Abstract
Poly(lactic-co-glycolic acid) (PLGA) based biomaterials have many advantages and potential applications in bone tissue engineering. Whereas, a significant problem which could not be ignored was that the acidic by-products generated during its degradation induced severe inflammatory reactions and negatively regulated bone regeneration. In this research, feasibility of using dexamethasone (Dex) to improve the biocompatibility of PLGA is investigated in detail. Hereby, various contents of PLGA/hydroxyapatite (PH) nanofibers loaded with Dex were synthesized by electrospinning technique. It was shown that 0.5% (wt) Dex in PH scaffolds was the minimum content which was required for anti-inflammatory effect. Admittedly, Dex to some extent had cytotoxic effect on osteoblasts and an inhibitory effect on ALP activity in this study; nevertheless, the relatively low Dex (<2% [wt]) had no inhibitory effects on osteoblasts maturation and mineralization. By this token, Dex is a good candidate for improving biocompatibility of PLGA based biomaterials. Moreover, the cytotoxic effects of Dex should be concerned. This study will provide a rationale for optimizing biocompatibility of PLGA based biomaterials by using Dex.
Introduction
The treatment of large bone defects is still a major clinical challenge. Recently, bone tissue engineering has provided a potential strategy in curing of large bone defects. Innovative scaffolds, stem cells, and biological molecules are three significant elements in bone tissue engineering [Citation1]. However, stem cell-based strategy has many restrictions including the limited source of autologous stem cells, the potential immune response of the allogeneic stem cells, complex cultural expansion procedures and difficulty of stemness retention, which limited its clinical transformation. On the contrary, innovative scaffolds and biological molecules based strategy could construct a good microenvironment to activate the self-healing potential and provide a simple way to use in the clinic.
The criterion for designing a scaffold is following the nature of the target tissue. Hence, bone tissue engineering scaffolds should be designed as an extracellular matrix (ECM) like structure. A variety of methods have been developed to fabricate ECM like scaffolds, such as self-assembly, phase-separation, and electrospinning [Citation2]. Among these techniques, electrospinning has gained considerable interest due to its easy way of preparation, high yield and low cost [Citation3,Citation4]. Electrospun nanofibers have numerous attractive features such as large surface area, interconnected pores, and potential applications for controlling drug release [Citation5]. A wide range of materials can be used for preparing electrospun nanofibers, for instance, chitosan, gelatin, poly-caprolactone (PCL), poly-lactic acid (PLA) and PLGA [Citation6]. Among these materials, PLGA has been approved for clinical use on human beings by the U.S. Food and Drug Administration (FDA), due to its good biocompatibility, tailored biodegradation rate and good drug loading performance [Citation7]. Many research groups have developed PLGA-based electrospun nanofibrous scaffolds as bone tissue engineering scaffolds [Citation8–10]. Among these studies, nano-hydroxyapatite has been widely used to improve the osteogenic activity of PLGA nanofibers [Citation11,Citation12]. Whereas, the authors’ previous study had proved that nano-hydroxyapatite modified PLGA electrospun nanofibers possessed limited osteogenic activity in vitro and in vivo. Moreover, acidic byproducts of this PLGA induced serious local inflammation in the PH group in vivo, which may do harm to tissue repair [Citation13]. Thus, for adapting its clinical application, it is critical to figure out a way for improving osteogenic activity and biocompatibility of PLGA based nanofibers.
Biological molecules including growth factors and osteogenic small molecules are another important component in bone tissue engineering for improving osteogenic activity and biocompatibility. Nevertheless, high costs, instability, immunogenic properties and structural integrity requirements of growth factors will reduce their possibility of potential clinical applications. In contrast, osteogenic small molecules possess many advantages compared to growth factors, including physicochemically well-defined structure, minimized costs, low risk of contamination, stability and the non-immunogenic characteristic [Citation14]. Hereby, small molecules such as simvastatin [Citation13], vitamin D [Citation15], ascorbic acid (AA)[Citation16] and amethasone[Citation17] are good options for improving the osteogenic activity and biocompatibility of PLGA nanofibers. Among them, dexamethasone (Dex) has already been widely used to upregulate osteogenic activity and biocompatibility of biomaterials [Citation18–20]. Dex has been shown to stimulate differentiation of MSCs towards osteogenic lineage in vitro, together with ascorbic acid and β-glycerophosphate, in defined concentrations [Citation21]. Importantly, Dex is also a kind of potent steroidal anti-immune and inflammatory response-suppressing drug, and could inhibit inflammatory response induced by PLGA byproducts [Citation22]. Hence, Dexamethasone is very suitable for improving the osteogenic activity and biocompatibility of PLGA based nanofibers. However, numerous studies have demonstrated that bone mass is subnormal in patients treated with steroids for asthma and rheumatoid arthritis [Citation23,Citation24]. Osteoporosis in patients treated with supraphysiologic doses of glucocorticoids is greatly associated with the direct inhibition of osteoblastic proliferation and function. By this token, screening the best drug loading contents in PH nanofibers is crucial for avoiding the side effects caused by Dex.
