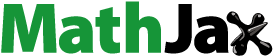
Abstract
Conventional anti-tumour chemotherapy is facing the challenges of poor specificity, high toxicity and drug resistance. Tumour microenvironment (TME) plays a critical role in tumour development and drug resistance. To address this problem, we constructed a novel anti-tumour nanoparticle platform RBC@BPQDs-DOX/KIR, black phosphorus nanoparticle quantum dots (BPQDs) with one of the chemotherapeutics (doxorubicin, DOX) and an anti-inflammatory traditional Chinese medicine active component (Kirenol, KIR). Red blood cell membrane (RBCm) vesicles were used as the shell to envelop several nanocores. The combination of DOX and KIR may promote therapeutic efficacy, at which the anti-apoptotic effect of the tumour cells was inhibited (by downregulating Bcl-2 and upregulating Bax) and the tumour progression-related inflammatory factors, such as tumour necrosis factor α (TNF-α) and interleukin-6 (IL-6) were downregulated. Furthermore, TME was remodelled and the anti-tumour effect of DOX was magnified. RBCm imparts high biocompatibility and enhanced permeability and retention (EPR) effects to RBC@BPQDs-DOX/KIR, thus enhancing its tumour passively targetability. Overall, the RBCm-camouflaged drug delivery system RBC@BPQDs-DOX/KIR as a promising therapy for targeted chemotherapeutics and anti-inflammatory therapeutics may provide a specific and highly efficient anti-tumour treatment choice.
Introduction
For tumour therapy, chemotherapy remains one of the most common and effective anti-tumour treatments, though some of the limitations still exist, such as poor specificity, high clearance rate, low drug accumulation in tumours and drug resistance [Citation1,Citation2]. To address these issues, nanodrug delivery system has been developed in the past decades. By loading the chemotherapeutics into nanocarriers, the drug bioavailability can be increased while the toxic side effects are minimized [Citation3]. Due to these merits, varieties of nanocarriers have been constructed, including liposomes, polymer nanocomposites, quantum dots and metal derived nanoparticles.
On the one hand, tumour microenvironment (TME) is closely related to drug resistance of tumours. The formation of TME involves several types of immune cells and inflammatory factors [Citation4]. Inflammation as one critical microenvironment factor is popular in tumours. Accumulating evidences from present studies demonstrate that inflammation also existed in different stages of tumour development, and become a dominant hallmark [Citation5,Citation6]. Under tumour inflammatory microenvironment, the infiltration of immune cells promotes tumourigenesis, invasion and metastasis through releasing survival and growth factors such as tumour necrosis factor α (TNF-α) and interleukin-6 (IL-6) [Citation7,Citation8]. On the other hand, inflammation can attenuate the host immune response against tumours through suppressing tumour immuno-surveillance and immuno-editing [Citation9]. Despite the aforementioned merits of nanodrugs, most of them as foreign agent may activate immune systems to induce inflammatory responses. For chemotherapy, many tumours remain resistance for DOX [Citation10], one major reason is that the dying cells after therapy are readily to stimulate immune cells with pro-inflammatory effect, further to promote the resistance to chemotherapy [Citation11,Citation12]. All these two aspects would promote inflammatory response, causing tumour regeneration and eventually therapy failure. Therefore, anti-inflammation therapy in accompany with antitumour therapy is emerging as a progressive strategy for cancer treatment. However, there are short of effective nanocarriers simultaneously carrying out anti-inflammatory and anti-tumour treatments, and the carrier itself may exacerbate inflammation.
Kirenol (KIR) is a bioactive component extracted from Herba Siegesbeckiae, belonging to diterpenoid (). KIR as a traditional Chinese herbal medicine for rheumatoid arthritis can reduce the level of inflammatory factors. Recent studies have demonstrated anti-photoaging effects of KIR through inhibiting inflammation signalling pathways [Citation13]. Therefore, KIR may be an ideal natural compound for inhibiting inflammation and magnifying the effect of conventional chemotherapeutics.
Figure 1. Schematic illustration of RBC@BPQDs-DOX/KIR fabrication and application for tumour-targeted chemotherapy and anti-inflammatory therapy in mice.
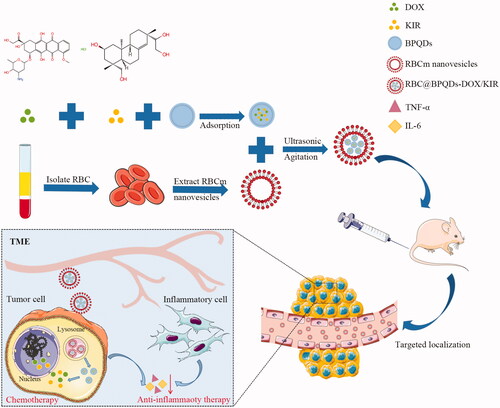
In recent years, some types of cell membrane-derived biomimetic nanomaterials bring extra advantages. Natural protein could be grafted on nanovesicles, biocompatible without consideration of toxicity [Citation14,Citation15]. Therefore, various types of cell membrane have been used to deliver nanocarriers for promoting biocompatibility and targetability in recent years, including red blood cell membrane (RBCm), white blood cell membrane, tumour cell membrane and bacterial cell membrane [Citation16–19]. Furthermore, RBCm abundant with CD47 membrane protein as a self-marker can be utilized to camouflage the nanoparticles as the host cells to evade the immune system [Citation20,Citation21], giving long blood circulation time. This is crucial for increasing the enhanced permeability and retention (EPR) effect in tumour targeting-ability [Citation22,Citation23]. Moreover, red blood cell-camouflaged nanoparticles are easy to prepare and have become the most widely used biomimetic strategy. However, drugs are ready to leak when cell membrane-derived vesicles were used as nanocarriers, resulting in upsetting loading capacity.
BP attracts an increasing attention because of its unique physical structure and special properties, including high drug-loading capacity, excellent photothermal and photodynamic features. These make BP to be an ideal candidate carrier for drug delivery and anti-tumour therapy [Citation24,Citation25]. Especially, black phosphorus nanoparticle quantum dots (BPQDs) with good biocompatibility and outstanding drug loading capacity excel in the therapy of cancer [Citation26].
