Abstract
The aim of this paper was to create an in vitro setup to quantify the oxygen offloading capabilities of dodecafluoropentane emulsion (DDFPe) in a hypoxic environment. Oxygen offloading from DDFPe was characterized under different gaseous environments and under pre-oxygenated conditions. Results of this study showed that (1) oxygen offloading is inversely related to the solubility of the selected sparging gas in saline and (2) both pre-oxygenated and simultaneously oxygenated DDFPe display similar magnitudes of oxygen transport. These results could be applicable to on-going and future studies involving a variety of hypoxic conditions where oxygen delivery might be therapeutically beneficial.
Introduction
Intravenous therapeutics that are able to facilitate the exchange of respiratory gases have shown promising results in treating a variety of acute medical conditions including stroke, traumatic brain injury (TBI) [Citation1–3], and haemorrhagic shock [Citation2,Citation4]. It is well known that the body requires both a constant supply of oxygen and an effective mechanism to remove carbon dioxide to maintain proper metabolic function. Consequently, an injectable therapeutic should aim to facilitate efficient gas exchange and restore adequate oxygen delivery after an acute hypoxic event. Because of their unique chemistry, recent investigations have focused on the use of stabilized perfluorocarbon emulsions for the re-oxygenation of hypoxic tissues.
Perfluorocarbons (PFCs) have a long history of being explored as red blood cell substitutes (RBCS) since 1966, when Clark and Gollan demonstrated that a rat immersed in an oxygenated, perfluorocarbon solution could respire normally. The hyperfluorination present in PFCs results in an ideal gas like chemical inertness that allows for lack of metabolism in vivo [Citation5] and additionally allows for the dissolving of gases of similarly low cohesivity such as O2, CO2, N2 and NO [Citation6]. The efficacy of PFC’s as an oxygenation therapeutic, however, is due to its lower affinity for the O2 molecule in comparison to haemoglobin. Under atmospheric PO2, PFCs are much less efficient oxygen-dissolving agents than blood; however, physiologically, the oxygen delivery capability takes priority over the oxygen dissolving capability [Citation7]. The combination of PFCs lower affinity for O2, in concurrence with its higher diffusion rates, allows for more efficient O2 delivery and extraction by the tissues [Citation8]. However, isolated PFCs are not stable enough to be used as an injectable therapeutic. Consequently, recent PFC-based studies have investigated the use of stabilized perfluorocarbon emulsions, which utilize the encapsulation of PFCs in a surfactant monolayer to allow for the prolongation of its intravascular stability and half-life [Citation9].
One such stabilized PFC emulsion is dodecafluoropentane emulsion (DDFPe). DDFPe was originally developed as an ultrasound contrast agent by Sonus Pharmaceuticals (Bothell, Washington, DC) under the name EchoGenTM as a 2% w/v sub-microemulsion of dodecafluoropentane (DDFP) [Citation10]. Sonus Pharmaceuticals licensed the rights for DDFPe to NuvOx Pharma (Tucson, Arizona), which repurposed its development as an oxygen therapeutic for indications such as stroke and TBI, and as a radiosensitizer to treat glioblastoma multiforme (GBM). DDFPe has been tested at doses of 0.05, 0.1, and 0.17 ml/kg (0.001, 0.002, and 0.0034 g DDFP/kg) in a Phase Ib/II clinical trial for GBM [Citation11] and has been shown to be safe in humans at three doses of 0.17 ml/kg administered 90 min apart in a Phase Ib/II trial in acute ischemic stroke patients.
