Abstract
The medical community has expressed significant interest in the treatment of cartilage defect. Successful repair of articular cartilage defects remains a challenge in clinics. Due to the huge advantages of 3D micro/nanomaterials, 3D artificial micro/nano scaffolds have been widely developed and explored in the tissue repair of articular joints. In this study, chondrocyte/osteoblast-loaded β-tricalcium phosphate (β-TCP) bioceramic scaffold and chondrocyte-loaded β-TCP bioceramic scaffold were prepared by micromass stem cell culture and bioreactor-based cells-loaded scaffold culture for articular cartilage defect treatment. The results demonstrate chondrocyte and osteoblast can be successfully induced from allogeneic bone marrow stromal cells using micromass stem cell culture. Further, chondrocyte-loaded β-TCP scaffold and osteoblast-loaded β-TCP scaffold can be successfully prepared by bioreactor-based cells-loaded scaffold culture. Finally, the scaffolds are applied for Beagle articular cartilage defect treatment. The relative cartilage regeneration abilities on Beagle femoral trochleae were as follows: Chondrocyte/osteoblast-loaded β-TCP bioceramic scaffold group > chondrocyte-loaded β-TCP bioceramic scaffold group > β-TCP bioceramic scaffold. Therefore, micromass stem cell culture and bioreactor-based cells-loaded scaffold culture can be applied to prepare chondrocyte/osteoblast-loaded β-TCP bioceramic scaffold for articular cartilage defect treatment. It suggests allogenic chondrocyte/osteoblast-loaded β-TCP bioceramic scaffold could be potentially used in the treatment of patients with cartilage defects.
Introduction
Articular cartilage is a non-vascular tissue, which is mainly composed of chondrocyte and cartilage extracellular matrix. Injured articular cartilage is a common and increasing disease in the field of orthopedics, articular wear, trauma, etc [Citation1]. It can lead to disability and reduced quality of life. Although articular cartilage injuries are common, injured cartilage has a limited ability to heal [Citation2]. Articular cartilage injuries with a diameter of more than 4 mm cannot spontaneously heal. Reconstruction of articular cartilage defects with a large diameter still remains one of the most difficult problems for joint surgeons [Citation3,Citation4]. Currently, traditional conservative treatment is mainly applied for mild articular cartilage injuries and artificial joint replacement is mainly applied for severe articular cartilage injuries in clinics. These treatments have their disadvantages such as restricted activity, limited life of the server, and potential infection. Many types of research demonstrated chondrocyte transplantation is a promising method for articular cartilage defect treatment [Citation5–7]. Therefore, it is necessary to explore the clinical use of chondrocyte-loaded tissue engineering artificial bone with good ability of chondrogenesis.
3D artificial micro/nano scaffolds have already developed for bone regeneration [Citation8,Citation9], load-bearing bone defect repair [Citation10,Citation11] and large osteochondral defects in the articular joints [Citation12]. Autologous chondrocytes-loaded micro/nano β-tricalcium phosphate (β-TCP) bioceramic scaffold has been explored in the repair of osteochondral defects and showed good regeneration behaviors [Citation13–15]. However, limited autologous chondrocyte availability, the need for additional surgery, the formation of donor site defects, donor site pain, possible blood loss and infection, and impracticability of utilizing autologous bone in the clinics have restricted their potential application. Moreover, the application of allogenic chondrocyte is also not ideal in clinics because of many disadvantages for the donor person such as limited allogenic chondrocyte availability, the need for additional surgery, the formation of surgical defects, donor person site pain, possible blood loss and infection, and impracticability of utilizing allogenic chondrocyte in the clinics. Therefore, it is necessary to develop efficient allogenic stem cell sources and explore the preparation of chondrocyte-loaded 3D bioceramic scaffolds for articular cartilage defect treatment.