Inspired by the advantages of BPQDs nanomaterials, herein, we constructed RBCm camouflaging BPQDs (RBC@BPQDs) as drug carriers for synergistic chemo-/anti-inflammatory therapy of cancer. DOX and KIR were employed as antitumour and anti-inflammatory drugs, respectively. With BPQDs as trapper, both DOX and KIR could be efficiently loaded into RBC@BPQDs nanocomposites with high loading capacity. RBCm camouflage enables RBC@BPQDs to evade immuno-recognition. Upon accumulation at tumours through EPR effect, weak acid environment of tumour trigger drug release from RBC@BPQDs nanocomposites at tumour sites. The combination of DOX and KIR normalizes inflammatory TME and therefore synergistically improves the total anti-tumour efficacy (). We believe RBC@BPQDs nanocomposites with high drug-loading capacity, immune evading property, anti-inflammatory and antitumour activity will have great potential for future clinical applications.
Materials and methods
Materials
Black Phosphorus Quantum Dots Dispersion (XF208) was manufactured by Nanjing XFNANO Materials Tech Co., Ltd. (Nanjing, China). Kirenol was purchased from Desite Biotech Co., Ltd. (Chengdu, China). Doxorubicin (DOX) hydrochloride was manufactured by Sigma-Aldrich (St. Louis, MO). Cy5, rhodamine B (RhB), Hoechst 33342 and LysoTracker Green DND-26 were purchased from Yeasen Biotechnology (Shanghai, China). Dialysis membrane (2 kDa) was obtained from Solarbio (Beijing, China). Annexin V-FITC/propidium (PI) Apoptosis Detection Kit was purchased from KeyGEN BioTECH (Nanjing, China). Cell Counting Kit-8 (CCK-8) was purchased from Dojindo Laboratories (Kumamoto, Japan), penicillin and streptomycin cocktail, foetal bovine serum (FBS), DMEM (high glucose), RPMI-1640 and trypsin EDTA were from Life Technologies (Carlsbad, CA). Anti-Bax, anti-Bcl-2, anti-TNF-α, anti-IL-6 and HRP goat anti-rabbit IgG secondary antibodies were manufactured by Proteintech (Rosemont, IL), cy3 goat anti-IgG, FITC goat anti-IgG Secondary antibodies, DAPI were from Wuhan Servicebio Technology Co., Ltd. (Wuhan, China). Polycarbonate porous membrane syringe filters (200 nm) were provided by Whatman (Palo Alto, CA). Pre-stained protein Ladder was from Thermo Scientific (Waltham, MA). TNF-α Elisa kit and IL-6 Elisa kit were purchased from Abclone Co., Ltd. (Wuhan, China).
Cell lines and murine xenograft models
Cervical cancer models were constructed using the method described in the literature [Citation27]. Human cervical cancer cell lines Hela was donated by Cancer Research Institute, Central South University. The cells were cultured in DMEM (high glucose) containing 10% FBS, 1% penicillin (100 U/mL) and streptomycin (100 μg/mL). RAW264.7 cells were cultured in RPMI-1640 medium containing 10% FBS, 0.5% penicillin and streptomycin. All cells were placed at 37 °C in a 5% CO2 incubator. Six-week-old male BalB/c-nu mice were manufactured by Hunan SJA Laboratory Animal Co., Ltd. (Changsha, China). The murine xenograft models of Hela tumour cells were constructed according to the previously described [Citation28].
Preparation of RBC membrane vesicles
Whole blood with heparin anticoagulation from male BalB/c-nu mice was used. RBCm was extracted according to the previously mentioned steps with minor change [Citation29]. Red blood cells were obtained by centrifugation and washing, which were broken by the hypotonic method. These cells were re-suspended in PBS and ultrasonically treated (2 min, 42 kHz, 100 W) to obtain RBCm vesicles of about 100 nm.
Preparation of BPQDs-DOX/KIR
BPQDs (10 mg), DOX (4 nmol) and KIR (80 nmol) were simultaneously dissolved in PBS (2 mL). After stirring for 24 h at room temperature, free DOX and KIR were removed through dialysis. The dialysate was collected for determination of DOX and KIR concentrations. As a comparison, RBCm vesicles-loaded DOX was prepared as previously described. The encapsulation efficiency (EE) and load efficiency (LE) were calculated for both drugs, respectively.
Construction of RBC@BPQDs-DOX/KIR
RBCm vesicles were separated, and then fused with an equal volume of BPQDs-DOX/KIR by ultrasonic treatment (5 min, 42 kHz, 100 W). After that, they were passed through 200 nm porous membrane syringe filters for 20 times. Excess RBCm was removed by centrifugation (2500 rpm, 10 min, 4 °C), and RBC@BPQDs-DOX/KIR was obtained.
Characterization of RBC@BPQDs-DOX/KIR
RBC@BPQDs morphology and size were observed under the transmission electron microscope (TEM) (Tecnai G2 Spirit, FEI, Hillsboro, OR). For determining whether the RBCm encapsulated the nanoparticles, the nanoparticle size and surface charge were measured by Zetasizer Nano ZS (Malvern Nano series, Malvern, UK). The absorbance of RBC@BPQDs-DOX/KIR was measured by UV/Vis spectroscopy (Scandrop, Analytik, Jena, Germany).
DOX and KIR release features of RBC@BPQDs-DOX/KIR under different conditions
One millilitre RBC@BPQDs-DOX/KIR (DOX 2 μM, KIR 40 μM) was placed on dialysis membrane. Dialysis was performed at 37 °C in 20 mL PBS with pH 7.4 and pH 5.4, respectively. The absorbance of DOX in the dialysate was measured using EnSpire 2300 Multilabel Plate Reader (Waltham, MA) at 480 nm, while KIR concentration of the dialysate was determined by deoxidizing K2MnO4 (1 mg/mL) in mixed solution at 545 nm. Cumulative release amount of DOX and KIR was estimated from the standard curve, respectively.