The oxygen-carrying capacity of PFCs in their liquid state is directly related to their CF3 group density [Citation12] and inversely related to their molecular weight and boiling point, so long as the ambient temperature does not exceed the boiling point. [Citation13]. As the PFC approaches its boiling point, it experiences a phase of expansion where the CF3 cavity space increases and can acts as a “respiratory sink” for surrounding O2 to dissolve into. Past its boiling point, a PFC will generally volatilize. Consequently, this expansion phase between liquid and gas states acts as the ideal state for oxygen absorption. DDFP has a much lower molecular weight and boiling point in comparison to preceding perfluorocarbon emulsions such as perftoran, fluosol, and oxycyte, which translates to a superior oxygen carrying capacity and efficacy at lower doses [Citation2]. It is important to note that DDFP boils at 29 °C and should theoretically volatilize in vivo at 37 °C. However, DDFP is stabilized in an emulsion, which is administered as an intravenous injection. The vasculature acts as a pressurized, closed-loop system, which allows the DDFP emulsion to remain stable in vivo and maintain its oxygen dissolving and offloading capability [Citation12,Citation13]. Larger PFCs, such as perfluorooctylbromide (PFOB) and perfluorodecalin (PFD), generally experience less expansion corresponding to their decreased oxygen dissolving abilities [Citation12]. In vitro experimentation using DDFP derived nanodroplets has proven its ability to exchange gas with its surrounding aqueous environment, and absorb up to three times more oxygen than perfluorodecalin emulsion (PFDe) and perfluorooctylbromide emulsion (PFOBe) at 21 °C and up to seven times more oxygen at 37 °C [Citation12]. Additional tests performed in an anaemic rat model using DDFPe have proven its ability to mediate and facilitate gas transport to maintain tissue function [Citation14].
The aim of this paper was to introduce an in vitro setup capable of quantifying the oxygen offloading capabilities of DDFPe in a hypoxic environment. The experimental setup aimed to address limitations of preceding in vitro analyses such as Johnson et al. [Citation12], which aimed to quantify the absorption of oxygen by DDFPe in comparison with other PFC emulsions. These limitations included the need to address (1) the effect of additional pressures exerted in a semi-closed circulatory system, (2) the effect of cyclic oxygenation and re-oxygenation of DDFPe as seen in biological circulatory systems, and (3) possible errors in dissolved oxygen measurements caused by the direct interaction between the probe and emulsion constituents. Additionally, the circuit worked to simulate efficient gas transfer in low volumes, with easily replaceable components to reduce inter-trial effects [Citation15]. These limitations were addressed through the use of SilasticTM tubing, which is permeable to gases, to create a semi-closed circulation system subjected to repetitive cycles of re-oxygenation [Citation16,Citation17]. The results of this in vitro experimental setup can be used to (1) quantify the oxygen offloading behaviour of DDFPe in various hypoxic environments and (2) explore whether pre-oxygenation of DDFPe prior to administration affects its oxygen offloading behaviour.
Materials and methods
The in vitro set-up illustrated in was designed to simulate gas exchange and oxygen delivery from the lungs to hypoxic tissues. The oxygen off-loading capability of the drug product, DDFPe, was tested through a series of assays () using the same in vitro experimental set up () while changing the inert gases used for purging the headspace and sparging the fluid, within the gas exchange vessel.
Figure 1. Experimental set-up. In vitro oxygenation set-up used to simulate gas exchange and oxygen delivery from the lungs (fluid reservoir) to hypoxic tissues (gas exchange vessel).
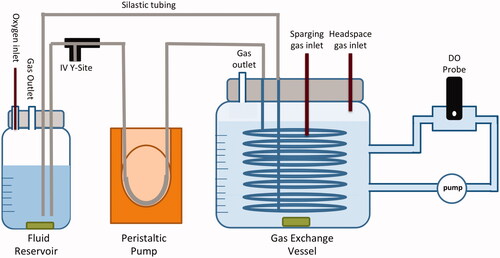
Table 1. Table of assays.
Preparation of product
Sodium chloride and sodium sulphite were purchased from Sigma Aldrich (St. Louis, MO) while water (18MΩ·cm) from an in-house purification system was used as the diluent. Dissolved oxygen measurements were obtained using an Oakton DO110 meter. Silastic tubing (1.47 mm I.D. × 1.96 mm O.D.) was purchased from VWR. DDFPe with 2% w/v PFC (active) and without PFC (PTB + sucrose blank) was prepared similar to the method previously described by Lundgren et al. [Citation18]. Specifically, sucrose solution was homogenized along with PEG-Telomer B (PTB) and DDFP (except for the blank). Each emulsion was processed using a semi-sealed, stainless steel containment system attached to an Avestin Emulsiflex-C50 homogenizer. The homogenates were then subject to terminal sterile filtration immediately prior to filling into 10 ml vials.
