Abstract
Polymeric micelles (PMs) play a vital role in multidrug co-delivery and cancer treatment. However, development of intelligent PMs further allows PMs to accurately target tumour, selectively release cargo multidrug and increase uptake. Therefore, targets, controlled release and uptake of intelligent PMs should be paid more attention to improvement of synergistic therapeutic outcomes and minimize side effects. In this review, tumour targeting of co-delivery intelligent PMs and its intracellular trafficking mechanisms were overviewed. And this review provides a comprehensive summarization of several intelligent co-delivery PMs. Such a system could control the multidrug to be released simultaneously or sequentially by special properties of tumour microenvironment (TME) (including acidic PH, redox, overexpressed enzyme, excessive temperature) and external environment trigger. Additionally, limitations, clinical translation and future perspectives of intelligent co-delivery PMs were also being discussed in this article.
Introduction
Currently, cancer still posed a serious threat to human health. Among plentiful therapies, chemotherapy remains the most common means of cancer treatment in the clinic [Citation1]. However, the outcome of chemotherapy was often unsatisfactory. On the one hand, poor solubility, serious side effect and multi-drug resistance (MDR) had been identified the “Achilles’ heel” of chemotherapy drugs [Citation2,Citation3]. On the other hand, the pathogenesis of cancer was complex leading to single anti-tumour drug was not sufficient to completely inhibit the tumour, which may induce rapid recurrence of cancer and MDR [Citation4,Citation5].
However, chemotherapy was improved to some extent thanks to the application of nanoscale drugs delivery systems (NDDSs) especially polymeric micelles (PMs). Generally, PMs refer to nanoscale aggregates formed by self-assembly of amphiphilic polymers in water [Citation6]. And, it not only increases the solubility of hydrophobic drugs, avoid drugs being quickly degraded and improve the bioavailability, but also had greater advantage in biological and pharmacokinetics such as good biocompatibility, higher uptake and strong tissue permeability [Citation7,Citation8]. Moreover, combination therapy of multidrug was recognized as a specifically beneficial strategy to boost the anti-tumour effect and reduce adverse reactions. Yet, synergistic combined therapy benefits of drugs cocktail were limited due to significant pharmacokinetics or biodistribution distinction [Citation9,Citation10]. Thus, various co-delivery PMs were designed for co-delivery of multiple drugs to improve the synergistic therapeutic effect in the past few years [Citation11,Citation12]. Unfortunately, cargo multidrug release behaviour of traditional PMs was non-selective and incomplete resulted in lower the synergistic treatment outcome of the cargo drugs. And, non-specific tumour-targeting of PMs led to its bio-distribution at the tumour site to be insufficiently concentrated. Consequently, many kinds of novel intelligent PMs were developed in order to improve the co-delivery performance and synergistic treatment effect of the cargo multidrug (). Usually, intelligence of PMs was mainly reflected in two aspects. First, cargo drugs could be concentrated at the tumour site via active and passive targeting of intelligent PMs, while also significantly increasing cancer cells uptake of drugs [Citation13,Citation14]. Furthermore, intelligence PMs could control drugs release sequentially or simultaneously in the tumour sites by the unique characteristics of the tumour microenvironment (TME) trigger (e.g. acidic pH, excessive temperature, altered redox potential, specificity enzymes) [Citation15,Citation16]. And, external environment stimulation such as light will also be applied to allow intelligent PMs to control release of cargo drugs [Citation17,Citation18]. Certainly, the intelligent release of the multidrug effectively prevented cargo multidrug were released prematurely in blood circulation and non-tumour tissue and enhances the synergistic therapeutic effect of the cargo multidrug.
Table 1. Examples of intelligent polymeric micelles for multidrug co-delivery and cancer therapy.
Therefore, the tumour-target and controlled release of intelligent PMs in the field of multi-drug co-delivery was discussed in this review to explore a feasible strategy for their improvement. Moreover, intracellular trafficking of PMs, unique properties of the TME was summarized and introduced, which would provide a useful reference for the design of new intelligent PMs. The limitations and clinical translation of intelligent PMs as well as their perspectives also were discussed in this review.
Polymer micelles
PMs are definition via the capacity of the co-polymer to self-assemble into nanoscale aggregates that have a hydrophilic shell and hydrophobic core. The hydrophobic core of PMs provided a home for water-insoluble drugs and the hydrophilic shell could be well isolated the external environment and avoid cargo drugs being quickly degraded [Citation19,Citation20]. However, ideal PMs not only co-loaded multidrug but also possessed excellent physical properties such as suitable size distribution, morphology and blood stability. Otherwise, the therapeutic effect of the PMs was closely related to its intracellular transformation including endocytosis of PMs and endosomal/lysosomes escape [Citation21,Citation22]. Therefore, in this chapter, the physical properties of PMs and intracellular trafficking were discussed, and it was hoped to provide ideas for the design of novel intelligent PMs.
Physical characteristic of polymer micelles
The co-polymer is composed by covalently attaching hydrophilic and hydrophobic biomaterials including hydrophilic polyethylene glycol (PEG) and hydrophobic polycaprolactone [Citation23,Citation24]. Notably, RNAs had been exploited as biomaterials to construct a novel RNA-based micelle in recent years [Citation25]. For example, a micelle based on phi29 pRNA 3-way junction was designed via Shu et al. [Citation26]. Such platform not only gave excellent versatility, strong cancer cells binding and higher uptake, but also co-loaded different types of chemo-drugs for improved combination treatment. Hence, RNA-based micelles co-delivery carriers possessed huge potential for clinical applications.
RNA was applied successfully to significantly improve the performance of micelles, but delivery behaviour of cargo multidrug in vivo was influenced by the physical property of PMs. For example, the blood circulation of PMs could be extended by controlling its particle size distribution. Due to adequate size distribution (50–200 nm) could be to avoid the quick clearance of the reticuloendothelial system (RES). It also enhances the accumulation of cargo multidrug in tumour tissues via the enhanced permeability and retention (EPR) effect [Citation27,Citation28]. Duong et al. [Citation29] noticed that micelles with a particle size of less than 200 nm had lower clearance and higher cellular uptake. Moreover, the morphology of the PMs was undoubtedly another important physical property. Usually, PMs existed in multiple morphology including spherical, vesicular and cylindrical, in balances with each other [Citation30]. Among these morphologies, the spherical was the most common and was thought to improve cellular uptake than other morphology [Citation31,Citation32]. Apart from, in order to make sure PMs stabile in blood circulation, it was necessary to determine the critical micelle concentration (CMC) of the PMs. The stability of the micelle was maintained when the concentration of the copolymer in the solution was near or above the CMC. Conversely, the micelles were dissociated into a copolymer when the concentration of the copolymer in the solution was lower than the CMC [Citation33,Citation34]. Therefore, the effective reduction of the CMC of the micelles was advantageous for improving its stability in vivo.
Intracellular trafficking of polymer micelles
Usually, treatment results of some PMs were suboptimal, part of the reason was that PMs failed to enter the cancer cells by endocytic pathways and escape lysosomes/endosomes, which ultimately led to cargo multidrug release blocked [Citation22,Citation35]. Therefore, the endocytic pathways and lysosomes/endosomes escape of PMs had been elucidated, which contribute to the optimization of the therapeutic effect of PMs and the design of novel PMs.
The PMs was rapidly internalized into cancer cells via a variety of endocytosis pathways include clathrin-dependent receptor-mediated endocytosis (RME), pinocytosis and phagocytosis [Citation36,Citation37]. Among these, RME was deemed to be the major and most effective pathway for the uptake of PMs that surface modified via ligands. And RME processes mainly involved PMs modified by the ligand binds to a specific receptor on tumour cells membrane, and then, the PMs enter the cell via membrane invagination [Citation38]. Moreover, pinocytosis and phagocytosis also be involved in the endocytosis pathways of PMs. These endocytic pathways required the tumour cell membrane to formed the pseudopods and ruffles to encapsulate PMs [Citation39]. Notably, the process of PMs being endocytosed usually related multiple pathways. Therefore, various endocytosis pathways should be considered simultaneously when the endocytic mechanism of the novel PMs was studied.