Biocompatibility of RBC@BPQDs
Biocompatibility of RBC@BPQDs was estimated, based on haemolytic rates. RBC@BPQDs (12.5–100 μg/mL) of various concentrations was mixed with the 5% mouse red blood cells suspension. After incubation at 37 °C for 2 h, the solution was centrifuged at 3500 rpm for 5 min. The supernatant was collected and its absorbance was measured using a microplate reader at 545 nm. Ultra-pure water and PBS were added as positive control and negative control, respectively. The haemolytic rate was calculated as follows:
To determine the ability of RBC@BPQDs to evade immune response, RAW264.7 cells were inoculated to the six-well plate at a density of about 3 × 105 cells per well. RBC@BPQDs-RhB was added to incubate RAW 264.7 macrophages for 24 h. The nuclei of RAW264.7 macrophages were stained by Hoechst 33342. The phagocytosis of RBC@BPQDs by macrophages and the fluorescence intensity were observed under confocal fluorescence microscope (LSM 800, Carl Zeiss, Oberkochen, Germany).
Evaluation of in vitro anti-inflammatory effects of RBC@BPQDs-DOX/KIR
Pro-inflammatory cytokines secreted from macrophages were assessed by Elisa. The Raw264.7 cells inoculated to a six-well plate were pretreated with PBS, RBC@BPQDs, KIR and RBC@BPQDs-KIR at KIR dose of 10 μM to 40 μM for 12 h, and then added LPS (1 μg/mL) to each well. After 24 h, the culture supernatants of cells were collected by centrifugation (1000×g) for 10 min. The level of TNF-α and IL-6 in culture supernatant was measured according to manufacturer’s instruction. The percentage of cytokine was calculated as follows:
Evaluation of in vitro anti-tumour effects of RBC@BPQDs-DOX/KIR
Cytotoxicity of RBC@BPQDs-DOX/KIR on Hela cells was assessed using CCK-8 assay. These cells were inoculated to a 96-well plate at a density of 2 × 103 cells per well. After incubation for 24 h, Hela cells were treated with PBS, BPQDs, DOX, DOX + KIR, BPQDs-DOX/KIR and RBC@BPQDs-DOX/KIR, respectively. DOX of each group was at 2 μM concentration; meanwhile, the concentration of KIR was 40 μM. Then, 10 μL of CCK-8 was added into each well to further incubate the cells at 37 °C for 3 h in a 5%CO2 incubator. EnSpire 2300 Multilabel Plate Reader was used to detect the absorbance value at 450 nm. To further determine the in vitro anti-tumour effect of RBC@BPQDs-DOX/KIR, Annexin V-FITC Apoptosis Detection Kit was used to detect the apoptosis of Hela cells. To this end, the cells were inoculated to a small culture flask (1 × 106/flask), and the same treatments described above were used. After 24 h, these cells were digested with EDTA-free trypsin. The rates of cell apoptosis were detected using flow cytometry (FACSCantoTM II, BD, San Jose, CA) according to the instruction.
RBC@BPQDs distribution assay in vivo
To evaluate the targeting capability of RBC@BPQDs in vivo, cy-5 labelled RBC@BPQDs was lined-up by mixing BPQDs with cy-5, then, the murine xenograft model was injected intravenously with the cy-5 labelled RBC@BPQDs via tail vein at cy5 dose of 2 μg/kg. The fluorescence intensity was detected on Xenogen IVIS lumina XR imaging system (Caliper Life Science, Hopkinton, MA) at 6, 24 and 48 h post-administration. Afterwards, all the mice were euthanized; the tumours and major organs were gleaned and subjected for ex vivo further determination.
RBC@BPQDs-DOX/KIR treatment in cervical cancer-bearing mice
Day 0 (D0) was defined as the time when the tumour volume was about 100 mm3. The mice were randomly divided into six groups (n = 5) and received tail intravenous injection of 100 μL PBS, BPQDs, DOX, DOX + KIR, BPQDs-DOX/KIR and RBC@BPQDs-DOX/KIR, once a day for three days consecutively. The dose of DOX was 1 mg/kg/d, and KIR dosage of each group was 20 mg/kg/d. The tumour size and body weight of all the mice were measured once every two days. All the mice were sacrificed under anaesthesia on the 14th day (D14). Whole blood, tumours and major organs (the heart, liver, spleen, lungs and kidneys) were harvested. Whole blood samples were added with EDTA anticoagulant, and the level of blood cells was detected by five-part differential hematology analyzer (BC-5390, Mindray, Shenzhen, China). The enzyme level in serum was measured by automatic biochemical analyzer (7100, HITACHI, Tokyo, Japan) after centrifugation at 3000 rpm for 10 min. The frozen tumour tissue samples were sliced into sections. After counterstaining with DAPI, the fluorescence intensity of these sections was observed through confocal fluorescence microscope in the mice treated with RBC@BPQDs-DOX/KIR. Western blotting was used to determine total protein extracts of fresh tumour tissue samples. The primary antibodies used were TNF-α and IL-6. All harvested organs and tumours were fixed in 4% paraformaldehyde, embedded in paraffin and made into sections. These sections were stained with H&E staining and immunofluorescence staining.
Immunofluorescence staining
Paraffin-embedded tumour tissues were deparaffinized and subjected to antigen retrieval. Immunofluorescence staining was performed using anti-TNF-α and anti-IL-6 according to the standard protocol. Later, tumour cells were counterstained with DAPI. Tumour cells were observed under the fluorescence microscope and images were taken.
Western blotting analysis
Total proteins were extracted from cell lysate using RIPA buffer. Total protein concentrations were determined with BCA protein assay kit. Protein expressions of Bax and Bcl-2 in Hela cells and those of TNF-α and IL-6 in tumour tissues were detected by western blotting according to the standard protocol.
Statistical analysis
SPSS Software13.0 was used for statistical analysis (SPSS Inc., Chicago, IL). Data were expressed as mean ± SD. Intergroup differences were assessed by one-way ANOVA, followed by Tukey’s post-test. Data were considered statistically significant for p< .05.