Bone marrow stromal cells (BMSCs) are important cell sources for bone tissue engineering [Citation8,Citation16]. BMSCs can be extracted from allogeneic bone marrow by a minimally invasive surgery, which has many advantages for the donor person such as short treatment time, smaller incision, less pain, less blood loss, shorter recovery time, and shorter hospitalization compared with traditional surgical treatment [Citation17,Citation18]. They can be differentiated into a number of cell lineages such as chondrocyte and osteoblasts [Citation19]. Human BMSCs-loaded 3D porous polymer scaffolds have been developed for cartilage tissue engineering [Citation20,Citation21]. A bioreactor has been developed for perfusing cells in 3D tissue engineered scaffolds and the results demonstrated that it was an efficient way to perfuse cells in 3D porous scaffolds [Citation22–24]. 2D monolayer chondrocyte culture has a tendency to undergo dedifferentiation and have a higher probability to differentiate and form hypertrophic chondrocytes [Citation25,Citation26]. High density 3D micromass chondrocyte induction has been shown to secrete higher amounts of collagenous matrix and showed better cell differentiation behaviors [Citation27,Citation28]. Therefore, it would be a good idea to explore the combination of 3D micromass culture with bioceramic scaffolds. In this work, in order to obtain good treatment behavior of chondrocyte and osteoblast-loaded β-TCP bioceramic scaffolds for articular cartilage defect treatment, we try to explore the application of 3D micromass stem cell culture for chondrocyte and osteoblast induction prior to bioreactor-based cells-loaded scaffold culture.
Materials and methods
Chemicals
Percoll diluted solution was prepared by mixing 9 volume of Percoll solution (Pharmaeia, Uppsala, Sweden) and one volume of 10X PBS. Complete cell culture medium consists of 90% low-glucose Dulbecco’s modified Eagle’s medium (L-DMEM, HyClone Inc, USA), 10% fetal bovine serum (FBS; Gibco, Carlsbad, USA), 100 U/mL penicillin, and 100 μg/mL streptomycin. Osteoblast induction solution was prepared by adding dexamethasone (100 nM), β-glycerol phosphate sodium (10 mM), and vitamin C (0.05 mM) into Hepes-buffered Dulbecco’s modified Eagle’s medium (H-DMEM, HyClone Inc, USA) with 10% FBS. Chondrocyte induction solution was prepared by adding bone morphogenetic protein-2 (BMP-2, 200 ng/mL), insulin-like growth factor 1 (IGF-1, 10 ng/mL), transferrin (6.25 μg/mL), dexamethasone (0.1 μM), and vitamin C (50 μg/mL) into H-DMEM with 10% FBS.
Extraction, isolation, and culture of BMSCs
In order to prepare BMSCs, three healthy Beagles with an age of ∼4–10 months and a weight of 7.2–10.1 kg were used. The Beagles were anaesthetized. The wool on the Beagle iliac bone was shaved, and the areas to be used for the surgeries were disinfected with tincture of iodine. The iliac bone was punctured by using a skin incision trocar with a diameter of 1.5 mm. Then 6–7 mL bone marrow was extracted and stored in a 50 mL centrifuge tube with 0.4 mL Heparin (3000 U/mL) for each Beagle. The extracted bone marrow was mixed with D-Hanks solution. The supernatant and adipose tissues were removed. The solution was re-dispersed and was transferred into 1.073 g/mL Percoll diluted solution (3:2, v/v). The mixture solution was gently vortexed and then was centrifuged at 2800 rpm for 20 min. BMSCs were taken out and transferred into D-hanks solution (1:1, v/v). The mixture solution was gently vortexed and then was centrifuged at 1000 rpm for 8 min. The BMSCs were seeded in complete cell culture medium and cultured in a humidity-saturated incubator with 5% CO2 at 37 °C. The culture medium was half-replaced on day 4 and fully replaced every 2 days after 7 days. The cell growth was examined by inverted optical microscopy. When cultured cells reached more than 90% confluence at day 12–14, adherent cells were detached with 0.05% trypsin-EDTA (Gibco, USA) and replated (passaged) at a density of 1 × 106 per 175 cm2 flask until processing for infusion [Citation29].