In this study, the optimum condition to fabricate PLGA/HA electrospun nanofibrous have been focused. What’s more, gradient content of Dex loaded electrospun PH nanofibrous scaffolds were fabricated by electrospinning, and the optimum drug loading for modulating its biocompatibility was evaluated in-depth both in vitro and in vivo. The results indicated that 0.5% (wt) Dex in PH scaffolds was the minimum content which was required for anti-inflammatory effect. Low content of Dex in PH had no inhibitory effects on osteoblasts maturation and mineralization. This study will provide a good rationale for the optimization and application of PH based electrospun nanofibrous scaffolds in bone regeneration.
Materials and Methods
Materials PLGA/HA nanofibrous scaffolds synthesis
PLGA (the ratio of lactic to glycolic acid = 85:15, molecule weight 70,000 Da) was purchased from Sigma (St. Louis, MO, USA), and used to fabricate biodegradable nanofibrous scaffolds by electrospinning. Hydroxyapatite (HA) nano-particles (diameter <200 nm, Sigma, USA) and Dexamethasone were also purchased from Sigma, the same as acetonitrile, methanol-chloroform and dimethylformamide (DMF, Sigma, USA).
Effect of solute concentration and solvent ratio on morphology of electrospun nanofibers
To begin with, solute concentration and solvent ratio on the morphology of electrospun nanofibers were assessed. Firstly, 10, 5 and 20% (w/v) electrospun solutions were prepared by dissolved PH (with HA 10% [wt]) in chloroform, followed by magnetic stirring for 12 h. Then, electrospun solutions with a fixed solute concentration (15% PH, w/v) were prepared in chloroform and DMF blended solvent at different ratio (V:V = 9:1, 7:3 and 5:5) followed by magnetic stirring for 12 h. All mixtures were loaded into a 10 ml syringe with a 23-gauge needle and electrospun to fabricate nanofibrous scaffolds under high voltage (18 kV, working distance 18 cm), with a consistent feed rate (1.0 ml/h), using electrospray/spinning method. Generated fibers were collected on an 18 mm diameter coverslip and the morphology was evaluated by upright optical microscope (Olympus BX51, Japan).
Preparation and characterization of Dex loaded nanofibrous scaffolds
Based on the effects of solute concentration and solvent ratio on the morphology of electrospun nanofibers, 15% solute concentration and blended chloroform and DMF solvent with a ratio of 7:3 was identified to prepare Dex loaded PH nanofibrous scaffolds (Please refer to “Results and discussion” section). Thus, PH (with HA 10% [wt]) mixtures incorporated with different quantity of Dex (5, 2, 1, 0.5 and 0.25% [wt] PH) were added into chloroform and DMF blended solution (V:V = 7:3) to prepare the various electrospun solutions with 15% solute concentration (w/v). After magnetic stirring for 12 h, all mixtures were loaded into a 10 ml syringe with a 23-gauge needle to fabricate nanofibrous scaffolds under the above parameters. Generated fibers were collected on an 18 mm diameter coverslip and stored in vacuum desiccators before use. The surface morphology of Dex-loaded electrospun PH nanofibers was analyzed by scanning electron microscopy (SEM; JEOL FESEM 6700F, Tokyo, Japan). Specimens were sputter-coated with a thin layer of platinum to decrease the surface charging before observation and micrographs were recorded at 3 kV. The diameter distribution of the prepared fibers was calculated according to SEM analysis by ImageJ software (version 1.51r, NIH, USA).
Drug release profile analysis
PH nanofibrous scaffold containing 5% (wt) Dex was chosen as a candidate to evaluate the drug release profile. 50 mg Dex loaded PH nanofibrous scaffold were weighed and incubated at 37 °C in 10 ml of phosphate buffer solution and stirred at 100 rpm (n = 3). Aliquots of 2 ml were retrieved in predetermined time intervals and stored at −20 °C for subsequent use. Meanwhile, an equal volume of fresh media was added to the suspension. After collecting all the samples, the release behaviour of Dex were analyzed by High Performance Liquid Chromatography (HPLC) (Waters, USA, 0.01–20.00ML/MI/P600) consisting of a model 2489 UV detector, a Prostar e2695 solvent delivery system with an inline filter and degasser, and a Dynamax HPLC Method Manager analytical software. All solvents were of HPLC grade. Dex, the external standard used was obtained by Macklin (Shanghai Macklin Biochemical Corporation, China) and dissolved in HPLC grade methanol to make standard solutions. All samples were filtered by 0.45 μm filtrator (Millipore, USA) before detection. The analysis was performed at 242 nm using a Water’s C-18 column (4.6 mm × 150 mm, 5 μm) with a mobile phase of 50% methanol and 50% acetonitrile flowing at 1.2 ml/min, the injection volume each time was 20 μl. In the process, the retention time of Dex is 1.37 min. The Dex concentration of each sample was calculated from a standard curve (concentrations ranging from 0.015625 to 0.25 mg/ml). Replacement of Aliquots with the fresh medium was taken into account when calculating the amount of drug released. Unloaded control PH nanofibrous scaffolds were considered as blanks in the performed quantification. The results presented in this research are an average of three measurements.