Results and discussion
Preparation and characterization
To prepare RBC@BPQDs-DOX/KIR nanocomposites, DOX and KIR were loaded into BPQDs to obtain BPQDs-DOX/KIR. Afterwards, BPQDs-DOX/KIR was encapsulated into RBCm nanovesicles under ultrasound assistance. As can be seen from TEM micrographs (), BPQDs were monodispersed with average diameter around 10 nm. According to dynamic light scattering (DLS) analysis (), the average size of RBC@BPQDs nanocomposites was 63.0 ± 1.6 nm (close to the particle size of 62.5 ± 3.3 nm with RBCm). The zeta potential of BPQDs was –22.3 ± 1.2 mV; while after the encapsulation, the zeta potential of RBC@BPQDs nanocomposites was decreased to –27.2 ± 0.9 mV, closing to that of RBC vesicles (–27.3 ± 2.0 mV). The result of SDS-PAGE () reveals that almost all the RBC membrane proteins were preserved in RBC@BPQDs nanocomposites. According to UV–Vis spectrometry (), RBC@BPQDs-DOX/KIR had absorption peaks at 196 nm, 210 nm, 410 nm and 500 nm, respectively, which agreed with those of BPQDs, KIR, RBC membrane vesicles and DOX detected alone. This further demonstrated the successful assembly of RBC@BPQDs-DOX/KIR. To confirm whether KIR was successfully loaded into BPQDs or not, we measured the release liquid of RBC@BPQDs-DOX/KIR under 545 nm through K2MnO4 oxidization of the hydroxyl of KIR.
Figure 2. Characterization of the RBC@BPQDs-DOX/KIR. (A) The TEM micrographs of nanovehicles. The scale bar: 50 nm. (B) The particle size and zeta potential of BPQDs after coating with RBCm. (C) SDS-PAGE protein analysis. (D) UV–Vis spectra of BPQDs, KIR, DOX, RBCm vesicles and RBC@BPQDs-DOX/KIR. (a) BPQDs, (b) RBCm vesicles and (c) RBC@BPQDs.
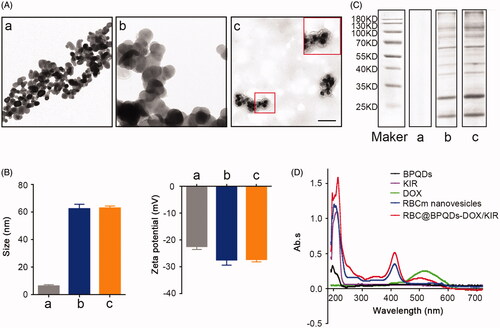
Drug loading and release
The drug loading capacity of RBC@BPQDs nanocomposites were measured with RBCm nanovesicles for comparison. Only 10.2% DOX and 4.1% KIR were encapsulated within the nanovesicles (Figure S1). The data in the present study suggest that both DOX and KIR as small molecules are ready to leak from the nanovesicles, giving rise to poor drug loading capacities. By contrast, for RBC@BPQDs nanocomposites, BPQDs abundant with phosphate groups on their surface could interact with drug molecules through hydrogen bonds or electrostatic interactions. Therefore, with BPQDs as drug trappers, the drug loading capacities of RBC@BPQDs-DOX/KIR nanocomposites were 96.3% (DOX) and 42.0% (KIR), respectively (). Next, we investigated the drug release profile of RBC@BPQDs-DOX/KIR nanocomposites (). At pH7.4, only about 4% of DOX and 18% of KIR were released from RBC@BPQDs-DOX/KIR and BPQDs-DOX/KIR within 8 h, respectively; and about 9% of DOX and 24% of KIR were released within 48 h, respectively. However, a higher cumulative release rate was achieved for RBC@BPQDs-DOX/KIR and BPQDs-DOX/KIR at pH 5.4. Within 8 h, about 28% of DOX and 38% of KIR were released from RBC@BPQDs-DOX/KIR and BPQDs-DOX/KIR, respectively, in 48 h, about 74% of DOX and 76% of KIR were released, respectively. Since the tumour environment is a weak acid, the pH responsive drug release profiles of RBC@BPQDs-DOX/KIR nanocomposites are favourable for drug release in tumours. In addition, RBCm encapsulation might slightly reduce the release rate of DOX and KIR, suggesting that RBCm inhibited the explosive release of the drug from BPQDs to a certain extent, which was the sustained-release effect. In a word, the in vitro release experiments of DOX and KIR proved that an acidic environment facilitated the dissociation of RBC@BPQDs-DOX/KIR and the release of the two loaded drugs from the carrier. This was a convincing evidence that RBC@BPQDs-DOX/KIR released anti-tumour drug in the acidic TME.
Biocompatibility studies
The biocompatibility of RBC@BPQDs nanocomposites was investigated using anti-phagocytosis and haemolysis assays. Both BPQDs and RBC@BPQDs nanocomposites were labelled with RhB (red fluorescence). RAW264.7 macrophages were treated with RBC@BPQDs nanocomposites for 24 h. Meanwhile, BPQDs without RBCm camouflage was taken for comparison. As can be seen from , all the macrophages present bright red fluorescence when treated with BPQDs-RhB, indicating the intensive phagocytosis by macrophages. By sharp contrast, faint fluorescence of RBC@BPQDs-RhB nanocomposites was observed under the same condition, i.e. the phagocytosis was efficiently inhibited. The results indicate that RBCm coating endow the nanocomposites with immune evading ability, which will reduce their clearance from the body. Besides, the haemolysis rate induced by RBC@BPQDs nanocomposites was less than 1% (). Therefore, all these results suggest the excellent biocompatibility of RBC@BPQDs nanocomposites.
Figure 4. The biocompatibility of RBC@BPQDs-DOX/KIR. (A) CLSM micrographs of macrophages after cultured with BPQDs-RhB and RBC@BPQDs-RhB for 4 h. The scale bar: 20 μm. (B) Fluorescence intensities of collected cells after treatment with BPQDs-RhB and RBC@BPQDs-RhB, as quantified by a fluorometre. (C) Haemolysis rate of RBCs at various concentrations of BPQDs and RBC@BPQDs at 37 °C after 2 h. Data are mean ± SD (n = 3). **p<.01.
