in agriculture: a review. Environ Chem Lett. 2009;7:191–204.
- Lungu II, Radulescu M, Mogosanu GD, et al. pH sensitive core-shell magnetic nanoparticles for targeted drug delivery in cancer therapy. Rom J Morphol Embryol. 2016;57:23–32.
- Haidar ZS. Bio-inspired/-functional colloidal core-shell polymeric-based nanosystems: technology promise in tissue engineering, bioimaging and nanomedicine. Polymers 2010;2:323–352.
- Moydeen AM, Ali Padusha MS, Aboelfetoh EF, et al. Fabrication of electrospun poly(vinyl alcohol)/dextran nanofibers via emulsion process as drug delivery system: kinetics and in vitro release study. Int J Biol Macromol. 2018;116:1250–1259.
- Tang J, Liu Y, Zhu B, et al. Preparation of paclitaxel/chitosan co-assembled core-shell nanofibers for drug-eluting stent. Appl Surf Sci. 2017;393:299–308.
- Zeng X, Luo M, Liu G, et al. Polydopamine‐modified black phosphorous nanocapsule with enhanced stability and photothermal performance for tumor multimodal treatments. Adv Sci. 2018;5(10):1800510..
- Liang C, Wang H, Zhang M, et al. Self-controlled release of Oxaliplatin prodrug from d-α-tocopheryl polyethylene glycol 1000 succinate (TPGS) functionalized mesoporous silica nanoparticles for cancer therapy. J Coll Interface Sci. 2018;525:1–10.
- Zeng X, Liu G, Tao W, et al. A drug‐self‐gated mesoporous antitumor nanoplatform based on pH‐sensitive dynamic covalent bond. Adv Funct Mater. 2017;27:1605985.
- Cheng W, Liang C, Xu L, et al. TPGS-functionalized polydopamine-modified mesoporous silica as drug nanocarriers for enhanced lung cancer chemotherapy against multidrug resistance. Nano Micro Small. 2017;13(29):1700623.
- Cheng W, Nie J, Gao N, et al. A multifunctional nanoplatform against multidrug resistant cancer: merging the best of targeted chemo/gene/photothermal therapy. Adv Funct Mater. 2017;27(45):1704135.
- Mickova A, Buzgo M, Benada O, et al. Core/shell nanofibers with embedded liposomes as a drug delivery system. Biomacromolecules. 2012;13:952–962.
- Onoe H, Okitsu T, Itou A, et al. Metre-long cell-laden microfibres exhibit tissue morphologies and functions. Nature Mater. 2013;12:584.
- Bonadies I, Maglione L, Ambrogi V, et al. Electrospun core/shell nanofibers as designed devices for efficient Artemisinin delivery. Euro Polym J. 2017;89:211–220.
- Nguyen TTT, Chung OH, Park JS. Coaxial electrospun poly (lactic acid)/chitosan (core/shell) composite nanofibers and their antibacterial activity. Carbohydrate Polym. 2011;86:1799–1806.
- Cui W, Zhou Y, Chang J. Electrospun nanofibrous materials for tissue engineering and drug delivery. Sci Technol Adv Mater. 2010;11:014108.
- Chen P, Wu Q-S, Ding Y-P, et al. A controlled release system of titanocene dichloride by electrospun fiber and its antitumor activity in vitro. Euro J Pharma Biopharma. 2010;76:413–420.