In vitro performance testing
In vitro model
The in vitro oxygenation experimental set-up was designed to simulate the oxygen uptake by the blood in the lungs, the transport of oxygen by the blood to the tissues, and finally, the uptake of oxygen by the tissues from the blood. The fluid reservoir held approximately 80 ml of solution and acted as the lungs where oxygen uptake occurs, while the gas exchange vessel held approximately 900 ml of saline and acted as the hypoxic tissue or site of oxygen offloading. Silastic™ tubing, used to simulate the circulatory system of the body [Citation16,Citation17], was weaved through polymer netting to maximize the surface area and placed inside the gas exchange vessel filled with a 0.9% saline solution. Sparging with either helium, nitrogen, or carbon dioxide gas was used to lower the dissolved oxygen concentration of saline in the gas exchange vessel to approximately 1.75 mg/l (. Since the gas exchange vessel was not completely sealed, a continuous supply of a given gas (2.0 standard ft3/min) was pumped into the headspace of the vessel throughout the duration of the experiment to displace air in the vessel and prevent re-oxygenation of the saline from atmospheric oxygen. Once the sparging process was complete, the solution in the fluid reservoir received a constant influx of oxygen (2.0 l/min). A peristaltic dosing pump was used to pump fluid out of the reservoir and through the Silastic™ tubing at a rate of 30 ml/min. To simulate drug administration, 1 ml of either saline (control), DDFPe (active), or the PTB + sucrose blank was injected into the IV Y-Site, where it travelled through the Silastic™ tubing to offload oxygen into the gas exchange vessel. The dissolved oxygen concentration within the gas exchange vessel was continuously monitored through an external circuit in which the fluid oxygen concentration was sampled using a dissolved oxygen probe. Each assay was conducted over a 45-min duration at 21 °C. The dissolved oxygen probe was recalibrated each day and saline in the gas exchange vessel was flushed and re-filled after each experimental run.
Sparging gas study: To determine what effect respiratory gas solubility has on oxygen transport by DDFPe, nitrogen, helium, and carbon dioxide gas were used in various combinations (assays 1–5, ) to remove oxygen dissolved in the saline as well as displace atmospheric oxygen in the headspace of the gas exchange vessel. These assays were conducted under the same experimental conditions as previously mentioned.
Pre-oxygenation study: Pre-oxygenation of DDFPe was tested against simultaneous oxygenation of DDFPe in assay 6 () to observe the oxygen offloading behaviour of pre-oxygenation versus simultaneous oxygenation of DDPFe during testing. CO2 gas was used to de-gas the saline in the gas exchange vessel and displace atmospheric air in the headspace of the vessel. Pre-oxygenation was conducted by injecting 1 ml of DDFPe into 50 ml of 0.9% saline solution and infusing the diluted DDFPe with a continuous supply of oxygen (2.0 l/min) over the course of 45 min, in order to keep the amount of oxygen infused consistent with previous trials. The pre-oxygenated DDFPe dilution was then connected to the in vitro performance-testing set-up () in place of the fluid reservoir and allowed to circulate through the Silastic™ tubing within the gas exchange vessel via the same in vitro performance testing experimental set-up.
Results
Sparging gas study
shows the oxygen offloading data for all the tested samples and controls. Assay 4 (), which consisted of the He (de-gassed with He) DDFPe experimental run, resulted in the greatest net oxygen increase in the gas exchange vessel. The CO2 saline control run (assay 5; ) resulted in the lowest net oxygen increase. In the assays tested with nitrogen, it should be noted that the PTB + sucrose blank showed a moderately statistically significant increase in oxygenation from its saline control (p = .08). However, the DDFPe run from that data set also showed a statistically significant increase in oxygenation from its saline control (p = .0000123) and from the PTB + sucrose blank (p = .01). These results demonstrate that the presence of DDFPe increases oxygen offloading within the gas exchange vessel.