Cell culture
The MC3T3-E1 sub colon 14 cell line originated from a C57BL/6 mouse. It is a useful in vitro model system to study the process of osteoblast differentiation because of similar to primary calvarial osteoblasts [Citation25]. They were cultured in high glucose Dulbecco’s Modified Eagle Medium (H-DMEM, Gibco, Grand Island, NY, USA) containing 10% foetal bovine serum (FBS, Gibco) and 1% penicillin–streptomycin (Gibco) in 5% CO2 at 37 °C. The medium was changed every 3 d. Besides, rat bone marrow stromal cells (BMSCs) were extracted from Sprague-Dawly (SD) rat (80 ∼ 100 g body weight). Total bone marrow was pumped out by a 20 ml syringe and placed in a cell culture dish (Corning, USA), and were cultured in low glucose DMEM (L-DMEM, Gibco) containing 10% FBS and 1% penicillin–streptomycin. These cells were cultured under standard cell culture conditions, and the medium was changed 3 d afterwards. Passage 3 isolated BMSCs were used for all experiments in this study.
Cell proliferation assay
Electrospun PH nanofibrous scaffolds with different quantity (5, 2, 1, 0.5, 0.25 and 0% [wt] polymer) of Dexamethasone was prepared to match the inside diameter of 24 well cell culture plate. Then each side of the scaffolds was irradiated with UV light for 1 h and placed into the culture plate. Besides, sterilized 12% polystyrene (w/v) in tetrahydrofuran solution was used to stick the scaffold membrane to the culture plate. MC3T3-E1 cells and passage 3 isolated BMSCs were seeded on the scaffolds at a density of 5 × 104 cells/well. Cell viability for each predetermined culturing time was investigated using the CellTiter 96 AQueous One Solution Cell Proliferation Assay (Promega, USA). This assay is based on the bioreduction of a tetrazolium compound, 3-(4,5-dimethylthiazol-2-yl)-5-(3-carboxymethoxyphenyl)-2-(4-sulfofenyl)-2H-tetrazolium [MTS], into a water-soluble brown formazan product. Fresh medium was used to replace primary medium on days 1, 3, 5 and 7 after cell seeding and 80 μl of MTS solution was added to each well, followed by incubation at 37 °C in the cell incubator for 3 h. After incubation, the samples were pipetted out into 96-well plates. The absorbance was determined at 490 nm using a microplate reader (RT-6000, Lei Du Life Science and Technology Co, Shenzhen, China). The absorbance was directly proportional to the number of living cells in the scaffolds. Three samples of each nanofiber mesh per time point were characterized.
The cytotoxic effects of pure Dex on MC3T3-E1 cells were also investigated by 3-(4,5-dimethyl-2-thiazolyl)-2,5-diphenyl-2-H-tetrazolium bromide (MTT) assay. A serial concentration of Dex (0, 5, 10, 25, 50, 100, 200, 300, 400 and 500 μM) in the medium was used to stimulate MC3T3-E1 cells. The cells also were seeded on the scaffolds at a density of 5 × 104 cells/well in 96-well plates.
Differentiation of MC3T3-E1 cells on scaffolds
The method for preparation of the scaffolds was the same as mentioned before. MC3T3-E1 cells were seeded at a density of 5 × 104 cells/well on scaffolds in 24-well cell culture plates. The cells were cultured in osteogenic conditioned medium of H-DMEM containing 10% FBS, 10 mM sodium β-glycerol phosphate (Sigma, USA) and L-ascorbic acid (50 mg/ml) (Sigma, USA), and the medium was changed every 3 d.
Osteogenic differentiation was evaluated by alkaline phosphatase (ALP) staining and calcium deposition. After 14 days of cell seeding, for detection of samples, alkaline phosphatase (ALP) staining (Leukocyte Alkaline Phosphatase Assay Kit, Sigma) was used. Graphs were recorded using a scanner (Epson, Perfection V800, Japan), and micrographs were observed by an upright optical microscope (Olympus BX51, Japan).
Alizarin red staining is used to determine the presence of calcific deposition on the extracellular matrix by osteoblasts. After 21 days’ culture, the scaffolds were briefly rinsed with PBS and fixed in 90% ice-cold ethanol for 10 min. Then, the samples were washed thrice with distilled water. After that, they were stained with ARS (0.1%) (Sigma, USA) for 30 min and incubated at 37 °C. Graphs were recorded using a scanner after washing several times with distilled water.