Micromass stem cell culture
The third-passage cells were detached with 0.05% trypsin-EDTA and dispersed in complete cell culture medium at a density of 1 × 106 per mL. 6 drops (100 μL per drop) of cell suspension was placed onto 60 mm petri dish with a space of 8 mm and was cultured in a humidity-saturated incubator with 5% CO2 at 37 °C for 2 h. A total of 9 Petri dishes were randomly divided into three groups: chondrocyte induction solution, osteoblast induction group and control media were added to these three groups, respectively. Then, these samples were incubated in a humidity-saturated incubator with 5% CO2 at 37 °C. The culture media were fully replaced every 2 days. The cell growth was examined by inverted optical microscopy.
Chondrocyte and osteoblast suspensions were prepared as follows: After 14 days of induction, the media in the chondrocyte and osteoblast groups were removed and washed by D-hanks solution three times. Then, the cells were detached with 0.05% trypsin-EDTA and dispersed in D-hanks solution in centrifugal tubes. The cell suspensions in centrifugal tubes were centrifuged at 1000 rpm for 8 min. The supernatants were removed and 2 mL H-DMEM with 10% FBS was added into the centrifugal tubes. The tubes were gently vortexed to prepare chondrocyte and osteoblast suspensions. The cell density was adjusted to 4 × 106 per mL by adding an appropriate volume of H-DMEM with 10% FBS.
After 14 days micromass culture, the chondrocyte induction group and control group were examined by glycosaminoglycan content analysis and cell viability assay. Glycosaminoglycan content analysis was performed using a modification of the dimethylmethylene blue method [Citation30]. The cell viability was examined by MTT assay [Citation31].
Bioreactor-based construction of chondrocyte-loaded or osteoblast-loaded β-TCP bioceramic scaffolds
β-TCP bioceramic rods (Shanghai Bio-lu Biomaterials Co., Ltd., China) with a diameter of 0.45 cm and a height of 0.45 cm were put into the wells of 12-well cell culture plates and were disinfected by ultraviolet irradiation for 1 h and immersed into 75% ethanol overnight. Then, the bioceramic rods were washed by PBS six times and then immersed into H-DMEM overnight. Six bioceramic rods were randomly divided into two groups: (a) chondrocyte-loaded β-TCP bioceramic scaffold group; and (b) osteoblast/β-TCP bioceramic scaffold group. For each group, 2 mL cell suspension was added onto β-TCP bioceramic rod, which was turned upside down to make sure that cells attached on every surface of the rod. The rods were incubated in a humidity-saturated incubator with 5% CO2 at 37 °C for 4 h. After that, the rods were put in a custom-designed bioreactor with a flow speed of 3 mL/min for H-DMEM with 10% FBS. The bioreactor was put in a humidity-saturated incubator with 5% CO2 at 37 °C. The culture media were fully replaced every 6 days.
The scaffolds after 21 days of incubation were examined by JSM-T300 (JOEL, Japan) SEM. Briefly, the cells in the scaffolds were fixed by 2.5% glutaraldehyde (pH 7.2–7.4) for 30 min. The scaffolds were washed thrice by immersing in fresh PBS for 15 min. Then, the scaffolds were fixed by 1% osmium tetroxide solution (pH 7.2–7.4) for 60 min and were washed thrice by immersing in fresh PBS for 15 min. The fixed scaffolds were dehydrated by immersion in graded acetone solutions (30, 50, 70, 80, 90, 100 and 100%) for 7 min in each solution. The scaffolds were immersed into dimethyl sulfoxide and then transferred into liquid nitrogen. After that, the scaffolds were dried under vacuum and were coated with an ultrathin gold layer. Finally, the scaffolds were observed by SEM at an accelerated volage of 10 kV. Three specimens were observed for each sample.
Implantation of bioceramic scaffolds into beagle femoral trochleae
In this work, healthy Beagles with an age of 10–12 months and a weight of 8.1–10.2 kg were employed as animal models. A total of 9 beagles were obtained from the Animal Center in Shandong Provincial Hospital Affiliated to Shandong University. The use of the Beagles and the experimental procedures in this study were approved by Shandong Provincial Hospital Affiliated to Shandong University’s Animal Care and Use Committee.