It was captured and destroyed by lysosomes/connotations after intelligent PMs being transported into the cells by endocytic pathways, finally led to loss of therapeutic effect [Citation22]. Therefore, the lysosome/endosomal escape of PMs was the key to their operative treatment. At present, a variety of techniques such as the proton sponge effect, photochemical internalization and lysosome/endosomal membrane-selective destabilization could mediate the escape of PMs [Citation40–42]. For instance, PMs with excess tertiary amines induced chloride ions and water influx into the lysosome/endosome, and eventually resulted in cracked lysosome/endosome. This technique was referred to as the proton sponge effect [Citation43]. Unfortunately, such a system could produce cytotoxicity owing to tertiary amine caused a strong positive charge. Similarly, photochemical internalization mediated the escape also caused cytotoxicity due to photosensitizers were stimulated by irradiation produced reactive singlet oxygen for the destruction of the endosomal/lysosomal membrane [Citation44]. Moreover, it was another important technique to mediate evasion of PMs via lysosome/endosomal membrane-selective destabilization. Usually, the hydrophilicity of such PMs increased in lysosome/endosomal, then inserted into the membrane of lysosome/endosomal and destroyed its stability to mediate the escape of micelles [Citation42]. In general, internalization and escape processes of micelles were often multi-channel, so their research should also be comprehensive. In addition, deep insights into the knowledge of the internalization and escape of micelles were a key point to improve the therapeutic effect of micelles and promote clinical translation.
Passive and active targeting are applied for intelligent co-delivery of PMs
The PMs had been proved to effectively improve the solubility of drugs, but it still remains challenges such as non-specific tumour targeting, which resulted in the biodistribution of cargo multidrug in the tumour tissue were not concentrated. Fortunately, Maeda et al. [Citation45] designed the original polymer anti-tumour drug and successfully improved tumour accumulation of drugs via EPR effect-mediated passive targeting, which also opened the curtain of passive targeting of micelles in 1979. Moreover, strategies for actively targeting tumours by modified homing molecules such as ligands or antibody on PMs surfaces had also been widely reported due to active targeting was more accurate than passive targeting [Citation46] ().
Figure 1. (a) Passive targeting of PMs. PMs selectively were trapped in the tumour tissue through the incomplete vasculature around tumours and appropriate particle size. On the contrary, the free drug diffuses into the tumour tissue by free diffusion and then spreads from the tumour tissue to the other tissue. (b) Active targeting of PMs. PMs selectively bind to various receptors overexpressing the surface of a cancer cell by modified the corresponding ligand on its surface.
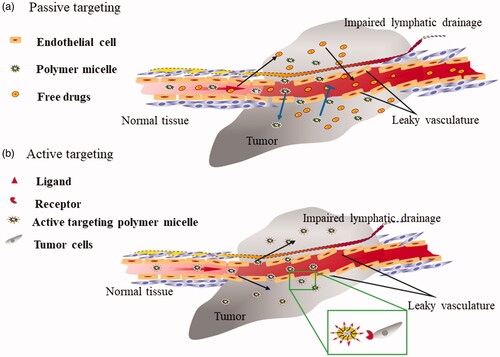
Passive targeting co-delivery PMs based on the EPR effect
Solid tumours hold specific characteristics compared with normal tissue including the incomplete structure of vasculature and lymphatic drainage obstruction, hence resulted in enhanced permeability and retention of macromolecular and nanoparticles [Citation47,Citation48]. This phenomenon was called EPR effect of solid tumour. Therefore, intelligent PMs based on the EPR effect had been extensively studied for passively target tumours. And the EPR effect was inseparable from the particle size distribution of PMs usually the particle size range of 50–200 nm was suitable [Citation49,Citation50]. For example, a multidrug co-delivery mixed PMs was designed by Yin M etc [Citation51]. And experimental manifested that PMs with particle size in the range of 93.6–161.6 nm could be significantly concentrated in S180 tumour-bearing mice due to the EPR effect. Therefore, particle size of the PMs was controlled within a certain range, which will be very beneficial to increase the accumulation of cargo multidrug in the tumour tissue by the EPR effect.
Unfortunately, it was insufficient for EPR effect-mediated passive targeting outcomes. The EPR effect was affected by various factors such as the size distribution of the PMs, the type and location of the tumour. Moreover, the complex relationship between the three cases the exact correlation between them was not clearly understood. Until today, EPR effect had grown up to become a controversial topic in the passive targeting tumour area.
Cancer-specific homing devices mediated active targeting of co-delivery PMs
EPR effect-mediated passive targeting frequently failed to accurately target tumours due to a variety of factors. However, more precise target of PMs was necessary to further improve the accumulation of drugs at the tumour site. Accordingly, various cancer-specific homing molecules modified PMs were designed for accurately co-delivery of multidrug [Citation52,Citation53]. Usually, these molecules were capable of specifically recognizing and target certain tumour-specific antigens and the receptor that are overexpressed by cancer cells but rarely expressed or under-expressed on normal cells [Citation54,Citation55].
Ligands mediated active targeting strategies
Numerous ligands had been successfully utilized in PMs to mediate the active targeting of PMs owing to high specificity and strong binding affinity such as folate acid [Citation56], hyaluronic acid [Citation57], transferrin [Citation58], aptamer [Citation59], low-density lipoprotein [Citation60]. Therefore, some classical ligands had been reviewed in this chapter to provide ideas for the design of novel active targeting PMs.
Folate receptors (FRs) are often overexpressed on a variety of cancer cells surfaces for maximum uptake of folic acid (FA), while the expression of FRs in normal tissues was very low. And FA toward FRs possessed very high affinity [Citation61,Citation62]. Therefore, FA was often modified as a ligand on the surface of co-delivery PMs for accurate targeting tumour. Tao et al. [Citation63] reported a novel FA covalently PMs for active targeting co-delivery of docetaxel (DOC) and small interfering RNA of matrix metalloproteinase-9. This system obviously improved the accumulation of cargo multidrug in tumour sits via the active targeting of FA. Moreover, growth of tumour and proliferating cell nuclear antigen (PCNA) expression was suppressed effectively when treated with drug-loaded PMs. Undoubtedly, their work fully proof FA was an effective active targeting ligand, but the optimal amount of FA on the PMs still lacks specific research.
CD44 protein plays a critical role in tumour proliferation, invasion and metastasis; thus, it is expressed at high levels in many tumours [Citation64,Citation65]. Especially, it was reported that hyaluronic acid (HA) could efficiently bind to CD44 to become a complex and was then endocytoses by tumour cells [Citation57,Citation66]. HA was conjugated to the surface of co-delivery PMs as a target by researchers based on this principle. For instance, Ma et al. [Citation67] produced HA-vitamin E succinate (HA-VES) graft co-polymer for targeting co-delivery of doxorubicin (DOX) and curcumin (CUR). Their research indicates accumulation concentration and uptake of HA-VES/DOX/CUR could be significantly increased by HA-mediated active targeting and CD44-mediated RME. Also, it had higher tumour inhibition rate and lower side effects compared with non-targeted groups. This indicates that CD44 protein targeting by HA-mediated was a promising strategy for intelligent co-delivery of multidrug and enhances uptake rate.
Additionally, transferrin (Tf) was also a classic ligand. The expression of TfR on the cancer cells can be dramatically up-regulated in order to provide enough iron during rapid proliferation [Citation58,Citation68]. Thus, Tf was widely regarded as an ideal homing device for PMs. For instance, Zou et al. [Citation69] designed a TfR-targeted PMs to co-delivery paclitaxel (PTX) and tariquidar (TRQ), which was consisted of three block polymers, PEG, ethanolamine (PE) and Tf. Such system successfully increased accumulation of PMs in tumour tissue by Tf-mediated active targeting, and enhance its uptake rate by TfR-mediated endocytosis. However, compared to other small molecule ligands, the stability of Tf could be decreased in complicated biological environments in vivo. And, its industrial production costs were higher. Therefore, these effectively solved issues were conducive to promoting the clinical translation of Tf-modified PMs.