Subcutaneous implantation of scaffolds in vivo
SD rats (200 ∼ 250 g body weight) were used to make the subcutaneously implanted model, and the procedures were approved by Jilin University Animal Care and Use Committee. The experimental procedures could be described briefly as follows: rats were anaesthetized with 10% chloral hydrate by intraperitoneal injection (dissolved in normal saline, 3.5 ml/kg body weight). The surgical sites were cleaned and disinfected using 70% ethanol and iodine scrub. Seven paravertebral incisions (1.5 cm each) per rat, ∼1 cm lateral to the vertebral column, were made to expose the dorsal subcutis, and the longitudinal distance between two incisions is 2 cm. Blunt dissection was used to isolate subcutis pockets that adjacent to the back muscles. 0.42 mg sterilized various scaffolds with a rectangular shape (∼3 mm × 12 mm) were rolled up along the long edge with a sterile steel wire (diameter = 1 mm) and then, respectively implanted into the subcutaneous pocket. Besides, the sham operation was made for paired observation. After insertion of an implant, the incisions were then sutured with 3–0 at submucous layer and skin respectively. After the surgery, all rats were raised in the same environment and received an antibiotic for 3 d.
On day 28 after the operation, three rats were anaesthetized and euthanized. The tissue-covered specimens were collected and fixed in 4% paraformaldehyde, dehydrated in a graded series of ethanol. The specimens were incised perpendicular to the major axis and embedded in paraffin. Slices (5 μm cross-section) of each sample were prepared for hematoxylin and eosin (H&E). Histological evaluation was performed by two independent examiners according to a histological grading scale that had been used in our previous study and other research groups as showed in [Citation12,Citation26]. Two mutually perpendicular lines, passing through the center of circle-like shaped scaffold-remnants, intersecting with the fibrous capsule surrounded the remnants were determined as the four representative areas for qualitative and quantitative examination of the fibrous capsule quality.
Table 1. Histological grading scale for inflammatory immune response.
Statistical analysis
Results are expressed as mean ± standard deviation (SD). A one-way ANOVA was used to test for statistical significance. Differences were considered statistically significant at p < .05, p < .01, p < .001 or p < .0001 level. All in vitro experiments were repeated three times.
Results and discussion
Effect of solute concentration and solvent ratio on the morphology of electrospun nanofibers
In order to obtain nanofibers with good morphology and nice structure, we first analyzed the optimum solute concentration and solvent ratio for electrospinning Dex loaded PH scaffolds. Thus, 10, 15 and 20% (w/v) of PLGA (with HA 10% [wt]) were dissolved in chloroform to explore the optimal PH concentration. The results showed that the nanofibrous structure appeared and increased with the increase of concentration PH concentration. 5% PH group had no nanofibrous structure. In contrast, 15 and 20% PH group had some nanofibers generated, while the latter group generated more (). But all of these are not ideal, and solute concentration needs further investigation to identify the optimum concentration. Studies had demonstrated that the density of beads in electrospun nanofibers decreased if more viscous solutions (i.e. higher concentrations) were used [Citation27,Citation28]. Thus, more fibers were observed in a high concentration of PH group in our experiments.
Figure 1. Micrographs of PLGA nanofibrous scaffolds. (A) 10% PH group, (B) 15% PH group, (C) 20% PH group, (D) 15% PH dissolved in chloroform and DMF (V:V = 5:5), (E) 15% PH dissolved in chloroform and DMF (V:V = 7:3), (F) 15% PH dissolved in chloroform and DMF (V:V = 9:1). Black circles indicate generated fibers, blue circles indicate generated beads.
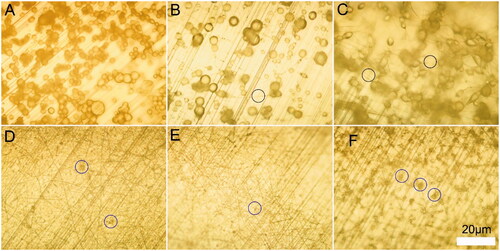
With the aim of obtaining good morphology of nanofibers at relatively low solute concentration, we changed the solvent composition. Various ratios of chloroform and DMF blended solvents were used to prepare 15% (w/v) PH electrospun solutions. Data showed that robust unwanted droplets formed when chloroform to DMF ratio at 9:1 () and pure chloroform (). However, a fine nanofibrous structure formed when chloroform to DMF ratio was at 7:3 and 5:5, while there were still some beads formed and with more in the latter group (). Rayleigh had demonstrated that surface tension tends to convert the liquid jet into droplets(beads) [Citation29]. As is known, the surface tension of chloroform (28.9 dyne/cm) is higher than DMF(25.7 dyne/cm). Thus, blending DMF in chloroform will reduce the surface tension and will be favourable for generating fibers structure. Whereas, there were more beads formed in a group of chloroform to DMF ratio at 5:3 than 7:3. Hence, there should be other intrinsic factors of solvents that affect the formation of fibers and need further investigation. Collectively, our results indicated that fine nanofibrous structure can be obtained while chloroform to DMF ratio is at 7:3, with a relative low concentration of PH (15%, w/v), and were identified for preparing Dex loaded nanofibers subsequently.