The Beagles were anaesthetized and the wool on the interior of the knee joint was shaved. Then, the areas to be used for the surgeries were disinfected with tincture of iodine. The skin and subcutaneous fascia were incised. The knee joint was incised through the interior of kneecap. The kneecap tendon and quadriceps femoris tendon were stretched out. The kneecap was stretched to form dislocation and the femoral trochlea was exposed. Three cylinder-like articular cartilage defects with a diameter of about 0.45 cm and a depth of about 0.45 cm were constructed in the femoral trochlea. Chondrocyte/osteoblast-loaded β-TCP bioceramic scaffold, chondrocyte-loaded β-TCP bioceramic scaffold, β-TCP bioceramic scaffold were randomly implanted into these three defects of each femoral trochlea. Then, the kneecap was restored, knee capsule and muscular fascia tissue were sutured, and the skin incision was sutured. Gentian violet was spread on the skin incision. The Beagles were cultured at 15–20 °C and received intramuscular injection of penicillin 800,000 IU once a day in the first three days to prevent infection. The Beagles (3 of each group) were sacrificed at 12 weeks. The treatments in femoral trochlea were evaluated by both naked eyes/digital cameras and histology.
Histological observation
The Beagles were sacrificed at 12 weeks postimplantation for histological observation. The femoral trochleae were harvested from the Beagle legs (the soft tissues were removed from the cartilages). The bioceramic scaffolds and the surrounding host cartilage tissues were excised and fixed in a 10% formalin solution at 4 °C for 24 h. The specimens were decalcified in Formical-4 (formalin and formic acid; Decal Corporation, Tallman, NY, USA) for 24 h. After washing with water, fixed specimens were dehydrated by immersion in graded ethanol solutions (70, 75, 80, 85, 90, 95, and 100%) for 24 h in each solution. After dehydration, specimens were embedded in the medium of poly(methyl methacrylate) (Merck, Germany) and sectioned with a microtome (Leica, SP1600, Leica SP1600) equipped with sturdy tungsten carbide knives. The sections were polished and were imaged with a digital camera.
Results and discussion
Induction of chondrocyte and osteoblast from beagle BMSCs from marrow based on micromass stem cell culture
Healthy Beagles was applied to extract bone marrow. Then, BMSCs were isolated and were cultured. The primary cells () and passage cells () showed good cell shapes and good cell growth ability. The third-passage cells were detached from cell culture bottle wall for micromass stem cell culture. After 14 days micromass culture, the cells were examined by immunochemical staining observation (), MTT assay (), glycosaminoglycan content analysis ().
Table 1. Comparisons of chondrocyte amounts, absorbance of MTT assay, and glycosaminoglycan contents between chondrocyte induction group and control group.
Type II collagen is mainly produced by chondrocytes. Chondrocyte amounts were counted () and showed significant differences between chondrocyte group and control group. Glycosaminoglycan is mainly secreted by chondrocytes. Glycosaminoglycan content analysis () by a modification of the dimethylmethylene blue method showed significant differences between chondrocyte group and control group. Further, the MTT assay was applied to analyze cell viability. The results () showed significant differences between chondrocyte group and control group, which suggested the chondrocytes had good cell activity. Therefore, these results confirmed the successful induction of chondrocytes from BMSCs and the induced chondrocytes had good viability.