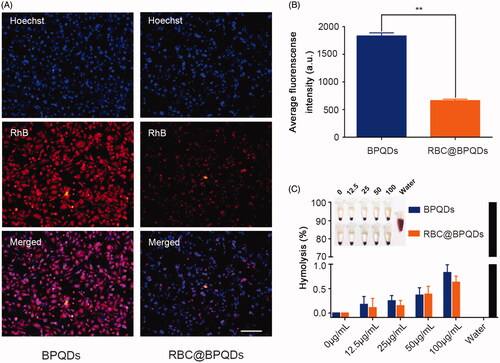
In vitro anti-inflammatory effects
It is well documented that both tumour cells and immune cells are involved in the inflammatory process of tumour. Some pro-inflammatory cytokines, such as TNF-α and IL-6 are closely associated with tumour development, metastasis and drug resistance [Citation30]. Therefore, it was necessary to evaluate the anti-inflammation activity of RBC@BPQDs-KIR nanocomposites. Macrophages were stimulated by lipopolysaccharides (LPSs) to induce inflammation. In Figure S2, after LPS stimulation, the secretion level of TNF-α and IL-6 in the supernatant of macrophages was significantly upregulated, indicating the successful construction of in vitro inflammation model. KIR, as a bioactive anti-inflammatory ingredient, substantially decreased the secretions of TNF-α and IL-6 by 30.7% and 28.6% (Figure S2), respectively. The anti-inflammatory activity of RBC@BPQDs-KIR nanocomposites was close to that of KIR. Therefore, it can be concluded that RBC@BPQDs-KIR nanocomposites are capable to attenuate inflammation through KIR release.
In vitro anti-tumour therapy
Considering the critical role of inflammation in tumour development, we hypothesise that the combination of anti-inflammatory therapy and chemo-therapy would synergistically enhance the final antitumour efficacy. The in vitro anti-tumour performance of RBC@BPQDs-DOX/KIR nanocomposites was evaluated through cytotoxicity and apoptotic detections. From the results in , RBC@BPQDs-DOX/KIR possesses significant cytotoxicity against HeLa cells. For the chemotherapy of free DOX, the cell viability was only decreased by 24.3% (). By contrast, when DOX was combined with KIR, 30.9% cells were killed. It is noteworthy that KIR itself possesses little cytotoxicity to HeLa cells (Figure S3). Similarly, for RBC@BPQDs-DOX/KIR nanocomposites, the cell viability was slightly decreased to 33.4%. To further confirm the cytotoxicity results, cell apoptosis after the treatments was analysed through flow cytometry. As shown in , after the treatment of RBC@BPQDs-DOX/KIR nanocomposites, the early and late apoptotic cells account for 41.7% and 24.0%, respectively, which are much higher than those of other groups. Therefore, RBC@BPQDs-DOX/KIR nanocomposites combining chemotherapy and anti-inflammatory therapy may own a better anti-tumour effect than DOX-based chemotherapy alone.
Figure 5. In vitro anti-tumour efficiency of RBC@BPQDs-DOX/KIR. (A) Cell viability and (B) analysis of apoptosis rate by flow cytometry of Hela cells treated with PBS, BPQDs, DOX, DOX + KIR, BPQDs-DOX/KIR and RBC@BPQDs-DOX/KIR at the DOX concentration of 2 μM and KIR concentration of 40 μM for 24 h. (C) The western blotting and (D) semi-quantitative analysis of Bax and Bcl-2 expression in Hela cells treated with different scheme as above for 24 h. Data are mean ± SD (n = 3). **p<.01 vs. control. ##p<.01.
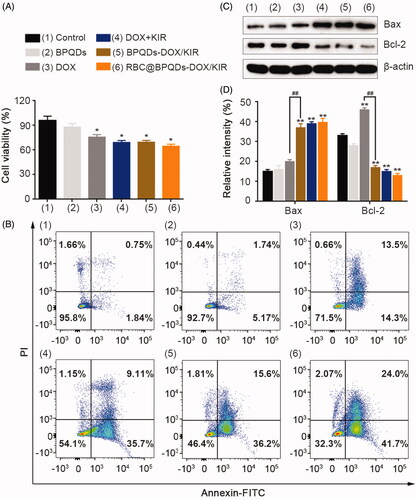
To understand the underlying antitumour mechanism, the expressions of apoptosis-related proteins were analysed via western blotting. As shown in , DOX caused an upregulation of protein expression of Bax and Bcl-2 by 33% and 39%, respectively. It is well documented that upregulating anti-apoptotic protein Bcl-2 is a common mechanism in tumours to evade apoptosis [Citation31]. With the combination of anti-inflammatory ingredient, KIR, the protein expression of Bax further increased by 85%, while Bcl-2 protein expression was significantly decreased by 63%. Thus, the combination of KIR could significantly inhibit Bcl-2 expression, which synergistically enhances the chemotherapy of DOX [Citation32,Citation33].
Distribution of RBC@BPQDs after intravenous injection
RBC@BPQDs nanocomposites with immune evading ability were expected to accumulate at tumours through EPR effect. To confirm our hypothesis, RBC@BPQDs nanocomposites were labelled with NIR fluorescent dye Cy5, and their biodistribution was measured through NIR fluorescence imaging. Meanwhile, BPQDs was taken for comparison. The results in show that the fluorescence intensity at tumour sites was gradually increased, indicating the accumulation of RBC@BPQDs nanocomposites. At 48 h post intravenous injection, the retention of RBC@BPQDs nanocomposites in tumour was 4.25 times higher than that of BPQDs. Moreover, the accumulation of RBC@BPQDs nanocomposites in other organs decreased significantly as well (,C)). Furthermore, for mice treated with RBC@BPQDs nanocomposites, bright fluorescence of DOX can be observed in tumour cells. The fluorescence intensity was 5.25 times higher than that of mice treated by BPQDs, confirming the high drug delivery efficiency of RBC@BPQDs nanocomposites. These results were consistent with the findings of fluorescence images of tumour tissues slide ().