Figure 2. Net oxygen offloading in sparging gas study. The net increase in oxygen concentration in the gas exchange vessel for assays 1–5 (). The number of trials for each assay (from left to right) is as follows: N2 control (n = 4), N2 DDFPe (n = 6), PTB + sucrose blank (n = 2), He control (sparged with N2) (n = 4), He DDFPe (sparged with N2) (n = 5), He control (sparged with He) (n = 3), He DDFPe (sparged with He) (n = 3), CO2 control (n = 5) and CO2 DDFPe (n = 3).
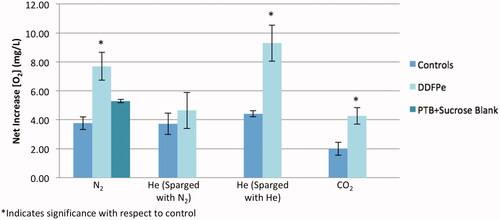
As can be inferred from , the gas used in the assay influences the net oxygen offloading. A comparison of assays 3 and 4 () demonstrates the effect of the selected sparging gas on oxygen transport. The results of these studies indicate that the magnitude of oxygen transport is inversely related to the solubility of the sparging gas used in the assay (). This is additionally reinforced by the solubility values displayed in . Assays 3 and 4 () were conducted under identical experimental conditions using helium to pressurize the vessel headspace (headspace gas defined in ), the only modified variable was the sparging gas used to initially de-gas the saline in the gas exchange vessel (defined as the sparging gas in ). The results of the DDFPe assays show a two-fold increase in the net oxygen concentration when initially de-gassed with He as opposed to N2 ( ) (p = .004). The results displayed in also demonstrate the logarithmic oxygen offloading behaviour of DDFPe due to the competing effects of the oxygen and sparging gas solubility in saline.
Figure 3. Effect of sparging gas selection on oxygen transport. The increase in oxygen concentration in the gas exchange vessel in assays 3 and 4 (), over the course of 45 min. Net increase of oxygen in the He DDFPe (sparged with N2) run compared to He DDFPe (sparged with He) run is statistically significant (p = .004).
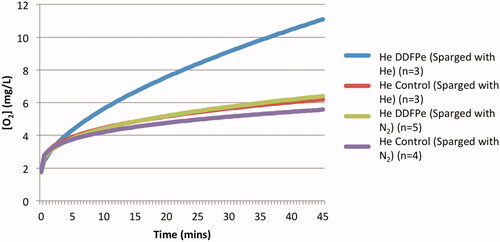
Table 2. Sparging gas solubility and net oxygenation.
Pre-oxygenation study
shows the oxygen offloading behaviour of pre-oxygenated DDFPe. The data demonstrates two types of offloading behaviour, which may indicate that the preoxygenation level of DDFPe varied with each run. Runs 2 and 4 display normal offloading behaviour, as observed when DDFPe was simultaneously oxygenated during the in vitro experimentation. Runs 1 and 3, however, display cyclic behaviour of de-oxygenation and re-oxygenation of the circulating DDFPe around an oxygen concentration of approximately 6.5 mg/l. This cyclic behaviour could be explained by DDFPe’s tendency to exchange gas based on a surrounding concentration gradient. Once all the oxygen had been offloaded from the DDFPe, the drug product may have started picking oxygen back up from the solution in the subsequent cycles through the in vitro experimental set up.
Figure 4. Oxygen offloading of pre-oxygenated DDFPe. The oxygen offloading behaviour of the pre-oxygenated DDPFe in assay 6 ().