Preparation method of chondrocyte-loaded and osteoblast-loaded β-TCP bioceramic scaffolds using bioreactor-based cells-loaded scaffold culture method
A custom-design bioreactor system () was applied for the preparation of cells-loaded scaffolds [Citation23]. It consists of a bioreactor vessel, peristaltic pump, air filter, three-way valve, media bottle, and two tubes to connect bioreactor vessel and media bottle. β-TCP bioceramic rods with a diameter of 0.45 cm and a height of 0.45 cm were applied as cell scaffolds. After 14 days of micromass culture of chondrocyte and osteoblast induction, the cells were detached and were explored for the preparation of cells-loaded β-TCP scaffolds. The chondrocyte-loaded β-TCP scaffold or osteoblast-loaded β-TCP scaffold were put in the bioreactor vessel. The media was forced to flow between the media bottle and bioreactor vessel by a peristaltic pump with a speed of 3.0 mL/min. When the system is assembled, it was put in a humidity-saturated incubator with 5% CO2 at 37 °C for the cells-loaded scaffold preparation.
Figure 2. Schematic (A) and experimental setup (B) of a custom-design bioreactor system. It consists of bioreactor vessel, peristaltic pump, air filter, two tubes (tube 1 and tube 2), three way valve and media bottle. These parts are indicated in the figure. It is located in a humidity-saturated incubator with 5% CO2 at 37 °C and is used for cells-loaded scaffold culture.
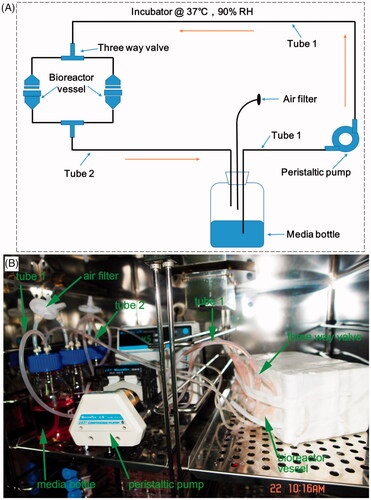
After 21 days bioreactor-based culture, the cells-loaded β-TCP scaffolds were taken out and examined by scanning electron microscopy (SEM), as shown in . β-TCP scaffolds without cell culture showed they had many pores with a diameter of 200–600 μm () and the surface at microscale () showed β-TCP scaffolds consisted of microparticles with a diameter of 1–10 μm. This is consistent with the previous report about β-TCP scaffolds [Citation32]. Chondrocytes adhered, spread, and proliferation well () on the surface of β-TCP scaffolds. Osteoblast adhered, spread, and proliferation well () on the surface of β-TCP scaffolds. Therefore, bioreactor-based cells-loaded scaffold culture method could be applied to successfully fabricate chondrocyte-loaded and osteoblast-loaded β-TCP scaffolds.
In vivo behaviors of allogenic chondrocyte/osteoblast-loaded β-TCP bioceramic scaffolds in beagle articular cartilage defect model
Biorector-based cells-loaded scaffold culture method was applied to prepare chondrocyte/osteoblast-loaded β-TCP bioceramic scaffold and chondrocyte-loaded β-TCP bioceramic scaffold. After 21 days bioreactor-based culture, these scaffolds were applied to treat Beagle articular cartilage defect model.
Healthy Beagles were applied to prepare articular cartilage defect model. Three cylinder-like articular cartilage defects with a diameter of about 0.45 cm and a depth of about 0.45 cm were constructed in the femoral trochlea (). Then, chondrocyte/osteoblast-loaded β-TCP bioceramic scaffold, chondrocyte-loaded β-TCP bioceramic scaffold, β-TCP bioceramic scaffold were randomly implanted into these three defects (). Then, the kneecap was restored, knee capsule and muscular fascia tissue were sutured, and the skin incision was sutured. After 12 weeks of animal culture, the Beagles were sacrificed and the treatments in femoral trochlea were evaluated by both naked eyes/digital cameras and histology.
Figure 4. General observation of articular cartilage defects (A), implantation of bioceramic scaffolds (B), and articular cartilage regeneration after 12 weeks postimplantation (C) in Beagle femoral trochleae. (a): chondrocyte/osteoblast-loaded β-TCP bioceramic scaffold group, (b): chondrocyte-loaded β-TCP bioceramic scaffold group, and (c): β-TCP bioceramic scaffold group. n = 3.