Figure 6. In vivo targeting ability of RBC@BPQDs. (A) In vivo fluorescence images of Hela xenograft model at 6 h, 24 h and 48 h after intravenous injection of cy5-labeled RBC@BPQDs and BPQDs. (B) Ex vivo bioluminescent images of the main organs and tumours at 48 h post injection. (C) Semi-quantitative analysis of fluorescence intensity from tumours and other tissues. (D) Fluorescence micrographs of tumour sections. The scale bar: 50 μm. (a) Cy5-labeled BPQDs and (b) Cy5-labeled RBC@BPQDs. 1: heart, 2: liver, 3: spleen, 4: lung, 5: kidney and 6: tumour. Data are mean ± SD (n = 3). **p<.01.
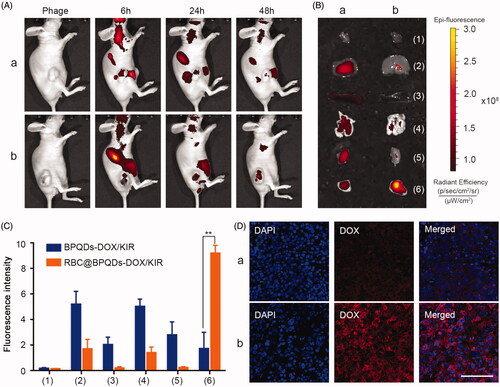
Anti-inflammatory activity in vivo
The in vivo anti-inflammatory activity of RBC@BPQDs-DOX/KIR nanocomposites was studied through immunofluorescence assay and western blotting analysis. For tumour without any treatment, strong fluorescence signals of TNF-α and IL-6 can be clearly observed, indicating their high expression. TNF-α and IL-6 expression levels in RBC@BPQDs-DOX/KIR group were significantly lower than those of the conventional chemotherapy group and control group (). Consistent with previous reports, after chemotherapy with DOX, TNF-α and IL-6 was upregulated in the tumour through NF-κB pathway, which promotes tumour cells survival [Citation34,Citation35] and lead to therapeutic resistance. After conventional chemotherapy with DOX, TNF-α (green fluorescence) and IL-6 (red fluorescence) was upregulated in the tumour, which is consistent with the study of Dash et al. [Citation36] and Zhang et al. [Citation37]. As shown in , protein expressions of TNF-α and IL-6 in the tumour of the mice treated with RBC@BPQDs-DOX/KIR was significantly decreased at the end of the observation compared with those in the control group (p< .01). Interestingly, we found, DOX treatment alone, without the component of RBC@BPQDs and (or) KIR, upregulated protein expression of TNF-α and IL-6 in tumour tissue samples (,C), p< .01). These results indicated that the DOX and KIR combined treatment altered TME and significantly inhibited the expressions of tumour-related inflammatory cytokines, including TNF-α and IL-6. It has been found that the tumour-associated macrophages, which are the immunocyte in TME, may secrete pro-inflammatory cytokines such as TNF. TNF may activate NF-κB pathway with further promotion of tumour development and metastasis. Besides, the tumour cells-secreted TNF may turn the normal fibroblasts into the cancer-associated fibroblasts (CAFs) [Citation38], which may play crucial roles in tumour deterioration and drug resistance. TNF-α is also a key molecule mediating the cardiotoxicity, lymphocyte suppression and chemotherapy-induced cognitive impairment (CICI) exerted by DOX [Citation36,Citation39]. Others have reported the IL-6, which is secreted by kinds of excessive proliferated tumour cells including cervical cancer cells [Citation40], may promote aggressive tumour growth. In summary, accumulated line of evidence indicates that these two factors play an important role in promoting tumour invasion, metastasis and angiogenesis. Moreover, conventional chemotherapeutic DOX generally increases the expression of TNF-α and IL-6, which are the important regulatory factors connecting cancer and inflammation. In contrast, we constructed the new chemotherapy combined with anti-inflammatory therapy may reduce the expression of TNF-α and IL-6 significantly. This provides a mechanistic explanation why RBC@BPQDs-DOX/KIR combining chemotherapeutics and anti-inflammatory ingredients not only magnifies the anti-tumour effect, but also reduces the toxic and side effects.
Figure 7. Anti-inflammatory effect of RBC@BPQDs-DOX/KIR. (A) The CLSM micrographs of tumour region after treated with different formulations at D14. Red: anti-IL-6, green: anti-TNF-α, blue: nucleus. The scale bar: 50 μm. (B) The western blotting test and (D) semi-quantitative analysis of the TNF-α and IL-6 expression in tumour tissues. Data are mean ± SD (n = 3). **p<.01. (Color online.)
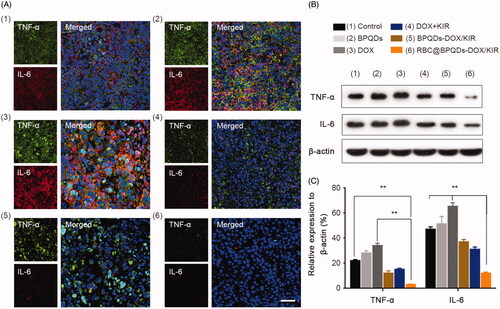
Anti-tumour effects of RBC@BPQDs-DOX/KIR in vivo
Encouraged by the extraordinary properties of RBC@BPQDs-DOX/KIR nanocomposites, we then performed in vivo studies in murine xenograft model. As shown in , the tumour growth rate of BPQDs group was almost same as that of control. Despite the ability of DOX to kill tumour cells, the tumour size of the mice treated with DOX alone was 4-fold increase after 14 days. Compared with DOX alone, the combination of DOX with KIR caused a considerable decrease of tumour growth. This further demonstrated the synergistic action between DOX and KIR in magnifying the anti-tumour effect. However, free drugs from blood circulation are likely to be cleared. The highest anti-tumour effect was achieved when mice were treated by RBC@BPQDs-DOX/KIR nanocomposites (p< .01). The tumour eradicating efficiency of RBC@BPQDs-DOX/KIR nanocomposites was also better than either RBC@BPQDs-DOX or RBC@BPQDs-KIR nanocomposites alone (Figure S4). As shown by H&E staining (), the majority of tumour cells are necrosis in the tumour tissues of RBC@BPQDs-DOX/KIR group. During the entire course of treatment, there were no dramatic changes in body weight in all the mice groups (). The alterations of blood cell count exhibited a decreasing trend in all treatment groups, while changes of AST level represented an increasing trend (Table S1), which was probably due to the bone marrow suppression effects and cardiotoxicity of DOX [Citation41,Citation42]. However, the mice treated with RBC@BPQDs-DOX/KIR nanocomposites exhibited a lower cardiotoxicity through analysing the histological micrographs and biochemical parameters (Figure S5 and Table S1), probably because of its EPR effect, which cause DOX primarily present in the tumour section. No abnormalities were found in the histologic images of other organs (Figure S6). The in vivo experiment indicated an outstanding anti-tumour effect and biocompatibility of RBC@BPQDs-DOX/KIR nanocomposites.