Active targeting endowed by antibody fragments
Monoclonal (mAbs) were considered to be another homing device for PMs due to its accurately target tumour-specific antigens and high binding affinity [Citation70]. However, antibody derivatives such as Fab fragments, nanobody, single-chain antibody fragments (scFv), etc., had higher application value than whole antibodies [Citation71] because they possessed small size, higher specific target, higher loading capacities and better tissue penetration compared with whole antibodies [Citation72,Citation73]. For instance, a glucose transporter-1 (GLUT1) antibody scFv-modified 1,2-distearoyl-sn-glycerol-3-phos phoethanolamine-N-PEG PMs was reported by Sarisozen et al. [Citation74] to co-delivery doxorubicin and curcumin. The cellular association of PMs and nuclear accumulation of DOX significantly increased in vitro studies owing to GLUT1 scFv modification. Moreover, GLUT1 scFv modification PMs demonstrated deeper penetration in the 3D spheroid model. However, the reported multidrug co-delivery PMs with antibody modifications were rare. This may cause by the low stability of the antibody which resulting in the loss of activity during binding to the PMs, and find an antibody or derivatives with the ideal structure and function was difficult.
Superior targeting properties were shown via these ligands and antibody, yet, in comparison to proteins or antibody the use of aptamer and small molecule ligand such as FA and HA might have advantages including higher stability in the complex biological environment and industrial production costs were relatively low. Hence, optimization of targeting performance of ligands and antibody as well as the development of novel homing molecules continue to be the focus of future research.
Tumour microenvironment-responsive co-delivery intelligent PMs
Targeting co-delivery of PMs significantly increased the accumulation of drugs in tumour tissues, but their drug release behaviour usually expressed as uncontrollable due to degradation of the skeleton was non-selective. [Citation75,Citation76]. Fortunately, the emergence of the tumour microenvironment (TME) sensitive PMs provided a breakthrough in the solution of the drug release problem of PMs.
Lots of research results demonstrated that tumour induces the formation of malformed blood vessels resulting in a severe hypoxia state of the TME, follow by cancer cells utilize anaerobic glycolysis as the main mode of energy supply. However, the accumulation of lactic acid by anaerobic glycolysis creates an acidic pH in the TME [Citation77]. Apart from acid pH, TME had a high concentration of glutathione (GSH), high temperature and many particular enzymes compared with the normal tissues [Citation78,Citation79]. Thereupon, characteristic of the TME was successfully utilized as the “keys”, and intelligent co-delivery PMs was designed as matching “locked storage box”. In other words, the intelligent PMs, when delivered to the tumor tissue, were destroyed via characteristic of the TME ultimately achieve the co-loaded drugs were controllably released at the tumour site. Thereby the strategies for the development of TME-responsive co-delivery intelligent PMs used in anticancer study were reviewed individually below.
Acid tumour microenvironment-responsive co-delivery intelligent PMs
The TME pH is reduced to 6.5 as a result of the accumulation of lactic acid. Further, endosomes and lysosomes in tumour cells possessed a lower PH value (5.0–6.0 and 4.0–5.0) [Citation80,Citation81]. Accordingly, the strategy that drugs were selectively released to the tumour site from the PMs based on the acidic TME trigger had become a research hotspot in recent years. Normally, PMs had several strategies for utilizing the difference in pH values.
The acid-labile linkage was introduced into the PMs structure to mediate the intelligent release of the micelles was an important strategy. Typically, acid-labile linkage was disassembled when exposed to a low pH environment such as acetal, [Citation82] hydrazine [Citation83] and Cis-aconityl bond [Citation84,Citation85]. Additionally, co-loaded drugs could be released sequentially from the PMs based on the difference in acid-labile linkage sensitivity at the same pH level. For example, Xu et al. [Citation86] prepared a dual pH-responsive cross-linked PMs N-(2-hydroxypropyl) methacrylamide (HPMA)-β-sitosterol-DOX for co-delivery of DOX and antiangiogenesis drug axitinib (AXI). DOX was conjugated to HPMA polymers by hydrazone linkage and AXI was physically encapsulated. Furthermore, in order to enhance PMs stabile in blood circulatory, drug-loaded PMs was cross-link with benzoic-imine. Interestingly, hydrazone linkage was very hard to cleavage than benzoic-imine bonds at pH 6.5, but when the pH level drops to 5.0, hydrazone linkage also was rapidly destroyed, thus allow AXI to be released extracellular (pH 6.5) and DOX was released into endolysosomal (pH 5.0). And this system effectively inhibits angiogenesis and tumour growth and significantly increases the synergistic anti-tumour. Therefore, the emergence of dual pH-sensitive intelligent co-delivery PMs paved the strategy for the co-delivery and release of antitumor drugs of different mechanisms in the extracellular and intracellular in the future. Overall, acid-labile bond as a link between the drugs and polymer or polymer to polymer, which prevents the premature release of the drug in the blood circulation and achieves drugs was released intelligently in the tumour tissue to effectively minimizing the adverse effects and increasing synergistic anti-tumour outcomes.
The protonation of ionized groups in polymers at acidic pH was commonly regarded as another effective pH-sensitive strategy [Citation87]. The electrostatic interaction between the co-polymer was changed and the hydrophobicity or hydrophilic properties of the polymer block was reversed eventually leading to the disintegration of the PMs when the ionizable groups were protonated in the acidic TME [Citation88–90]. For example, Yu et al. [Citation91] constructed a novel triple-layered micelle based on PEG, poly(aminolated glycidyl methacry-late)(PAGA) and poly(2-(diisopropyl amino)ethyl methacrylate)(PDPA) for co-delivery siRNA-p65 and cisplatin prodrug, in which the PDPA could undergo protonated at acidic PH. The protonated PDPA segment induced the transition of hydrophobic core from hydrophobic to hydrophilic eventually resulting in PMs dissociation and the siRNA-p65/cisplatin prodrug was released quickly, as indicated in . Furthermore, a pH-sensitive PMs based on an amphiprotic dendrimer was prepared by Han et al. [Citation92] to co-delivery 5-fluorouracil and DOX, which consisted of two block polymers, hydrophobic polylactide and hydrophilic pH-responsive polyami-doamine dendritic. The tertiary amine in the polyamine–polyamine dendritic structure was protonated at acidic buffer (pH 5.0) such that the entire PMs carries a positive charge. The appearance of a positive charge in the PMs causes extremely electrostatic repulsion between the polymer molecules which ultimately induce disintegration of the PMs. To sum up, the key to this strategy was the conversion of the properties of the co-polymer after protonation of the group at acidic PH.
Figure 2. Schematic illustrations for intracellular activation and delivery of cisplatin prodrug and siRNA co-loaded pH-responsive micelles. The micelles were degraded inside acidic late endosome/lysosome due to protonation of PDPA segment induction the transition of hydrophobic core from hydrophobic to hydrophilic. Simultaneously, endosome/lysosome rupture releases the Pt(IV)-OC prodrug and siRNA through the “proton sponge effect" and strong haemolytic activity of the micelles.
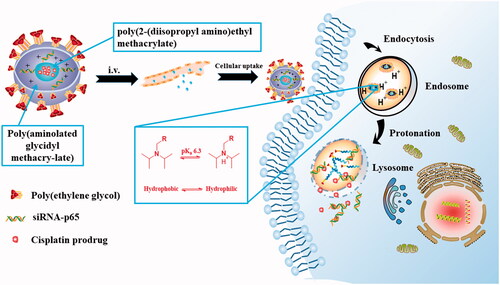
Interestingly, acidic TME was not only used to trigger the release of drugs but also to increasing internalization of co-loaded multidrug [Citation93]. As we know, the cell membrane was negatively charged, so that the positively charged co-delivery PMs could be internalized quickly through electrostatic interaction [Citation94]. However, positively charged co-delivery PMs was a challenge with short half-life towing to it was easily captured through the RES [Citation95,Citation96]. Wherefore, by constructing a charge-transforming micelle, usually negatively charged at physiological pH level, could convert its surface charge into a positive charge in an acidic TME, to avoid the clearance of the RES, increased the uptake of PMs. For instance, a 2,3-dimethylmaleic anhydride (DMA)-modified poly (ethylene glycol)-block-poly(l-lysine) (PEG-b-PLL) block PMs was prepared by Huo et al. [Citation16]. The prepared PMs could maintain negative surface charge in plasma (pH 7.4), but a large number of amino groups were exposed during the hydrolysis of DMA groups in tumour tissue (pH 6.5), and then the exposed amino group was rapidly protonated to become positive leading to increase of cellular internalization. Therefore, charge conversion, as acidic TME response strategy, provides a reliable way to solve the problem of long circulation and high internalization rate cannot have both. Notably, the presence of large amount of amino groups yielding strong positive charges could cause severe cytotoxicity.