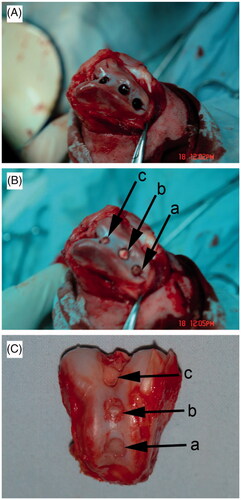
General observation of articular cartilage regeneration () showed there was no evidence of swelling and effusion of the knee joints. There was also no evidence of infection on the knee joints. Moreover, no synovial hyperplasia and cartilage destruction were found in operative joints. However, chondrocyte/osteoblast-loaded β-TCP bioceramic scaffold group and chondrocyte-loaded β-TCP bioceramic scaffold group showed better articular cartilage regeneration ability than β-TCP bioceramic scaffold group.
Histological observation of these three groups was performed by naked eyes/digital cameras. As shown in , chondrocyte/osteoblast-loaded β-TCP bioceramic scaffold group showed the new cartilage formed very dense structure in the defect area and connected very well to the surrounding host cartilage tissues (The borderline was not very clear). Compared with the surrounding cartilage and subchondral bone, the regeneration percentages of newborn cartilage and subchondral bone were 79 ± 10% and 91 ± 3%, respectively. As shown in , chondrocyte-loaded β-TCP bioceramic scaffold group showed the defect depth was shortened and the new cartilage formed dense structure in the defect area. The borderline between defect (scaffold) and the surrounding host cartilage was clear. Compared with the surrounding cartilage and subchondral bone, the regeneration percentages of newborn cartilage and subchondral bone were 74 ± 5% and 70 ± 5%, respectively. As shown in β-TCP bioceramic scaffold group showed the defect depth was shortened and the new cartilage formed dense structure in the defect area. Compared with the surrounding cartilage and subchondral bone, the regeneration percentages of newborn cartilage and subchondral bone were 0% and 58 ± 4%, respectively. Moreover, the relative cartilage regeneration abilities on Beagle femoral trochleae were as follows: Chondrocyte/osteoblast-loaded β-TCP bioceramic scaffold group > Chondrocyte-loaded β-TCP bioceramic scaffold group > β-TCP bioceramic scaffold. It suggested the co-presence of both chondrocyte and osteoblast might be more suitable for cartilage regeneration.
Figure 5. Histological observation of Beagle femoral trochleae after 12 weeks postimplantation. (A) Chondrocyte/osteoblast-loaded β-TCP bioceramic scaffold group. (B) Chondrocyte-loaded β-TCP bioceramic scaffold group. (C) β-TCP bioceramic scaffold. Arrows indicate the cartilage defects where the scaffolds were implanted.
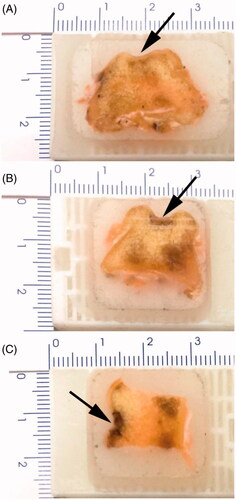
In this work, the articular cartilage defect treatment was explored by allogenic chondrocyte/osteoblast-loaded β-TCP bioceramic scaffolds. Firstly, chondrocyte and osteoblast were successfully induced from dog BMSCs from allogenic marrow based on micromass stem cell culture. Then, chondrocyte-loaded and osteoblast-loaded β-TCP bioceramic scaffolds were successfully prepared by bioreactor-based cells-loaded scaffold culture method. Finally, Beagle articular cartilage defect model was constructed and the in vivo cartilage defect regeneration ability of allogenic cells-loaded β-TCP bioceramic scaffold was explored and they showed good regeneration ability. The relative cartilage regeneration abilities on Beagle femoral trochleae were as follows: Chondrocyte/osteoblast-loaded β-TCP bioceramic scaffold group > Chondrocyte-loaded β-TCP bioceramic scaffold group > β-TCP bioceramic scaffold. Moreover, compared with previous results using 2D monolayer chondrocyte culture [Citation25,Citation26], this work showed no obvious formation of hypertrophic chondrocytes. Therefore, chondrocyte/osteoblast-loaded β-TCP bioceramic scaffolds using 3D micromass culture are better choice for articular cartilage defect treatment. Chondrocyte/osteoblast-loaded β-TCP bioceramic scaffolds had several advantages for the treatment of cartilage defects: (i) Good cartilage regeneration ability; (ii) wide allogenic BMSCs availability; (iii) no need additional surgery for patients; (iv) no donor site defect formation in patients; (v) less possible blood loss and infection. This work suggested micromass stem cell culture and bioreactor-based cells-loaded scaffold culture can be applied to prepare chondrocyte/osteoblast-loaded β-TCP bioceramic scaffold. The allogenic chondrocyte/osteoblast-loaded β-TCP bioceramic scaffold could be potentially used to treat cartilage defects and could benefit patients with cartilage repair need. It should be noted further work is needed to analyze the underlying mechanisms prior to clinical application.