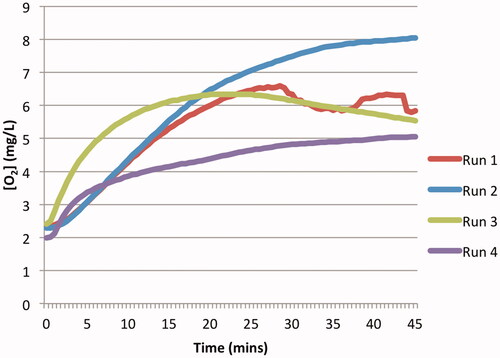
displays the average oxygen offloading behaviour of pre-oxygenated DDFPe in comparison to that of simultaneously oxygenated DDFPe from assay 6 (). The data show that the net oxygen offloading in the gas exchange vessel for both experimental groups is similar, 3.86 mg/l and 4.27 mg/l for the pre-oxygenated and simultaneously oxygenated groups, respectively. However, the pre-oxygenated experimental group displays more inter-run variability in oxygen offloading behaviour. Comparisons of the pre-oxygenated DDFPe to the simultaneously oxygenated DDFPe were not statistically significant (p = .64) indicating that there was most likely no implication in their offloading behaviour variance.
Figure 5. Average oxygen offloading under pre-oxygenation and simultaneous oxygenation of DDFPe. Comparison of pre-oxygenated and simultaneously oxygenated DDFPe oxygen offloading. The graph depicts the oxygen offloading behaviour of pre-oxygenated DDFPe assay 6 () in comparison to simultaneously oxygenated DDFPe assay 5 ().
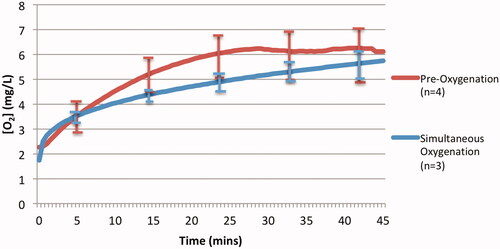
Discussion
The solubility of molecular oxygen in DDPFe is the result of the ideal gas-like chemical inertness of PFCs, which allows for the formation of CF3 group cavities within the liquid that stabilize the electron density of similarly inert gas molecules [Citation12]. The primary mechanism through which oxygen is delivered to tissues in vivo is by intracellular haemoglobin. However, in the case of haemoglobin as opposed to PFCs, oxygen molecules are chemically bound to the iron atom of a haeme molecule. Additionally, the four subunits of haemoglobin display cooperative oxygen binding behaviour due to conformational changes induced as neighbouring haeme subunits bind oxygen molecules. The difference in the nature of these interactions is reflected in the oxygen uptake curves for haemoglobin and PFCs. The sigmoidal uptake curve of haemoglobin reflects its subunit co-operativity in contrast to the linear uptake curve exhibited by PFCs [Citation9].
Various mechanisms of action have been previously theorized regarding the method through which DDFPe aids in the appreciable oxygenation of hypoxic tissues, especially when considering relatively low doses of emulsion administered in comparison to blood volume. Circulating red blood cells (RBCs) function as a bank of stored O2, which allows for a continual pressurization of surrounding plasma such that the net flux of O2 is oriented into metabolizing cells. It has been proposed that PFCs act as a “super charger” for oxygen delivery, and therefore could facilitate gas transport despite low dosages [Citation7]. Essentially, in order to reach tissues, O2 must travel from intracellular haemoglobin through blood plasma. Though the plasma is a conduit for gas transfer, its limited gas solubility can cause resistance. Consequently, due to the weak intermolecular forces experienced between PFCs and dissolved gases and the relative surface-to-volume ratio of PFCs, which is larger and more favourable for gas exchange than that of RBCs, circulating PFCs are hypothesized to act as a vehicle to facilitate transport of dissolved O2 through the resistive plasma into tissues [Citation1,Citation7].