Characterization of Dex loaded nanofibrous scaffolds
The surface morphology of Dex-loaded electrospun PH nanofibers was analyzed by scanning electron microscopy (SEM). The results showed that homogenous and smooth nanofibres were fabricated by electrospinning with or without Dex loaded (). The nanofibrous scaffolds were polyporous similar to ECM structure. There were some aggregations of nano-hydroxyapatite nanoparticles on the surface of nanofibers. Besides, micrographs showed that incorporating with Dex had a significant influence on the morphology and diameter of fibers among these scaffolds (). The fiber diameter decreased with the addition of Dex. One possible reason is that Dex increased the charge density of the jet which enhanced the stretching force during electrospinning [Citation28]. Low fiber diameter is always associated with high porosity in scaffolds, which is beneficial to nutrients and metabolic waste exchange.
Figure 2. Characterization of Dex loaded PH nanofibrous scaffolds. (A) PH group, (B) 5% Dex loaded PH group, (C) 2% Dex loaded PH group, (D) 1% Dex loaded PH group, (E) 0.5% Dex loaded PH group, (F) 0.25% Dex loaded PH group. (G) Fiber diameter distribution of various scaffolds. Blue arrows indicate nano-hydroxyapatite aggregation.
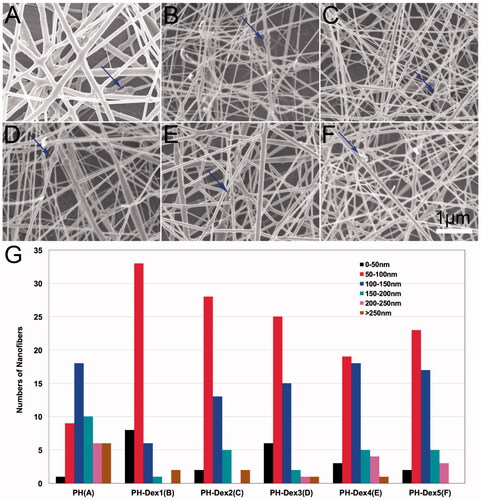
Controlled release of Dex from PH scaffold
Release behaviours of Dex on drug-loaded PLGA nanofibers were studied and shown in . The results demonstrated an initial burst release of Dex of 30.5% on the first day followed by a slow and sustained release of 43.2% during the following 55 d. Ultimately, 73.7% of Dex was released from the nanofibers in 49 d. The initial burst of drug release is related to polymer hydrophobicity as well as drug type. Drug on the surface, in contact with the medium in vitro or extracellular fluids in vivo, is released by directly dissolving and diffusing in them. Thus, the initial burst of drug release in vitro was similar to in vivo. Thereafter, a sustained drug release was observed mainly due to the degradation of the scaffold by hydrolysis of the polymer in vitro and this process may be also mediated by an enzymatic degradation in vivo [Citation30,Citation31]. Thus, the releases of Dex will have a difference in the later stage in vitro compared with in vivo. Characteristics of Dex release in vitro will give some guidance for the application of the scaffolds in vivo.
Effects of various Dex and Dex loaded PH scaffold on osteoblastic cell proliferation
In this study, MC3T3-E1 cells were seeded on the scaffolds and cell viability was investigated using MTS assay. The result showed () that pure PH nanofibrous scaffold and Dex loaded PH scaffolds exhibited inhibitory effects on osteoblastic cell proliferation compared with the control group. Moreover, the suppressive effects of Dex loaded PH scaffolds were more potent than pure PH scaffold. As time extended, the viability of MC3T3-E1 cells co-cultured with pure PH scaffolds increased observably, while Dex loaded PH groups had no change. The inhibitory effects of PH scaffolds on cell proliferation may be attributed to the hydrophobicity of the scaffolds that hindered cell adhesion quickly. Dex is a widely used glucocorticoid for the treatment of inflammatory diseases, pulmonary and autoimmune disorder [Citation32]. However, excessive Dex application has substantial adverse effects, such as osteoporosis and osteonecrosis, and may lead to bone damage [Citation33,Citation34]. It has been documented that Dex would induce apoptosis of osteoblasts through the induction of oxidative DNA damage and the generation of reactive oxygen species (ROS) [Citation35]. Our results () confirmed the cytotoxic effects of Dex in 3 d and 7 d to osteoblasts compared with the control group and also reminded the importance of screening the optimum drug loading. Due to the anti-inflammatory effects of Dex, there was a certain positive effect on cell proliferation in 1 d (). Besides, it can be observed that 500 μM is a critical point, and the concentration above 500 μM was obviously toxic.
Figure 4. MC3T3-E1 cells proliferation on various scaffolds. Cont.: control group; PH: PLGA/HA group; PH-Dex1: 5% Dex loaded PH group; PH-Dex2: 2% Dex loaded PH group; PH-Dex3: 1% Dex loaded PH A group; PH-Dex4: 0.5% Dex loaded PH group; PH-Dex5: 0.25% Dex loaded PH group. ****p < .0001 vs all the other groups.