Disclosure statement
No potential conflict of interest was reported by the authors.
Correction Statement
This article was originally published with errors, which have now been corrected in the online version. Please see Correction (http://dx.doi.org/10.1080/21691401.2023.2258319)
Additional information
Funding
References
- Buckwalter JA, Mow VC, Ratcliffe A. Restoration of injured or degenerated articular cartilage. J Am Acad Orthop Surg. 1994;2:192–201.
- Curl WW, Krome J, Gordon ES, et al. Cartilage injuries: a review of 31,516 knee arthroscopies. Arthroscopy 1997;13:456–460.
- Browne JE, Branch TP. Surgical alternatives for treatment of articular cartilage lesions. JAAOS - J Am Acad Orthop Surg. 2000;8:180–189.
- Sgaglione NA, Miniaci A, Gillogly SD, et al. Update on advanced surgical techniques in the treatment of traumatic focal articular cartilage lesions in the knee. Arthr J Arthr Rel Surg. 2002;18:9–32.
- Brittberg M, Nilsson A, Lindahl A, et al. Rabbit articular cartilage defects treated with autologous cultured chondrocytes. Clin Orthop Rel Res. 1996;326:270–283.
- Dahlin RL, Kinard LA, Lam J, et al. Articular chondrocytes and mesenchymal stem cells seeded on biodegradable scaffolds for the repair of cartilage in a rat osteochondral defect model. Biomaterials 2014;35:7460–7469.
- Makris EA, Gomoll AH, Malizos KN, et al. Repair and tissue engineering techniques for articular cartilage. Nat Rev Rheumatol. 2015;11:21.
- Wei D, Qiao R, Dao J, et al. Soybean lecithin-mediated nanoporous PLGA microspheres with highly entrapped and controlled released BMP-2 as a stem cell platform. Small 2018;14:1800063.
- Zhu Y, Chen Y, Xu G, et al. Micropattern of nano-hydroxyapatite/silk fibroin composite onto Ti alloy surface via template-assisted electrostatic spray deposition. Mater Sci Eng C. 2012;32:390–394.
- Xu N, Ye X, Wei D, et al. 3D artificial bones for bone repair prepared by computed tomography-guided fused deposition modeling for bone repair. ACS Appl Mater Interf. 2014;6:14952–14963.
- Bai W, Kuang T, Chitrakar C, et al. Patchable micro/nanodevices interacting with skin. Biosens Bioelectron. 2018;122:189–204.
- Swieszkowski W, Tuan BHS, Kurzydlowski KJ, et al. Repair and regeneration of osteochondral defects in the articular joints. Biomol Eng. 2007;24:489–495.
- Guo X, Wang C, Duan C, et al. Repair of osteochondral defects with autologous chondrocytes seeded onto bioceramic scaffold in sheep. Tissue Eng. 2004;10:1830–1840.
- Guo X, Wang C, Zhang Y, et al. Repair of large articular cartilage defects with implants of autologous mesenchymal stem cells seeded into β-tricalcium phosphate in a sheep model. Tissue Eng. 2004;10:1818–1829.