Pre-clinical studies of DDFPe in stroke models have shown efficacy with gram weight doses of PFC < 1/200th the amount necessary for higher molecular weight PFCs [Citation19–21]. As noted above, Johnson, et al, showed that DDFPe carried approximately seven-fold more oxygen per gram than PFOBe or PFDe at 37 °C [Citation12]. The superior oxygen dissolving capability of DDFP is reflective of three main factors: CF3 group density, PFC size (MW), and PFC boiling point [Citation13]. However, the greater oxygen carrying capacity of DDFPe compared to PFOBe and PFDe only likely accounts for part of the apparently greater efficacy at low doses of PFC. Other factors that could account for the apparent increase in in vivo efficacy might include more efficient oxygen release, better delivery to the tissues or other factors. In subsequent experiments, we plan to compare the oxygen release of DDFPe to oxygen release of other PFCs at both 21 °C and 37 °C.
It is important to note that DDFPe transfers gases based on local pressure gradients [Citation12,Citation22]. The results observed in the sparging gas study regarding the inverse relationship between oxygen offloading and the solubility of the sparging gas in saline (assays 3 and 4, ) support that DDFPe oxygen transport behaviour is largely pressure gradient-based. As the sparging gas solubility increases, the local pressure gradients between the DDFPe and surrounding saline decrease in magnitude. Consequently, it becomes increasingly unfavourable for the oxygen carried by the DDFPe to dissolve into the saline. The results observed in the pre-oxygenation experiments (assay 6, ) further support the idea that DDFPe oxygen transport behaviour is largely regulated by local pressure gradients. As seen in , the results of runs 1 and 3 from assay 6 in this in vitro experimental set-up display cyclic oxygenation and de-oxygenation patterns that support the idea that DDFPe oxygenates regions of low oxygen concentrations based on localized pressure gradients. This cyclic behaviour, in conjunction with the theory that the oxygenation of hypoxic tissues by DDFPe may prompt an intracellular cascade that reverses the effects of anaerobic metabolism, may explain how in some studies the effects of oxygenation by DDFPe have manifested past the 90-min terminal half-life window [Citation23].
The results of our in vitro experimentation additionally lead us to believe that the gas preferentiality of DDFPe could play a role in its oxygen delivery in hypoxic environments. It has been previously established that the gas dissolving capacity of PFCs decreases in the order: C02 ≫ O2 > CO > N2 > H2 > He, following the decrease in molecular volume [Citation8] since van der Waals forces are the primary intermolecular forces experienced by PFCs and dissolved gases. As can be seen in , although CO2 is 3× as soluble as O2 in 0.9% saline, the in vitro experimentation still displayed a net 2.00 mg/l increase in oxygenation in assay 5. Consequently, the results of our in vitro experimentation reiterate the preferential dissolution of CO2 over O2 in DDFPe. DDFPe has been previously utilized for TBI (pre-clinically) and stroke indications (pre-clinically and clinically), both of which involve gas transport via microcirculation within the brain under hypoxic conditions. With regard to tissue hypoxia, extended durations of anaerobic respiration result in a build-up of intracellular CO2, which thereby results in increased blood CO2 levels and hypercapnia. Studies investigating dynamic brain metabolism have revealed that mild hypercapnia results in a suppression of cerebral metabolic rate of oxygen utilization and consequent loss of brain function [Citation24]. Circulating oxygenated DDFPe may then release O2 and preferentially dissolve CO2 within the brain microcirculatory system. Therefore, DDFPe’s high solubility of CO2 and increased micro-flux due to its small particle size may contribute to its efficacy at low doses as an oxygenating agent for these indications.
It should be stated that the oxygen offloading concentrations measured in this in vitro model cannot be scaled quantitatively to an in vivo situation due to the physiological limitations of an acellular, in vitro model. With regards to the oxygen offloading behaviour of PFCs, Riess et al. argues that four factors ultimately contribute to the high in vivo O2-delivery/O2-dissolution ratio exhibited by PFCs. (1) their lower viscosity allows for a greater cardiac output and net flux, (2) they result in considerably higher arterial PO2 and therefore increased diffusion rates from the capillaries to the tissues, (3) due to the weak intermolecular forces experienced between PFCs and dissolved gases, PFC-dissolved O2 is more immediately available to tissues than haemoglobin-bound O2, and is consequently characterized by high extraction ratios, and (4) their smaller particle size allows for increased micro-flux [Citation8]. The synergistic effects of these four factors allows for the efficacy of PFCs at lower doses. It is worth noting, however, that although the numerical results of this in vitro setup may not be directly scalable to in vivo results, the trends observed in this in vitro model are still biologically relevant. Future in vitro studies should aim to incorporate these four factors into a cellular, in vitro model in order to further explore in vivo mechanisms of PFC oxygen transport.