Redox-responsive co-delivery intelligent PMs
Reduction potential had been identified a viable biomarker for distinguishing tumours from normal tissues because there was a significant discrepancy in the concentration of glutathione (GSH) between the TME and the normal tissue microenvironment. It has been reported that the concentration of GSH in the TME was four times that of normal tissues microenvironment, particularly important is the concentration of GSH intracellular (2–10 mM) in the tumour was 10–1000-fold the extracellular (2–10 μM) [Citation97–99]. Hence, redox-responsive strategy was applied to the co-delivery PMs field for intelligent release of co-loaded drugs [Citation76,Citation100]. Usually, disulphide bonds were introduced into the PMs due to disulphide bonds could be reduced to sulphhydryl groups in the presence of a certain concentration of GSH, dithiothreitol (DTT) or other reducing agents [Citation101], eventually leading to PM decomposition.
According to this theory, Hu et al. [Citation102] co-loaded DOX and the necrosis factor-related apoptosis-inducing ligand (pTRAIL) in GSH-sensitive PMs, in which disulphide bonds as response units. Such a system was degraded by the reduction of disulphide bonds that eventually caused the release of DOX and pTRAIL when PMs were delivered to the TME. Antitumour study demonstrated that it possessed significantly tumour inhibition rate (94.0%) more than other control groups. Together, GSH-sensitive was analogous to pH-sensitive which could be intelligently released to multidrug. However, compared with the acid-responsive, the GSH-responsive mainly releases co-loaded drugs to cytosol and cell nucleus. Additionally, GSH-responsive pathway was single, mainly through the break of disulphide bond cleavage, which means that the study of multipath GSH-response in the field of multidrug co-delivered PMs had broad prospects.
Enzyme response co-delivery intelligent PMs
Intelligent co-delivery PMs of enzyme response also had great clinical application prospects. Owing to many diseases such as cancer, the expression of the enzyme undergoes changes such as dysregulation and activity imbalance [Citation103]. Especially, the enzyme possesses specificity allowing for some specific and complex biologically inspired chemical reactivity. The catalytic efficiency of the enzyme was gentle and efficient [Citation104,Citation105]. Hence, enzymes had been extensively used as triggers for controlled drugs release of co-delivery PMs or improved internalization of PMs, in which the classical enzyme was matrix metalloproteinases (MMPs).
MMPs were a kind of the zinc-dependent endopeptidase family. According to reports, MMPs was over expressed on the surface of almost all tumour due to its closely involved in tumour invasion and metastasis [Citation106,Citation107]. Therefore, in the few studies, MMPs (mainly MMP2 and MMP9) as biological triggers be employed in co-delivery PMs for controlling the release of multidrug and increased internalization of PMs. For example, Zhu et al. [Citation108] produce a siRNA/paclitaxel (PTX) co-loaded PMs of MMP2-sensitive PEG-peptide-polyethylenimine-1,2-dioleoyl-snglycero-3-phosphoethanolamine (PEG-pp-PEI-PE) to improve internalization of PMs. Similar to low PH-mediated inversion charge, the cationic PEI was immediately exposed to improve the internalization of PMs based on MMP2 triggered PEG cleavage. And the IC50 of PTX was reduced to approximately 15 nM due to synergistic therapeutic between co-loaded drugs. Additionally, MMP2-sensitive mixed micelles (MM) was reported by Salzano et al. [Citation109], which was prepared by mixed self-assembly of the three block copolymers including MMP2-responsive Dox prodrug (PEG2k-peptide-Dox), miRNA covalently linked polymer (miRNA-34a-S-S-phospholipid) and cell-penetrating peptide (TATp) modifier polymer (TAT-PEG1k-phospholipid). The DOX was released via the triggers of MMP2 could be a successful reduction in the premature release of DOX. And MM had significant cytotoxicity against HT1080 cells (over expressing MMP2) than MCF-7 cells (low expression of MMP2) in vivo and in vitro. Collectively, MMPs were exploited as biological triggers control release of multidrug and facilitate micelle’s internalization by exposure of cationic after MMP2-mediated covalent bond cleavage. In addition, the variety of tumour-specific enzymes was favourable to the development of novel enzyme-responsive intelligent PMs.
Temperature-responsive co-delivery intelligent PMs
Temperature plays vital roles in TME signage. Due to tumour cells was required to provide sufficient energy for proliferation by rapid aerobic glycolysis, resulted in local temperature of tumour tissue was elevated (40–42 °C) [Citation110]. Additionally, the temperature could be raised by external force (using an external heat source/device). Wherefore, some temperature-responsive intelligent PMs were designed to co-loaded multidrug by physically encapsulated and covalently linking. And co-loaded multidrug were released by the physicochemical properties of thermo-responsive blocks of PMs were dramatically changed at the TME [Citation111,Citation112].
In recent years, Pluronics (poly (ethylene glycol)-block-Poly (propylene glycol)-block-poly (ethylene glycol) (PEO-PPO-PEO)) as thermo-responsive blocks in the co-delivery intelligent PMs was widely reported. For instance, a thermo-responsive shell cross-linked micelles was prepared by Lee et al. [Citation113] to achieve drug and gene co-delivery, which consisted of double-block polymers, Pluronic F127 (PF127) and polyethylenimine (PEI). The hydrophobic interaction between the rigid PPO chains in the micelle core was enhanced with increased temperature and the resulted squeezing effect makes the micelles more compact; thus, the drug was expelled to cancer sites from PMs. Undoubtedly, their research provided a favourable basis for the development of temperature-sensitive PMs. Additionally, the temperature also could be elevated by an external force (using an external heat source/device). Therefore, temperature-sensitive PMs were considered to have considerable potential as a novel intelligent co-delivery PMs for improving the anti-tumour effect.
External environment-responsive co-delivery intelligent PMs
Compared with TME-responsive intelligent PMs, external environment-responsive intelligent PMs had greater controllability via gave external force such as light [Citation114], magnetic [Citation115] and ultrasound [Citation116]. Until now, unfortunately, the external environmental signals that were deployed in the field of multidrug co-delivery were concentrated in light. For example, Wei et al. [Citation117] designed a new light responsive PMs based on a protoporphyrin IX (PpIX,)-conjugated and acetylated-chondroitin sulphate (AC-CS) for simultaneously encapsulated DOX and apatinib (Apa). In this, intelligent carrier could be cracked quickly and release DOX and Apa due to abundant reactive oxygen species generation via the photoconversion of PpIX when exposed to 635-nm red light. And, it successfully combats tumour MDR through the synergistic effect of chemotherapy and photodynamic therapy in vitro studies. Accordingly, cleavage of PMs by localized illumination could greatly enhance the safety profile of DOX/Apa owing to limit the release and accumulation of multidrug in non-tumour locations.
Dual-responsive co-delivery intelligent PMs
Various intelligent PMs in response to TME signals or external stimuli had been designed for multidrug co-delivery, but as research deepens it is found that dual or multiple responses intelligent PMs have higher controllability, antitumor efficacy and make the best of the special stimuli signals compared to the single sensitive intelligent PMs.
Dual pH/redox-sensitive co-delivery intelligent PMs
Among various dual stimulation co-delivery intelligent PMs, the most classical were pH and redox co-stimulation. Commonly, dual-response mechanism based on the degradation of acid-labile linkage or protonation of ionized groups and disulphide bonds was reduced to sulphhydryl groups by GSH. Recently, a dual PH/redox-responsive hybrid PMs composed of poly(ε-caprolactone) (PCL)-ss-PEG-ss-PCL, PCL-acetal-PEG and 1,2-distearoyl-sn-glycero-3-phosphoethanolamine-N-[folate (PEG)-2000] were obtained via Zhang et al.[Citation118] for co-encapsulated DOX and photosensitizer indocyanine green (ICG) to achieve chemo-photothermal combination therapy. Disulphide bonds and acetal cleavage of the hybrid PMs depend on low pH levels and high GSH concentrations, and comparable to additional test group leading to higher DOX (91.6%) release from PMs owing to the synergistic triggering effect of dual pH/redox-sensitive. More importantly, PMs outstanding antitumor activity and non-reversible ablate tumor tissues are due to a combination of chemo-photothermal. Considering this dual pH/redox-sensitive PMs had higher drug release rate and significant synergistic treatment, there was considerable potential to utilize it as a novel nanotherapeutic.