- Suo H, Wang Z, Dai G, et al. Polyacrylonitrile nerve conduits with inner longitudinal grooved textures to enhance neuron directional outgrowth. J Microelectromech Syst. 2018;27:457–463.
- Hao Z, Song Z, Huang J, et al. The scaffold microenvironment for stem cell based bone tissue engineering. Biomater Sci. 2017;5:1382–1392.
- Sun G, Wei D, Liu X, et al. Novel biodegradable electrospun nanofibrous P(DLLA-CL) balloons for the treatment of vertebral compression fractures. Nanomedicine: NBM. 2013;9:829–838.
- Liu X, Wei D, Zhong J, et al. Electrospun nanofibrous P(DLLA–CL) balloons as calcium phosphate cement filled containers for bone repair: in vitro and in vivo studies. ACS Appl Mater Interf. 2015;7:18540–18552.
- Murdoch AD, Grady LM, Ablett MP, et al. Chondrogenic differentiation of human bone marrow stem cells in transwell cultures: generation of scaffold-free cartilage. Stem Cells 2007;25:2786–2796.
- Li W-J, Tuli R, Okafor C, et al. A three-dimensional nanofibrous scaffold for cartilage tissue engineering using human mesenchymal stem cells. Biomaterials 2005;26:599–609.
- Wang Y, Kim U-J, Blasioli DJ, et al. In vitro cartilage tissue engineering with 3D porous aqueous-derived silk scaffolds and mesenchymal stem cells. Biomaterials 2005;26:7082–7094.
- Martin I, Wendt D, Heberer M. The role of bioreactors in tissue engineering. Trends Biotechnol. 2004;22:80–86.
- Pazzano D, Mercier KA, Moran JM, et al. Comparison of chondrogensis in static and perfused bioreactor culture. Biotechnol Prog. 2000;16:893–896.
- Sun S, Ren Q, Wang D, et al. Repairing cartilage defects using chondrocyte and osteoblast composites developed using a bioreactor. Chinese Med J. 2011;124:758–763.
- Caron MMJ, Emans PJ, Coolsen MME, et al. Redifferentiation of dedifferentiated human articular chondrocytes: comparison of 2D and 3D cultures. Osteoarthr Cart. 2012;20:1170–1178.
- Schnabel M, Marlovits S, Eckhoff G, et al. Dedifferentiation-associated changes in morphology and gene expression in primary human articular chondrocytes in cell culture. Osteoarthr Cart. 2002;10:62–70.
- Ronzière M-C, Roche S, Gouttenoire J, et al. Ascorbate modulation of bovine chondrocyte growth, matrix protein gene expression and synthesis in three-dimensional collagen sponges. Biomaterials 2003;24:851–861.
- Megat Abdul Wahab R, Mohamed Rozali NA, Senafi S, et al. Impact of isolation method on doubling time and the quality of chondrocyte and osteoblast differentiated from murine dental pulp stem cells. Peer J. 2017;5:e3180.
- Koç ON, Gerson SL, Cooper BW, et al. Rapid hematopoietic recovery after coinfusion of autologous-blood stem cells and culture-expanded marrow mesenchymal stem cells in advanced breast cancer patients receiving high-dose chemotherapy. JCO. 2000;18:307–316.
- Roche S, Ronzière MC, Herbage D, et al. Native and DPPA cross-linked collagen sponges seeded with fetal bovine epiphyseal chondrocytes used for cartilage tissue engineering. Biomaterials 2000;22:9–18.
- Ma Z, Piao T, Wang Y, et al. Astragalin inhibits IL-1β-induced inflammatory mediators production in human osteoarthritis chondrocyte by inhibiting NF-κB and MAPK activation. Int Immunopharm. 2015;25:83–87.
- Lin K, Chang J, Lu J, et al. Properties of β-Ca3(PO4)2 bioceramics prepared using nano-size powders. Ceram Int. 2007;33:979–985.