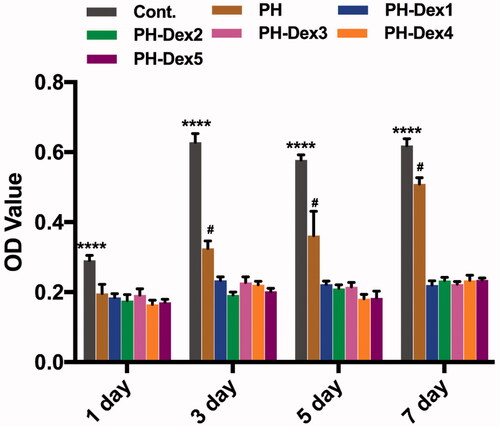
Effects of various Dex loaded PH scaffold on osteoblastic cell function
Alkaline phosphatase (ALP) staining and alizarin red staining(ARS) were used to evaluate osteoblasts function on Dex loaded scaffolds. Alkaline phosphatase (ALP) staining showed that ALP-positive cells decreased markedly when cells were cultured on scaffolds with Dex incorporation compared to the pure PH scaffold (). Besides, the ALP activity of cells cultured on PH scaffold was much higher than the control group. However, the ALP activity among the various Dex loaded scaffolds could not be distinguished based on ALP assay, and thus needs in-depth investigation. Herein, the results demonstrated that Dex could inhibit ALP activity in MC3T3-E1 cells. Nevertheless, the effects of Dex on osteoblasts differentiation is controversial. Jane B. Liano and co-workers indicated that exposure of MC3T3-E1 cells to Dex, initiated during the proliferation period, blocked osteoblast differentiation, had a negative effect on ALP activity, which is consistent with our results [Citation36]. In contrast, Hong and co-workers demonstrated that Dex could enhance the differentiation of osteoblastic cells through upregulation of PDZ-binding motif (TAZ) expression in rat mesenchymal stem cells, and had a positive effect on ALP expression [Citation37]. Hence, the detailed underlying mechanisms still need further clarification.
Figure 6. ALP staining of MC3T3-E1 cells on day 21. (A) Control group on tissue culture plate, (B) PH group, (C) 5% Dex loaded PH group, (D) 2% Dex loaded PH group, (E) 1% Dex loaded PH group, (F) 0.5% Dex loaded PH group, (G) 0.25% Dex loaded PH group.
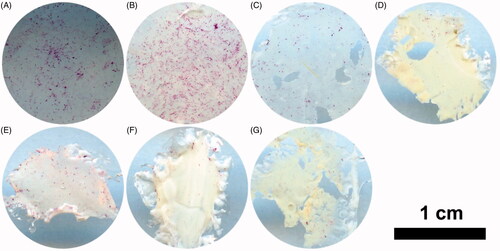
Alizarin red staining (ARS) was performed to evaluate the calcium deposition of the extracellular matrix produced by MC3T3-E1 cells. The results showed that the positive staining increased obviously on the pure PH scaffolds or Dex-loaded PH scaffolds compared with the control group (). Moreover, the amount of the calcium deposition on the 5% Dex-loaded PH scaffolds decreased significantly compared with the PH group, while the other Dex loaded PH groups had no obvious differences. This implied that a high dosage of Dex had negative effects on osteoblasts differentiation and maturation. Although Dex had an inhibitory effect on ALP activity, as shown above, a relatively low content of Dex in PH scaffolds did not inhibit osteoblasts maturation and forming of calcium nodules.
Biocompatibility of the scaffolds in vivo
The biocompatibility of a scaffold is a prerequisite for application in vivo. Any undesirable local or systemic responses are impermissible. The inflammatory responses to pure PH and Dex loaded scaffolds were analyzed after a 4-week subcutaneous implantation period in SD rat. Representative H&E staining sections of the scaffolds are shown in . Histological images show that fibrous capsules generated the various scaffolds in the surrounding. The results indicated that granulous and dense, containing both fibroblasts and many inflammatory cells in fibrous capsules of PH and PH-Dex5 groups, while the collagen fibrous capsules assembled well and no inflammatory cells were found in the other groups. Besides, as the content of Dex increased, the thickness of the fibrous capsule showed a decreasing tendency. Critically, multilayer multinuclear cells were found in PH and PH-Dex5 groups, especially for the former group, which would do harm to tissue regeneration. In contrast, there were no multinuclear cells in control and other Dex loaded PH scaffold groups. Histological grading results demonstrated that there was a significant reduction of inflammatory cells infiltration with the incorporation of Dex (). Collectively, our results found that 0.5% (wt) Dex in PH scaffolds was the minimal content that is needed for effectively repressing inflammatory cells infiltration. The reason is that using a high dosage of Dex will induce severe side effects as described above, identify optimal Dex containing PH scaffolds is significant for the future application of PH based electrospun nanofibrous scaffolds. These results suggested that Dex was a preferable drug for modifying the function of PH nanofibers scaffold and these composites could be excellent scaffolds for bone tissue engineering.