Figure 8. In vivo anti-tumour effects of RBC@BPQDs-DOX/KIR. (A) Tumour growth patterns after various treatments for 14 days. Tumour volumes were normalized to baseline values. (B) The body weight changes of Hela xenograft model during treatments were normalized to baseline values. (C) Representative images of tumours after intravenous injection of different formulations at day 14. (D) The histological observation of the tumour tissues after the treatment with different formulations stained with haematoxylin and eosin (H&E). Scale bar: 10 μm. Data are mean ± SD (n = 3). **p<.01.
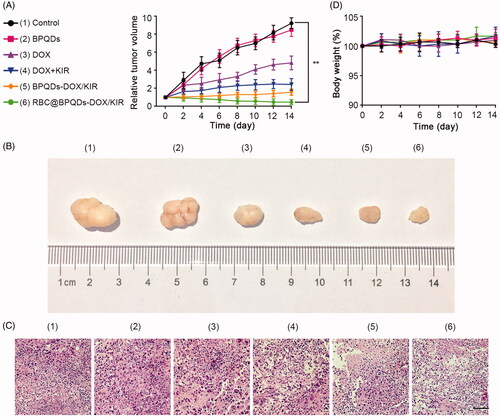
Conclusions
To conclude, RBC@BPQDs-DOX/KIR, the RBCm-encapsulated BPQDs passively targeting the tumour cells, was a combination of chemotherapeutics and anti-inflammatory therapeutics. RBC@BPQDs-DOX/KIR possessed excellent monodispersion capacity, high drug loading rates and fluorescence emission. Encapsulation by the RBCm was important for its evasion of the immune system, longer retention in the tumour, and shorter retention in the normal organs. This novel nanoparticle drug delivery system had tumour passively targetability through EPR effect. Moreover, DOX and KIR acted synergistically in RBC@BPQDs-DOX/KIR, inhibiting the tumour cells from resistance-to-death by reducing the expression of anti-apoptosis protein Bcl-2 and improving the pro-apoptosis protein Bax. These two active components also downregulated the pro-inflammatory cytokines TNF-α and IL-6, remodelled TME, magnified the anti-tumour effect and reduced the toxic and side effects from the treatment of DOX alone. Due to these features, RBC@BPQDs-DOX/KIR was a safe and effective targeted drug delivery system for efficient tumour therapy.
Acknowledgements
The authors thank Yanwei Luo, PhD and Weiwei Luo for providing cell lines, and Xinying Xiang for providing experimental support.
Disclosure statement
The authors have no conflicts of interest to declare.
Additional information
Funding
References
- Shapira A, Livney YD, Broxterman HJ, et al. Nanomedicine for targeted cancer therapy: towards the overcoming of drug resistance. Drug Resist Updat. 2011;14:150–163.
- Hori S, Herrera A, Rossi JJ, et al. Current advances in aptamers for cancer diagnosis and therapy. Cancers. 2018;10:9.
- Bairagi U, Mittal P, Singh J, et al. Preparation, characterization, and in vivo evaluation of nano formulations of ferulic acid in diabetic wound healing. Drug Dev Ind Pharm. 2018;44:1783–1796.
- Hanahan D, Weinberg RA. Hallmarks of cancer: the next generation. Cell. 2011;144:646–674.
- Wang K, Karin M. Tumor-elicited inflammation and colorectal cancer. Adv Cancer Res. 2015;128:173–196.
- Landskron G, De la Fuente M, Thuwajit P, et al. Chronic inflammation and cytokines in the tumor microenvironment. J Immunol Res. 2014;2014:1.
- Qiao Y, He H, Jonsson P, et al. AP-1 is a key regulator of proinflammatory cytokine TNFalpha-mediated triple-negative breast cancer progression. J Biol Chem. 2016;291:5068–5079.
- Coussens LM, Werb Z. Inflammation and cancer. Nature. 2002;420:860–867.
- Wang ZY, Liang CH, Shi F, et al. Cancer vaccines using supramolecular hydrogels of NSAID-modified peptides as adjuvants abolish tumorigenesis. Nanoscale. 2017;9:14058–14064.
- Buondonno I, Gazzano E, Jean SR, et al. Mitochondria-targeted doxorubicin: a new therapeutic strategy against doxorubicin-resistant osteosarcoma. Mol Cancer Ther. 2016;15:2640–2652.
- Chen CJ, Kono H, Golenbock D, et al. Identification of a key pathway required for the sterile inflammatory response triggered by dying cells. Nat Med. 2007;13:851–856.
- Zhang ZY, Lin GH, Yan YJ, et al. Transmembrane TNF-alpha promotes chemoresistance in breast cancer cells. Oncogene. 2018;37:3456–3470.
- Kim J, Kim MB, Yun JG, et al. Protective effects of standardized Siegesbeckia glabrescens extract and its active compound kirenol against UVB-induced photoaging through inhibition of MAPK/NF-kappaB pathways. J Microbiol Biotechnol. 2017;27:242–250.