Dual enzyme/redox-sensitive co-deliver PMs
In a recent study, Salzano et al. [Citation109] produced mixed PMs (MM) with a dual enzyme/redox responsive ability for co-delivery DOX and miRNA-34a. The specialty of MM includes MMP2-responsive Dox prodrug copolymer (PEG2k-peptide-Dox) and GSH-sensitive miRNA-34a (miRNA-34a-S-S-PE) conjugate copolymer prodrug, in which PEG2k-peptide and disulphide bonds were MMP2-responsive and redox-sensitive parts, respectively. In this enzyme/redox-sensitive PMs, DOX and miRNA-34a could be rapidly released via breakage of MMP2-sensitive peptide and disulphide bonds in the presence of MMP and GSH and effectively reduce HT1080 cell viability rate and expression of Bcl2 protein.
Conclusions and perspectives
The superiority of intelligent co-delivery PMs was mainly reflected in their passive/active targeting and cargo multidrug were selectively released. Among them, intelligent targeting properties of PMs significantly improve the biodistribution of multidrug in vivo, and selectively release cargo drugs can enhance the synergistic antitumor effect and reduce the side effect of drugs. Unfortunately, intelligent multidrug co-loaded PMs are still limited to laboratory research and this is the fruit of some factors. For instance, (1) Anti-tumour effect of intelligent PMs has been evaluated almost exclusively limited in solid tumours. Conversely, there is still a lack of research on non-solid tumours such as leukaemia. (2) The stability of intelligent PMs in the circulation is worrying which results from the concentration of block co-polymers after they are applied in vivo may be diluted to value below the CMC, leading to disintegration of the PMs. (3) Most research fails to evaluate interaction mechanisms between intelligent PMs and the body or intelligent PMs and drugs. (4) In addition, the clinical translation of intelligent co-delivery PMs still presents various challenges.
Among them, feasibility and challenges of clinical translation of stimuli-responsive intelligent PMs were considered to be the most concerned issue. For example, unique property of the TME provides a theoretical basis for the controlled release of TME-response intelligent PMs. Furthermore, polymer materials constituting the intelligent PMs have good biocompatibility and low cytotoxicity. Most notably, the effectiveness of controlled release for stimuli-responsive PMs was supported by numerous studies. The existence of these reasons provides favourable support for the feasibility of clinical translation of stimuli-responsive intelligent PMs. However, its clinical translation also has many challenges. Owing to non-tumour tissue could produce characteristics similar to the TME when the patient was attacked by other diseases include oxidative stress, inflammation. This ultimately leads to drugs was released in non-tumour tissue and induced side effects. Also, remote spatiotemporal control and non-invasive of exogenous stimulators (such as light) are the highlights of external environment-responsive PMs ability to achieve clinical translation. Unfortunately, tissue penetration of light is insufficient and long-term illumination of light can cause tissue damage that prevents the clinical translation of micelles.
Even so, it is undeniable that intelligent PMs still is a promising multidrug delivery carrier. Importantly, strategy and preparation technology of intelligent PMs are also accumulating and developing. Thence, we believe that the intelligence of PMs and multidrug co-loaded potential will be gradually optimized in the next decade. Moreover, the safety of intelligent PMs and the possibility of clinical translation will be further enhanced with the advancement of nanomedicine technology and research on TME.
Correction Statement
This article has been republished with minor changes. These changes do not impact the academic content of the article.
Disclosure statement
No potential conflict of interest was reported by the authors.
Additional information
Funding
References
- Bray F, Ferlay J, Soerjomataram I, et al. Global cancer statistics 2018: GLOBOCAN estimates of incidence and mortality worldwide for 36 cancers in 185 countries. CA Cancer J Clin. 2018;68:394–424.
- Holohan C, Van Schaeybroeck S, Longley DB, et al. Cancer drug resistance: an evolving paradigm. Nat Rev Cancer. 2013;13:714–726.
- Zhang P, Li J, Ghazwani M, et al. Effective co-delivery of doxorubicin and dasatinib using a PEG-Fmoc nanocarrier for combination cancer chemotherapy. Biomaterials. 2015;67:104–114.
- da Silva-Diz V, Lorenzo-Sanz L, Bernat-Peguera A, et al. Cancer cell plasticity: Impact on tumor progression and therapy response. Semin Cancer Biol. 2018;53:48–58.
- Dawson MA, Kouzarides T. Cancer epigenetics: from mechanism to therapy. Cell 2012;150:12–27.
- Gong F, Chen D, Teng X, et al. Curcumin-loaded blood-stable polymeric micelles for enhancing therapeutic effect on erythroleukemia. Mol Pharmaceutics. 2017;14:2585–2594.
- Gaspar VM, Baril P, Costa EC, et al. Bioreducible poly(2-ethyl-2-oxazoline)-PLA-PEI-SS triblock copolymer micelles for co-delivery of DNA minicircles and doxorubicin. J Control Release. 2015;213:175–191.
- Lee SH, Lee JY, Kim JS, et al. Amphiphilic siRNA conjugates for co-delivery of nucleic acids and hydrophobic drugs. Bioconjugate Chem. 2017;28:2051–2061.
- Jia J, Zhu F, Ma X, et al. Mechanisms of drug combinations: interaction and network perspectives. Nat Rev Drug Discov. 2009;8:111–128.
- Mayer LD, Janoff AS. Optimizing combination chemotherapy by controlling drug ratios. Mol Interv. 2007;7:216–223.
- Mondal G, Almawash S, Chaudhary AK, et al. EGFR-targeted cationic polymeric mixed micelles for codelivery of gemcitabine and mir-205 for treating advanced pancreatic cancer. Mol Pharmaceutics. 2017;14:3121–3133.
- Zhu C, Xiao J, Tang M, et al. Platinum covalent shell cross-linked micelles designed to deliver doxorubicin for synergistic combination cancer therapy. Int J Nanomedicine. 2017;12:3697–3710.
- Yao C, Liu J, Wu X, et al. Reducible self-assembling cationic polypeptide-based micelles mediate co-delivery of doxorubicin and microRNA-34a for androgen-independent prostate cancer therapy. J Control Release. 2016;232:203–214.
- Ruan H, Chen X, Xie C, et al. Stapled RGD peptide enables glioma-targeted drug delivery by overcoming multiple barriers. ACS Appl Mater Interfaces. 2017;9:17745–17756.
- Xia J, Du Y, Huang L, et al. Redox-responsive micelles from disulfide bond-bridged hyaluronic acid-tocopherol succinate for the treatment of melanoma. Nanomedicine. 2018;14:713–723.
- Huo Q, Zhu J, Niu Y, et al. pH-triggered surface charge-switchable polymer micelles for the co-delivery of paclitaxel/disulfiram and overcoming multidrug resistance in cancer. Ijn. 2017;12:8631–8647.
- Cao J, Huang S, Chen Y, et al. Near-infrared light-triggered micelles for fast controlled drug release in deep tissue. Biomaterials. 2013;34:6272–6283.
- Huang Y, Dong R, Zhu X, et al. Photo-responsive polymeric micelles. Soft Matter. 2014;10:6121–6138.
- Wang Q, Liu Y, Pu C, et al. Drug-polymer interaction, pharmacokinetics and antitumor effect of PEG-PLA/Taxane derivative TM-2 micelles for intravenous drug delivery. Pharm Res. 2018;35:208.
- Zhou Y, Wen H, Gu L, et al. Aminoglucose-functionalized, redox-responsive polymer nanomicelles for overcoming chemoresistance in lung cancer cells. J Nanobiotechnology 2017;15:87.