Figure 8. Histological response and grading of two scaffolds after 4-week subcutaneous implantation. (A,B) Cont. group, (C,D) PH group, (E,F) PH-Dex1 group, (G,H) PH-Dex2 group, (I,J) PH-Dex3 group, (K,L) PH-Dex4 group, (M,N) PH-Dex5 group. The right column of images (B,D,F,H,J,L,N) are the enlarged images of green rectangle box in the left column, of images (A,C,E,G,I,K,M). Green arrow: infiltrated macrophage. Black arrow: indicating fibrous capsule wall. FC: Fibrous capsule wall. S: scaffold remnants. Blue arrow: indicating area for evaluating histological response and grading.
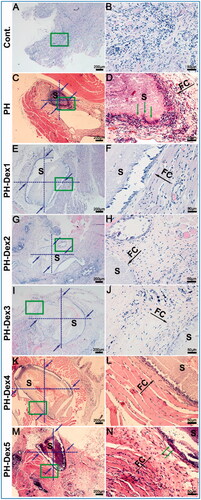
Table 2. Score of histological grading scale for inflammatory response of scaffolds.
Conclusions
It is demonstrated in this study that the incorporation of Dex into PH electrospun nanofibers could effectively reduce inflammatory response induced by acidic byproducts of PH scaffold. Importantly, it was found that 0.5% (wt) Dex in PH scaffolds was the minimal content that is needed for effectively repressing inflammatory cells infiltration. Although Dex has a certain cytotoxic effect on osteoblasts and an inhibitory effect on ALP activity in this study, the relatively low Dex (<2% [wt]) has no inhibition of osteoblasts maturation and mineralization. This study hopefully would provide guidance for the application of Dex in modifying PLGA based electrospun nanofibers.
Disclosure statement
No potential conflict of interest was reported by the authors.
Additional information
Funding
References
- Black CR, Goriainov V, Gibbs D, et al. Bone tissue engineering. Curr Mol Biol Rep. 2015;1(3):132–140.
- Rose JC, De Laporte L. Hierarchical design of tissue regenerative constructs. Adv Healthc Mater. 2018;7(6):e1701067.
- Liu W, Thomopoulos S, Xia Y. Electrospun nanofibers for regenerative medicine. Adv Healthc Mater. 2012;1(1):10–25.
- Bhardwaj N, Kundu SC. Electrospinning: a fascinating fiber fabrication technique. Biotechnol Adv. 2010;28(3):325–347.
- Su Y, Li X, Wang H, et al. Fabrication and characterization of biodegradable nanofibrous mats by mix and coaxial electrospinning. J Mater Sci Mater Med. 2009;20(11):2285–2294.
- Lu CH, Chang YH, Lin SY, et al. Recent progresses in gene delivery-based bone tissue engineering. Biotechnol Adv. 2013;31(8):1695–1706.
- Gentile P, Chiono V, Carmagnola I, et al. An overview of poly(lactic-co-glycolic) acid (PLGA)-based biomaterials for bone tissue engineering. Int J Mol Sci. 2014;15(3):3640–3659.
- Ngiam M, Liao S, Patil AJ, et al. The fabrication of nano-hydroxyapatite on PLGA and PLGA/collagen nanofibrous composite scaffolds and their effects in osteoblastic behaviour for bone tissue engineering. Bone. 2009;45(1):4–16.
- Fu C, Bai H, Zhu J, et al. Enhanced cell proliferation and osteogenic differentiation in electrospun PLGA/hydroxyapatite nanofibre scaffolds incorporated with graphene oxide. PLoS One. 2017;12(11):e0188352.
- Blakney AK, Simonovsky FI, Suydam IT, et al. Rapidly biodegrading PLGA-polyurethane fibers for sustained release of physicochemically diverse drugs. ACS Biomater Sci Eng. 2016;2(9):1595–1607.
- Lao L, Wang Y, Zhu Y, et al. Poly(lactide-co-glycolide)/hydroxyapatite nanofibrous scaffolds fabricated by electrospinning for bone tissue engineering. J Mater Sci Mater Med. 2011;22(8):1873–1884.
- Li D, Sun H, Jiang L, et al. Enhanced biocompatibility of PLGA nanofibers with gelatin/nano-hydroxyapatite bone biomimetics incorporation. ACS Appl Mater Interfaces. 2014;6(12):9402–9410.
- Jiang L, Sun H, Yuan A, et al. Enhancement of osteoinduction by continual simvastatin release from poly(lactic-co-glycolic acid)-hydroxyapatite-simvastatin nano-fibrous scaffold. J Biomed Nanotechnol. 2013;9(11):1921–1928.