Disclosure statement
Meghna Jayaraman is an intern at NuvOx Pharma. Kaitlin Graham is an employee of NuvOx Pharma and owns stock in the company. Dr Evan Unger is President and CEO of NuvOx Pharma, serves on the Board of Directors, and owns stock in the company. Dr Unger is also a patent holder of the NuvOx Pharma technology. The authors report no other conflicts of interest in this work.
Data availability statement
All data supporting the results or analyses presented in the paper reside at NuvOx Pharma. For further information, contact the corresponding author. Meghna Jayaraman is a senior at the University of Arizona, majoring in biomedical engineering and minoring in mathematics and astronomy. She has been an intern at NuvOx Pharma for the past 2 years, where she has worked on refining an in vitro model of the human lung. As an undergraduate student researcher, she also has experience with biocompatible material characterization and pressure volume loop analysis in cardiac research. Kaitlin Graham (Harpel) is the Program Manager at NuvOx Pharma. She received her Master of Science degree in Biomedical Engineering at the University of Arizona. Her previous research experiences include oncology, microscopy, and cancer detection through the use of targeted perfluorocarbon microbubbles.
Additional information
Notes on contributors
Evan C. Unger
Dr Evan Unger, MD is co-founder, President and CEO of NuvOx Pharma, a company developing a platform of oxygen therapeutics to treat life-threatening diseases where hypoxia plays a role. Dr Unger has founded three biotech companies. His first company, ImaRx Pharmaceutical, developed three FDA approved drugs (including Definity®, the world’s #1 selling ultrasound contrast agent), and was acquired by DuPont. Dr Unger’s second company, ImaRx Therapeutics, went public and performed clinical trials in a pioneering new technology to treat stroke. Dr Unger co-founded NuvOx, in-licensed the core patents and obtained ownership of the regulatory documents for NuvOx’s key product. Dr Unger is inventor on 113 issued US patents. He is a board-certified radiologist and has an appointment as Professor of Radiology and Biomedical Engineering at the University of Arizona. He has served on the NIH gene and drug delivery and NCI’s nanotechnology study sections, and currently serves as co-Leader of the University of Arizona Cancer Center Cancer Imaging Program.
References
- Spiess BD. Perfluorocarbon emulsions as a promising technology: a review of tissue and vascular gas dynamics. J Appl Physiol (1985). 2009;106:1444–1452.
- Graham K, Moon-Massat PF, Unger EC. Military supplement: dodecafluoropentane emulsion (Ddfpe) as a resuscitation fluid for treatment of hemorrhagic shock and traumatic brain injury: a review. Shock. 2017;1. . Available from: https://journals.lww.com/shockjournal/Abstract/publishahead/2017_Military_Supplement___Dodecafluoropentane.97932.aspx
- Mullah SH, Saha BK, Abutarboush R, et al. Perfluorocarbon NVX-108 increased cerebral oxygen tension after traumatic brain injury in rats. Brain Res. 2016;1634:132–139.
- Cheung AT, To PL, Chan DM, et al. Comparison of treatment modalities for hemorrhagic shock. Artif Cells Blood Substit Immobil Biotechnol. 2007;35:17390.
- Remy B, Deby-Dupont G, Lamy M. Red blood cell substitutes: fluorocarbon emulsions and haemoglobin solutions. Br Med Bull. 1999;55:277–298.
- Riess JG. Understanding the fundamentals of perfluorocarbons and perfluorocarbon emulsions relevant to in vivo oxygen delivery. Artif Cells Blood Substit Immobil Biotechnol. 2005;33:47–63.