- Decuzzi P, Ferrari M. The role of specific and non-specific interactions in receptor-mediated endocytosis of nanoparticles. Biomaterials. 2007;28:2915–2922.
- Cheng Q, Du L, Meng L, et al. The promising nanocarrier for doxorubicin and siRNA co-delivery by PDMAEMA-based amphiphilic nanomicelles. ACS Appl Mater Interfaces. 2016;8:4347–4356.
- Wang Q, Jiang H, Li Y, et al. Targeting NF-kB signaling with polymeric hybrid micelles that co-deliver siRNA and dexamethasone for arthritis therapy. Biomaterials. 2017;122:10–22.
- Yang C, Yuan C, Liu W, et al. DPD studies on mixed micelles self-assembled from MPEG-PDEAEMA and MPEG-PCL for controlled doxorubicin release. Colloids Surf B Biointerfaces. 2019;178:56–65.
- Yin H, Wang H, Li Z, et al. RNA micelles for the systemic delivery of anti-miRNA for cancer targeting and inhibition without ligand. ACS Nano. 2019;13:706–717.
- Shu Y, Yin H, Rajabi M, et al. RNA-based micelles: A novel platform for paclitaxel loading and delivery. J Control Release. 2018;276:17–29.
- Yang J, Lu W, Xiao J, et al. A positron emission tomography image-guidable unimolecular micelle nanoplatform for cancer theranostic applications. Acta Biomater. 2018;79:306–316.
- Deshantri AK, Varela Moreira A, Ecker V, et al. Nanomedicines for the treatment of hematological malignancies. J Control Release. 2018;287:194–215.
- Duong HH, Yung LY. Synergistic co-delivery of doxorubicin and paclitaxel using multi-functional micelles for cancer treatment. Int J Pharm. 2013;454:486–495.
- Muza UL, Greyling G, Pasch H. Characterization of complex polymer self-assemblies and large aggregates by multidetector thermal field-flow fractionation. Anal Chem. 2017;89:7216–7224.
- Chen L, Lee HS, Lee S. Close-packed block copolymer micelles induced by temperature quenching. Proc Natl Acad Sci USA. 2018;115:7218–7223.
- Behroozi F, Abdkhodaie MJ, Abandansari HS, et al. Engineering folate-targeting diselenide-containing triblock copolymer as a redox-responsive shell-sheddable micelle for antitumor therapy in vivo. Acta Biomater. 2018;76:239–256.
- Kim J, Kim MJ, Lee J. The critical micelle concentration of lecithin in bulk oils and medium chain triacylglycerol is influenced by moisture content and total polar materials. Food Chem. 2018;261:194–200.
- Dusa F, Chen W, Witos J, et al. Nanoplasmonic sensing and capillary electrophoresis for fast screening of interactions between phosphatidylcholine biomembranes and surfactants. Langmuir 2018;34:5889–5900.
- Pandey B, Patil N, Bhosle GS, et al. Amphiphilic glycopolypeptide star copolymer-based crosslinked nanocarriers for targeted and dual stimuli-responsive drug delivery. Bioconjug Chem. 2018.
- Chen M, Zhang Y, Chen Z, et al. Synergistic antitumor efficacy of redox and pH dually responsive micelleplexes for co-delivery of camptothecin and genes. Acta Biomater. 2017;49:444–455.
- Pan Z, Fang D, Song N, et al. Surface distribution and biophysicochemical properties of polymeric micelles bearing gemini cationic and hydrophilic groups. ACS Appl Mater Interfaces. 2017;9:2138–2149.
- Song X, Li R, Deng H, et al. Receptor mediated transcytosis in biological barrier: the influence of receptor character and their ligand density on the transmembrane pathway of active-targeting nanocarriers. Biomaterials. 2018;180:78–90.
- Vercauteren D, Rejman J, Martens TF, et al. On the cellular processing of non-viral nanomedicines for nucleic acid delivery: mechanisms and methods. J Control Release. 2012;161:566–581.
- Li Z, Cai Y, Zhao Y, et al. Polymeric mixed micelles loaded mitoxantrone for overcoming multidrug resistance in breast cancer via photodynamic therapy. Int J Nanomedicine. 2017;12:6595–6604.
- Uz M, Kalaga M, Pothuraju R, et al. Dual delivery nanoscale device for miR-345 and gemcitabine co-delivery to treat pancreatic cancer. J Control Release. 2019;294:237–246.
- Yu LY, Su GM, Chen CK, et al. Specific cancer cytosolic drug delivery triggered by reactive oxygen species-responsive micelles. Biomacromolecules. 2016;17:3040–3047.
- Synergistic effect of reduced polypeptide micelle for co-delivery of doxorubicin and TRAIL against [PMIDA27557520].pdf.
- Tian J, Xu L, Xue Y, et al. Enhancing photochemical internalization of DOX through a porphyrin-based amphiphilic block copolymer. Biomacromolecules. 2017;18:3992–4001.
- Maeda H, Takeshita J, Kanamaru R. A lipophilic derivative of neocarzinostatin. A polymer conjugation of an antitumor protein antibiotic. Int J Pept Protein Res. 2009;14:81–87.
- Wang J, Ma W, Guo Q, et al. The effect of dual-functional hyaluronic acid-vitamin E succinate micelles on targeting delivery of doxorubicin. Int J Nanomedicine. 2016;11:5851–5870.
- Hansen AE, Petersen AL, Henriksen JR, et al. Positron emission tomography based elucidation of the enhanced permeability and retention effect in dogs with cancer using copper-64 liposomes. ACS Nano. 2015;9:6985–6995.
- Khawar IA, Kim JH, Kuh HJ. Improving drug delivery to solid tumors: priming the tumor microenvironment. J Control Release. 2015;201:78–89.
- Danhier F, Danhier P, De Saedeleer CJ, et al. Paclitaxel-loaded micelles enhance transvascular permeability and retention of nanomedicines in tumors. Int J Pharm. 2015;479:399–407.
- Perry JL, Reuter KG, Luft JC, et al. Mediating passive tumor accumulation through particle size, tumor type, and location. Nano Lett. 2017;17:2879–2886.
- Yin M, Tan S, Bao Y, et al. Enhanced tumor therapy via drug co-delivery and in situ vascular-promoting strategy. J Control Release. 2017;258:108–120.
- Mochida Y, Cabral H, Kataoka K. Polymeric micelles for targeted tumor therapy of platinum anticancer drugs. Expert Opin Drug Deliv. 2017;14:1423–1438.
- Quader S, Liu X, Chen Y, et al. cRGD peptide-installed epirubicin-loaded polymeric micelles for effective targeted therapy against brain tumors. J Control Release. 2017;258:56–66.
- Zhang X, Wu Y, Zhang M, et al. Sodium cholate-enhanced polymeric micelle system for tumor-targeting delivery of paclitaxel. Int J Nanomedicine. 2017;12:8779–8799.
- Yao Q, Choi JH, Dai Z, et al. Improving tumor specificity and anticancer activity of dasatinib by dual-targeted polymeric micelles. ACS Appl Mater Interfaces. 2017;9:36642–36654.
- Xiao Y, Wang S, Zong Q, et al. Co-delivery of metformin and paclitaxel via folate-modified pH-sensitive micelles for enhanced anti-tumor efficacy. AAPS PharmSciTech. 2018;19:2395–2406.
- Sun F, Yu Y, Yang Z, et al. Hyaluronic acid-endostatin2-alft1 (HA-ES2-AF) nanoparticle-like conjugate for the target treatment of diseases. J Control Release. 2018; 288:1–13.
- Kang T, Jiang M, Jiang D, et al. Enhancing glioblastoma-specific penetration by functionalization of nanoparticles with an iron-mimic peptide targeting transferrin/transferrin receptor complex. Mol Pharmaceutics. 2015;12:2947–2961.
- Charbgoo F, Alibolandi M, Taghdisi SM, et al. MUC1 aptamer-targeted DNA micelles for dual tumor therapy using doxorubicin and KLA peptide. Nanomedicine. 2018;14:685–697.