- Balmayor ER. Targeted delivery as key for the success of small osteoinductive molecules. Adv Drug Deliv Rev. 2015;94:13–27.
- Yoon SJ, Park KS, Kim MS, et al. Repair of diaphyseal bone defects with calcitriol-loaded PLGA scaffolds and marrow stromal cells. Tissue Eng. 2007;13(5):1125–1133.
- Zhang J, Doll BA, Beckman EJ, et al. A biodegradable polyurethane-ascorbic acid scaffold for bone tissue engineering. J Biomed Mater Res A. 2003;67(2):389–400.
- Li L, Zhou G, Wang Y, et al. Controlled dual delivery of BMP-2 and dexamethasone by nanoparticle-embedded electrospun nanofibers for the efficient repair of critical-sized rat calvarial defect. Biomaterials. 2015;37:218–229.
- Martins A, Duarte AR, Faria S, et al. Osteogenic induction of hBMSCs by electrospun scaffolds with dexamethasone release functionality. Biomaterials. 2010;31(22):5875–5885.
- Chen W, Li D, Ei-Shanshory A, et al. Dexamethasone loaded core-shell SF/PEO nanofibers via green electrospinning reduced endothelial cells inflammatory damage. Colloids Surf B Biointerfaces. 2015;126:561–568.
- Vacanti NM, Cheng H, Hill PS, et al. Localized delivery of dexamethasone from electrospun fibers reduces the foreign body response. Biomacromolecules. 2012;13(10):3031–3038.
- Jaiswal N, Haynesworth SE, Caplan AI, et al. Osteogenic differentiation of purified, culture-expanded human mesenchymal stem cells in vitro. J Cell Biochem. 1997;64(2):295–312.
- Franz S, Rammelt S, Scharnweber D, et al. Immune responses to implants - a review of the implications for the design of immunomodulatory biomaterials. Biomaterials. 2011;32(28):6692–6709.
- Greenberger PA, Hendrix RW, Patterson R, et al. Bone studies in patients on prolonged systemic corticosteroid therapy for asthma. Clin Allergy. 1982;12(4):363–368.
- Adinoff AD, Hollister JR. Steroid-induced fractures and bone loss in patients with asthma. N Engl J Med. 1983;309(5):265–268.
- Quarles LD, Yohay DA, Lever LW, et al. Distinct proliferative and differentiated stages of murine MC3T3-E1 cells in culture: an in vitro model of osteoblast development. J Bone Miner Res. 1992;7(6):683–692.
- Jansen JA, Dhert WJ, van der Waerden JP, et al. Semi-quantitative and qualitative histologic analysis method for the evaluation of implant biocompatibility. J Invest Surg. 1994;7(2):123–134.
- Deitzel JM, Kleinmeyer JD, Hirvonen JK, et al. Controlled deposition of electrospun poly(ethylene oxide) fibers. Polymer. 2001;42(19):8163–8170.
- Li D, Xia Y. Electrospinning of nanofibers: reinventing the wheel? Adv Mater. 2004;16(14):1151–1170.
- Rayleigh L. XX. On the equilibrium of liquid conducting masses charged with electricity. Proc R Soc London. 1882;14:184.
- Crotts G, Park TG. Protein delivery from poly(lactic-co-glycolic acid) biodegradable microspheres: release kinetics and stability issues. J Microencapsul. 1998;15(6):699–713.
- Makadia HK, Siegel SJ. Poly Lactic-co-Glycolic Acid (PLGA) as Biodegradable Controlled Drug Delivery Carrier. Polymers (Basel). 2011;3(3):1377–1397.
- Schacke H, Docke WD, Asadullah K. Mechanisms involved in the side effects of glucocorticoids. Pharmacol Ther. 2002;96(1):23–43.
- den Uyl D, Bultink IE, Lems WF. Advances in glucocorticoid-induced osteoporosis. Curr Rheumatol Rep. 2011;13(3):233–240.
- Kerachian MA, Seguin C, Harvey EJ. Glucocorticoids in osteonecrosis of the femoral head: a new understanding of the mechanisms of action. J Steroid Biochem Mol Biol. 2009;114(3–5):121–128.
- Sato AY, Tu X, McAndrews KA, et al. Prevention of glucocorticoid induced-apoptosis of osteoblasts and osteocytes by protecting against endoplasmic reticulum (ER) stress in vitro and in vivo in female mice. Bone. 2015;73:60–68.
- Lian JB, Shalhoub V, Aslam F, et al. Species-specific glucocorticoid and 1,25-dihydroxyvitamin D responsiveness in mouse MC3T3-E1 osteoblasts: dexamethasone inhibits osteoblast differentiation and vitamin D down-regulates osteocalcin gene expression. Endocrinology. 1997;138(5):2117–2127.
- Hong D, Chen HX, Xue Y, et al. Osteoblastogenic effects of dexamethasone through upregulation of TAZ expression in rat mesenchymal stem cells. J Steroid Biochem Mol Biol. 2009;116(1–2):86–92.