- Spiess BD. Basic mechanisms of gas transport and past research using perfluorocarbons. Diving Hyperb Med. 2010;40:23–28.
- Jean G, Riess MLB. Solubility and transport phenomena in perfluorochemicals relevant to blood substitution and other biomedical applications. Pure Appl Chem. 1982;54:2383–2406.
- Riess JG. Perfluorocarbon-based oxygen delivery. Artif Cells Blood Substit Immobil Biotechnol. 2006;34:567–580.
- Grayburn P. Perflenapent emulsion (EchoGen): a new long-acting phase-shift agent for contrast echocardiography. Clin Cardiol. 1997;20:12–18.
- Unger EC. A phase Ib/II clinical trial of a novel oxygen therapeutic in chemoradiation of glioblastoma [Poster Presentation]. 2017 American Society of Clinical Oncology (ASCO) Annual Meeting 2017 June 5, 2017.
- Johnson JL, Dolezal MC, Kerschen A, et al. In vitro comparison of dodecafluoropentane (DDFP), perfluorodecalin (PFD), and perfluoroctylbromide (PFOB) in the facilitation of oxygen exchange. Artif Cells Blood Substit Immobil Biotechnol. 2009;37:156–162.
- Johnson JLH. Oxygen carriers: are they enough for cellular support? In: Lapchak PA, Zhang JH, editors. Neuroprotective therapy for stroke and ischemic disease. Cham, Switzerland: Springer International Publishing; 2017. p. 621–640.
- Lundgren CE, Bergoe GW, Tyssebotn IM. Intravascular fluorocarbon-stabilized microbubbles protect against fatal anemia in rats. Artif Cells Blood Substit Immobil Biotechnol. 2006;34:473–486.
- Torres Filho IP, Pedro JR, Narayanan SV, et al. Perfluorocarbon emulsion improves oxygen transport of normal and sickle cell human blood in vitro. J Biomed Mater Res. 2014;102:2105–2115.
- Hamilton RL, Berry MN, Williams MC, et al. A simple and inexpensive membrane "lung" for small organ perfusion. J Lipid Res. 1974;15:182–186.
- Ferguson ER, Clymer JJ, Spruell RD, et al. Perfluorocarbon oxygen transport. A comparative study of four oxygenator designs. Asaio J. 1994;40:M649–M653.
- Lundgren C, Bergoe G, Olszowka A, et al. Tissue nitrogen elimination in oxygen-breathing pigs is enhanced by fluorocarbon-derived intravascular micro-bubbles. Undersea Hyperb Med. 2005;32:215–226.
- Culp WC, Woods SD, Skinner RD, et al. Dodecafluoropentane emulsion decreases infarct volume in a rabbit ischemic stroke model. J Vasc Int Radiol: JVIR. 2012;23:116–121.
- Woods SD, Skinner RD, Ricca AM, et al. Progress in dodecafluoropentane emulsion as a neuroprotective agent in a rabbit stroke model. Mol Neurobiol. 2013;48:363–367.
- Brown AT, Arthur MC, Nix JS, et al. Dodecafluoropentane emulsion (DDFPe) decreases stroke size and improves neurological scores in a permanent occlusion rat stroke model. Toneuj. 2014;8:27–33.
- Van Liew HD, Burkard ME. Bubbles in circulating blood: stabilization and simulations of cyclic changes of size and content. J Appl Physiol (1985). 1995;79:1379–1385.
- Swyer TW, Strom J, Larson DF. Nanoparticle oxygen delivery to the ischemic heart. Perfusion. 2014;29:539–543.
- Xu F, Uh J, Brier MR, et al. The influence of carbon dioxide on brain activity and metabolism in conscious humans. J Cereb Blood Flow Metab. 2011;31:58–67.
- Solubility of Gases in Water [online]. Engineering ToolBox; 2008 [cited 2018, July 6]. Available from:https://www.engineeringtoolbox.com/gases-solubility-water-d_1148.html