- Yang SD, Zhu WJ, Zhu QL, et al. Binary-copolymer system base on low-density lipoprotein-coupled N-succinyl chitosan lipoic acid micelles for co-delivery MDR1 siRNA and paclitaxel, enhances antitumor effects via reducing drug. J Biomed Mater Res. 2017;105:1114–1125.
- Maurer AH, Elsinga P, Fanti S, et al. Imaging the folate receptor on cancer cells with 99mTc-etarfolatide: properties, clinical use, and future potential of folate receptor imaging. J Nucl Med. 2014;55:701–704.
- Tang Y, Li Y, Xu R, et al. Self-assembly of folic acid dextran conjugates for cancer chemotherapy. Nanoscale. 2018;10:17265–17274.
- Liu T, Wu X, Wang Y, et al. Folate-targeted star-shaped cationic copolymer co-delivering docetaxel and MMP-9 siRNA for nasopharyngeal carcinoma therapy. Oncotarget. 2016;7:42017–42030.
- Orian-Rousseau V, Ponta H. Perspectives of CD44 targeting therapies. Arch Toxicol. 2015;89:3–14.
- Schmitt M, Metzger M, Gradl D, et al. CD44 functions in Wnt signaling by regulating LRP6 localization and activation. Cell Death Differ. 2015;22:677–689.
- D'Arena G, Calapai G, Deaglio S. Anti-CD44 mAb for the treatment of B-cell chronic lymphocytic leukemia and other hematological malignancies: evaluation of WO2013063498. Expert Opin Ther Pat. 2014;24:821–828.
- Ma W, Guo Q, Li Y, et al. Co-assembly of doxorubicin and curcumin targeted micelles for synergistic delivery and improving anti-tumor efficacy. Eur J Pharm Biopharm. 2017;112:209–223.
- Shigeta S, Toyoshima M, Kitatani K, et al. Transferrin facilitates the formation of DNA double-strand breaks via transferrin receptor 1: the possible involvement of transferrin in carcinogenesis of high-grade serous ovarian cancer. Oncogene. 2016;35:3577.
- Zou W, Sarisozen C, Torchilin VP. The reversal of multidrug resistance in ovarian carcinoma cells by co-application of tariquidar and paclitaxel in transferrin-targeted polymeric micelles. J Drug Target. 2017;25:225–234.
- Kang SJ, Jeong HY, Kim MW, et al. Anti-EGFR lipid micellar nanoparticles co-encapsulating quantum dots and paclitaxel for tumor-targeted theranosis. Nanoscale. 2018;10:19338–19350.
- Alibakhshi A, Abarghooi Kahaki F, Ahangarzadeh S, et al. Targeted cancer therapy through antibody fragments-decorated nanomedicines. J Control Release. 2017;268:323–334.
- Ahangarzadeh S, Bandehpour M, Kazemi B. Selection of single-chain variable fragments specific for Mycobacterium tuberculosis ESAT-6 antigen using ribosome display. Iran J Basic Med Sci. 2017;20:327–333.
- Richards DA, Maruani A, Chudasama V. Antibody fragments as nanoparticle targeting ligands: a step in the right direction. Chem Sci. 2017;8:63–77.
- Sarisozen C, Dhokai S, Tsikudo EG, et al. Nanomedicine based curcumin and doxorubicin combination treatment of glioblastoma with scFv-targeted micelles: In vitro evaluation on 2D and 3D tumor models. Eur J Pharm Biopharm. 2016;108:54–67.
- Wang Z, Li X, Wang D, et al. Concurrently suppressing multidrug resistance and metastasis of breast cancer by co-delivery of paclitaxel and honokiol with pH-sensitive polymeric micelles. Acta Biomater. 2017;62:144–156.
- Maiti C, Parida S, Kayal S, et al. Redox-responsive core-cross-linked block copolymer micelles for overcoming multidrug resistance in cancer cells. ACS Appl Mater Interfaces. 2018;10:5318–5330.
- Qiu L, Zhu M, Gong K, et al. pH-triggered degradable polymeric micelles for targeted anti-tumor drug delivery. Mater Sci Eng C Mater Biol Appl. 2017;78:912–922.
- Liu Y, Yang J, Wang X, et al. In vitro and in vivo evaluation of redox-responsive sorafenib carrier nanomicelles synthesized from poly (acryic acid)-cystamine hydrochloride-D-alpha-tocopherol succinate. J Biomater Sci Polym Ed. 2016;27:1729–1747.
- Fisher DT, Appenheimer MM, Evans SS. The two faces of IL-6 in the tumor microenvironment. Semin Immunol. 2014;26:38–47.
- Chen Q, Zheng J, Yuan X, et al. Folic acid grafted and tertiary amino based pH-responsive pentablock polymeric micelles for targeting anticancer drug delivery. Mater Sci Eng C Mater Biol Appl. 2018;82:1–9.
- Guan J, Zhou ZQ, Chen MH, et al. Folate-conjugated and pH-responsive polymeric micelles for target-cell-specific anticancer drug delivery. Acta Biomater. 2017;60:244–255.
- Li M, Gao M, Fu Y, et al. Acetal-linked polymeric prodrug micelles for enhanced curcumin delivery. Colloids Surf B Biointerfaces. 2016;140:11–18.
- Zhao D, Li B, Han J, et al. PH responsive polypeptide based polymeric micelles for anticancer drug delivery. J Biomed Mater Res. 2015;103:3045–3053.
- Yan G, Wang J, Hu L, et al. Stepwise targeted drug delivery to liver cancer cells for enhanced therapeutic efficacy by galactose-grafted, ultra-pH-sensitive micelles. Acta Biomater. 2017;51:363–373.
- Ma Y, Fan X, Li L. pH-sensitive polymeric micelles formed by doxorubicin conjugated prodrugs for co-delivery of doxorubicin and paclitaxel. Carbohydr Polym. 2016;137:19–29.
- Xu X, Li L, Zhou Z, et al. Dual-pH responsive micelle platform for co-delivery of axitinib and doxorubicin. Int J Pharm. 2016;507:50–60.
- Nie SY, Lin WJ, Yao N, et al. Drug release from pH-sensitive polymeric micelles with different drug distributions: insight from coarse-grained simulations. ACS Appl Mater Interfaces. 2014;6:17668.
- Xiong D, Zhang X, Peng S, et al. Smart pH-sensitive micelles based on redox degradable polymers as DOX/GNPs carriers for controlled drug release and CT imaging. Colloids Surf B Biointerfaces. 2018;163:29–40.
- Liu X, Li Y, Tan X, et al. Multifunctional hybrid micelles with tunable active targeting and acid/phosphatase-stimulated drug release for enhanced tumor suppression. Biomaterials. 2018;157:136–148.
- Yan H, Han Z, Li K, et al. Molecular dynamics simulation of the pH-induced structural transitions in CTAB/NaSal solution. Langmuir. 2018;34:351–358.
- Yu H, Guo C, Feng B, et al. Triple-layered pH-responsive micelleplexes loaded with siRNA and cisplatin prodrug for NF-Kappa B targeted treatment of metastatic breast cancer. Theranostics 2016;6:14–27.
- Han R, Sun Y, Kang C, et al. Amphiphilic dendritic nanomicelle-mediated co-delivery of 5-fluorouracil and doxorubicin for enhanced therapeutic efficacy. J Drug Target. 2017;25:140–148.
- Mao J, Li Y, Wu T, et al. A simple dual-pH responsive prodrug-based polymeric micelles for drug delivery. ACS Appl Mater Interfaces. 2016;8:17109–17117.
- Nangia S, Sureshkumar R. Effects of nanoparticle charge and shape anisotropy on translocation through cell membranes. Langmuir. 2012;28:17666–17671.
- Ma SF, Nishikawa M, Katsumi H, et al. Cationic charge-dependent hepatic delivery of amidated serum albumin. J Control Release. 2005;102:583–594.
- Zhu Y, Meng T, Tan Y, et al. Negative surface shielded polymeric micelles with colloidal stability for intracellular endosomal/lysosomal escape. Mol Pharmaceutics. 2018;5;15:5374–5386.
- Li Y, Yang H, Yao J, et al. Glutathione-triggered dual release of doxorubicin and camptothecin for highly efficient synergistic anticancer therapy. Colloids Surf B Biointerfaces. 2018;169:273–279.