- Yin JT, Wang PQ, Yin YY, et al. Optimization on biodistribution and antitumor activity of tripterine using polymeric nanoparticles through RES saturation. Drug Deliv. 2017;24:1891–1897.
- Hu Y, Ke L, Chen H, et al. Natural material-decorated mesoporous silica nanoparticle container for multifunctional membrane-controlled targeted drug delivery. IJN. 2017;12:8411–8426.
- Shao JX, Abdelghani M, Shen GZ, et al. Erythrocyte membrane modified janus polymeric motors for thrombus therapy. ACS Nano. 2018;12:4877–4885.
- Parodi A, Quattrocchi N, van de Ven AL, et al. Synthetic nanoparticles functionalized with biomimetic leukocyte membranes possess cell-like functions. Nat Nanotechnol. 2013;8:61–68.
- Fang RH, Hu CMJ, Luk BT, et al. Cancer cell membrane-coated nanoparticles for anticancer vaccination and drug delivery. Nano Lett. 2014;14:2181–2188.
- Gao WW, Fang RH, Thamphiwatana S, et al. Modulating antibacterial immunity via bacterial membrane-coated nanoparticles. Nano Lett. 2015;15:1403–1409.
- Li RX, He YW, Zhang SY, et al. Cell membrane-based nanoparticles: a new biomimetic platform for tumor diagnosis and treatment. Acta Pharm Sin B. 2018;8:14–22.
- Oldenborg PA, Zheleznyak A, Fang YF, et al. Role of CD47 as a marker of self on red blood cells. Science. 2000;288:2051–2054.
- Maeda H. The enhanced permeability and retention (EPR) effect in tumor vasculature: the key role of tumor-selective macromolecular drug targeting. Adv Enzyme Regul. 2001;41:189–207.
- Li M, Liu Y, Chen J, et al. Platelet bio-nanobubbles as microvascular recanalization nanoformulation for acute ischemic stroke lesion theranostics. Theranostics. 2018;8:4870–4883.
- Tao W, Zhu X, Yu X, et al. Black phosphorus nanosheets as a robust delivery platform for cancer theranostics. Adv Mater. 2017;29;1603276.
- Chen WS, Ouyang J, Liu H, et al. Black phosphorus nanosheet-based drug delivery system for synergistic photodynamic/photothermal/chemotherapy of cancer. Adv Mater. 2017;29;1603864.
- Li Y, Liu Z, Hou Y, et al. Multifunctional nanoplatform based on black phosphorus quantum dots for bioimaging and photodynamic/photothermal synergistic cancer therapy. ACS Appl Mater Interfaces. 2017;9:25098–25106.
- Yang Y, He L, Liu Y, et al. Promising nanocarriers for PEDF gene targeting delivery to cervical cancer cells mediated by the over-expressing FRalpha. Sci Rep. 2016;6:32427.
- Jiang JG, Chen CL, Card JW, et al. Cytochrome P450 2J2 promotes the neoplastic phenotype of carcinoma cells and is up-regulated in human tumors. Cancer Res. 2005;65:4707–4715.
- Luk BT, Fang RH, Hu CMJ, et al. Safe and immunocompatible nanocarriers cloaked in RBC membranes for drug delivery to treat solid tumors. Theranostics. 2016;6:1004–1011.
- Kumari N, Dwarakanath BS, Das A, et al. Role of interleukin-6 in cancer progression and therapeutic resistance. Tumor Biol. 2016;37:11553–11572.
- Garcia-Aranda M, Perez-Ruiz E, Redondo M. Bcl-2 inhibition to overcome resistance to chemo- and immunotherapy. Int J Mol Sci. 2018;19:3950.
- Liu ZQ, Ding Y, Ye N, et al. Direct activation of Bax protein for cancer therapy. Med Res Rev. 2016;36:313–341.
- Zheng JH, Follis AV, Kriwacki RW, et al. Discoveries and controversies in BCL-2 protein-mediated apoptosis. FEBS J. 2016;283:2690–2700.
- Ben-Neriah Y, Karin M. Inflammation meets cancer, with NF-kappaB as the matchmaker. Nat Immunol. 2011;12:715–723.
- Chen MF, Chen PT, Lu MS, et al. IL-6 expression predicts treatment response and outcome in squamous cell carcinoma of the esophagus. Mol Cancer. 2013;12:26.
- Dash SK, Chattopadhyay S, Ghosh T, et al. Self-assembled betulinic acid protects doxorubicin induced apoptosis followed by reduction of ROS-TNF-alpha-caspase-3 activity. Biomed Pharmacother. 2015;72:144–157.
- Zhang F, Duan S, Tsai Y, et al. Cisplatin treatment increases stemness through upregulation of hypoxia-inducible factors by interleukin-6 in non-small cell lung cancer. Cancer Sci. 2016;107:746–754.
- Ishimoto T, Miyake K, Nandi T, et al. Activation of transforming growth factor beta 1 signaling in gastric cancer-associated fibroblasts increases their motility, via expression of rhomboid 5 homolog 2, and ability to induce invasiveness of gastric cancer cells. Gastroenterology. 2017;153:191–204.
- Keeney JT, Miriyala S, Noel T, et al. Superoxide induces protein oxidation in plasma and TNF-alpha elevation in macrophage culture: insights into mechanisms of neurotoxicity following doxorubicin chemotherapy. Cancer Lett. 2015;367:157–161.
- Wei LH, Kuo ML, Chen CA, et al. Interleukin-6 promotes cervical tumor growth by VEGF-dependent angiogenesis via a STAT3 pathway. Oncogene. 2003;22:1517–1527.
- Szwed M, Jozwiak Z. Genotoxic effect of doxorubicin-transferrin conjugate on human leukemia cells. Mutat Res Genet Toxicol Environ Mutagen. 2014;1;771:53–63.
- Abushouk AI, Ismail A, Salem AMA, et al. Cardioprotective mechanisms of phytochemicals against doxorubicin-induced cardiotoxicity. Biomed Pharmacother. 2017;90:935–946.