- Kim HC, Kim E, Ha TL, et al. Thiol-responsive gemini poly(ethylene glycol)-poly(lactide) with a cystine disulfide spacer as an intracellular drug delivery nanocarrier. Colloids Surf B Biointerfaces. 2015;127:206–212.
- Ko NR, Oh JK. Glutathione-triggered disassembly of dual disulfide located degradable nanocarriers of polylactide-based block copolymers for rapid drug release. Biomacromolecules. 2014;15:3180–3189.
- Li J, Xu R, Lu X, et al. A simple reduction-sensitive micelles co-delivery of paclitaxel and dasatinib to overcome tumor multidrug resistance. Int J Nanomedicine. 2017;12:8043–8056.
- Zhang J, Tang H, Shen Y, et al. Shell-sheddable poly(N-2-hydroxypropyl methacrylamide) polymeric micelles for dual-sensitive release of doxorubicin. Macromol Rapid Commun. 2018;39:1800139.
- Hu C, Gu F, Tai Z, et al. Synergistic effect of reduced polypeptide micelle for co-delivery of doxorubicin and TRAIL against drug-resistance in breast cancer. Oncotarget. 2016;7:61832–61844.
- Hu J, Zhang G, Liu S. Enzyme-responsive polymeric assemblies, nanoparticles and hydrogels. Chem Soc Rev. 2012;41:5933–5949.
- Fletcher NM, Belotte J, Saed MG, et al. Specific point mutations in key redox enzymes are associated with chemoresistance in epithelial ovarian cancer. Free Radic Biol Med. 2017;102:122–132.
- Xie C, Powell C, Yao M, et al. Ubiquitin-conjugating enzyme E2C: a potential cancer biomarker. Int J Biochem Cell Biol. 2014;47:113–117.
- Jayatilaka H, Umanzor FG, Shah V, et al. Tumor cell density regulates matrix metalloproteinases for enhanced migration. Oncotarget. 2018; 9:32556–32569.
- Isaacson KJ, Martin Jensen M, Subrahmanyam NB, et al. Matrix-metalloproteinases as targets for controlled delivery in cancer: an analysis of upregulation and expression. J Control Release. 2017;259:62–75.
- Zhu L, Perche F, Wang T, et al. Matrix metalloproteinase 2-sensitive multifunctional polymeric micelles for tumor-specific co-delivery of siRNA and hydrophobic drugs. Biomaterials. 2014;35:4213–4222.
- Salzano G, Costa DF, Sarisozen C, et al. Mixed nanosized polymeric micelles as promoter of doxorubicin and miRNA-34a co-delivery triggered by dual stimuli in tumor tissue. Small. 2016;12:4837–4848.
- Panja S, Dey G, Bharti R, et al. Tailor-made temperature-sensitive micelle for targeted and on-demand release of anticancer drugs. ACS Appl Mater Interfaces. 2016;8:12063–12074.
- Huang P, Song H, Zhang Y, et al. FRET-enabled monitoring of the thermosensitive nanoscale assembly of polymeric micelles into macroscale hydrogel and sequential cognate micelles release. Biomaterials. 2017;145:81–91.
- Sheu MT, Jhan HJ, Su CY, et al. Codelivery of doxorubicin-containing thermosensitive hydrogels incorporated with docetaxel-loaded mixed micelles enhances local cancer therapy. Colloids Surf B Biointerfaces. 2016;1;143:260–270.
- Lee S-Y, Choi S-J, Seo S-J, et al. Shell cross-linked polyethylenimine-modified micelles for temperature-triggered drug release and gene delivery. RSC Adv. 2014;4:57702–57708.
- Kim DH, Hwang HS, Na K. Photoresponsive micelle-incorporated doxorubicin for chemo-photodynamic therapy to achieve synergistic antitumor effects. Biomacromolecules. 2018;19:3301–3310.
- Li H, Yan K, Shang Y, et al. Folate-bovine serum albumin functionalized polymeric micelles loaded with superparamagnetic iron oxide nanoparticles for tumor targeting and magnetic resonance imaging. Acta Biomater. 2015;15:117–126.
- Wu P, Jia Y, Qu F, et al. Ultrasound-responsive polymeric micelles for sonoporation-assisted site-specific therapeutic action. ACS Appl Mater Interfaces. 2017;9:25706–25716.
- Wei X, Liu L, Guo X, et al. Light-activated ROS-responsive nanoplatform codelivering apatinib and doxorubicin for enhanced chemo-photodynamic therapy of multidrug-resistant tumors. ACS Appl Mater Interfaces. 2018;10:17672–17684.
- Zhang L, Qin Y, Zhang Z, et al. Dual pH/reduction-responsive hybrid polymeric micelles for targeted chemo-photothermal combination therapy. Acta Biomater. 2018;75:371–385.
- Zhao D, Wu J, Li C, et al. Precise ratiometric loading of PTX and DOX based on redox-sensitive mixed micelles for cancer therapy. Colloids Surf B Biointerfaces. 2017;155:51–60.
- Wu Y, Zhang Y, Zhang W, et al. Reversing of multidrug resistance breast cancer by co-delivery of P-gp siRNA and doxorubicin via folic acid-modified core-shell nanomicelles. Colloids Surf B Biointerfaces. 2016;138:60–69.
- Wang J, Li Y, Wang L, et al. Comparison of hyaluronic acid-based micelles and polyethylene glycol-based micelles on reversal of multidrug resistance and enhanced anticancer efficacy in vitro and in vivo. Drug Deliv. 2018;25:330–340.
- Sarisozen C, Abouzeid AH, Torchilin VP. The effect of co-delivery of paclitaxel and curcumin by transferrin-targeted PEG-PE-based mixed micelles on resistant ovarian cancer in 3-D spheroids and in vivo tumors. Eur J Pharm Biopharm. 2014;88:539–550.
- Zhao J, Mi Y, Feng SS. Targeted co-delivery of docetaxel and siPlk1 by herceptin-conjugated vitamin E TPGS based immunomicelles. Biomaterials. 2013;34:3411–3421.
- Zhu WJ, Yang SD, Qu CX, et al. Low-density lipoprotein-coupled micelles with reduction and pH dual sensitivity for intelligent co-delivery of paclitaxel and siRNA to breast tumor. Int J Nanomedicine. 2017;12:3375–3393.
- Suo A, Qian J, Zhang Y, et al. Comb-like amphiphilic polypeptide-based copolymer nanomicelles for co-delivery of doxorubicin and P-gp siRNA into MCF-7 cells. Mater Sci Eng C Mater Biol Appl. 2016;62:564–573.
- Li M, Tang Z, Lv S, et al. Cisplatin crosslinked pH-sensitive nanoparticles for efficient delivery of doxorubicin. Biomaterials. 2014;35:3851–3864.
- Kinoh H, Miura Y, Chida T, et al. Nanomedicines eradicating cancer stem-like cells in vivo by pH-triggered intracellular cooperative action of loaded drugs. ACS Nano. 2016;10:5643–5655.
- Sun J, Liu Y, Chen Y, et al. Doxorubicin delivered by a redox-responsive dasatinib-containing polymeric prodrug carrier for combination therapy. J Control Release. 2017;258:43–55.
- Guo Y, He W, Yang S, et al. Co-delivery of docetaxel and verapamil by reduction-sensitive PEG-PLGA-SS-DTX conjugate micelles to reverse the multi-drug resistance of breast cancer. Colloids Surf B Biointerfaces. 2017;1;151:119–127.
- Seo SJ, Lee SY, Choi SJ, et al. Tumor-targeting co-delivery of drug and gene from temperature-triggered micelles. Macromol Biosci. 2015;15:1198–1204.
- Ruttala HB, Chitrapriya N, Kaliraj K, et al. Facile construction of bioreducible crosslinked polypeptide micelles for enhanced cancer combination therapy. Acta Biomater. 2017;63:135–149.
- Yan T, Li D, Li J, et al. Effective co-delivery of doxorubicin and curcumin using a glycyrrhetinic acid-modified chitosan-cystamine-poly(epsilon-caprolactone) copolymer micelle for combination cancer chemotherapy. Colloids Surf B Biointerfaces. 2016;1;145:526–538.