Abstract
Objective
To develop an effective new method for early detection of pancreatic cancer biomarkers and to aid early clinical diagnosis.
Methods
A DNA probe (Probe) capable of specifically recognizing the target miRNA was designed. The specific probe of miRNA 21 is designed first, and then mixed with the miRNA 21 sample to form a complex molecule, and the complex molecule is added to the nanochannels to detect the received signal. The probe is designed to detect the electrical signal by means of pre-matching and post-matching and observe the stability of the signal. The miRNA 21, miRNA 155, miRNA 196a were added to the nano-single channel to detect the characteristic signals and blocking time. The miRNA 21·probe 21 mixture was mixed with other five cancer-associated microRNAs, and the signal results of the detection were collected and compared.
Results
The signal of miRNA 21 was successfully detected. Whether the probe is designed at the front or the back, there are two signal results. The Probe should be designed to match the middle region of the miRNA. The three microRNA complex molecules have different characteristic signals and blocking times, which can be effectively distinguished.
Conclusion
Nanochannels can effectively detect pancreatic cancer-related microRNAs.
Introduction
In recent years, the incidence of pancreatic cancer has been increasing, and its mortality has been the highest in various malignant tumours in gynaecological diseases. Most patients have been diagnosed as clinically late stages of tumour growth, losing valuable clinical early treatment opportunity. Therefore, the disease poses a serious threat to the life and health of patients and seriously affects the quality of life of patients.
The lack of early diagnosis techniques and the absence of durable and effective treatment options remain a thorny issue in the diagnosis and treatment of pancreatic cancer. At present, the commonly used real-time quantitative qRT-PCR method has problems such as non-specific hybridization and error amplification [Citation1] while the traditional detection method Northern hybridization method has low sensitivity to miRNA detection [Citation2] while other methods mostly require the target molecule is chemically modified or specifically labelled. Since early diagnosis can improve the treatment and prognosis of pancreatic cancer, finding new and effective early markers of pancreatic cancer has become a focus of international research on cancer. Because nanochannels sensors have the advantages of high sensitivity, high selectivity, high throughput, no labelling and no amplification, early detection of pancreatic cancer can be achieved quickly and easily. In 1996, Kasianowicz and his colleagues reported on the application of α-hemolysin nanochannels in nucleic acid detection for the first time at NIST [Citation3]. So far, researchers have also developed various new biological nanochannels [Citation4–7]. Nanochannels single-channel detection technology has also been applied to DNA sequencing [Citation4,Citation6], peptide detection [Citation8–10] and RNA detection [Citation11–14]. Due to the physical occupancy effect of biomolecules, when the biomolecules pass through the nanochannel, the blockage will cause a square wave signal, and when the molecular length is long, the modulation current caused by the lengthening will become longer. Therefore, when the different analyte molecules pass through the nanochannels, the ion current changes differently, and the clogging degree and duration of the characteristic signal are different [Citation15,Citation16]. Therefore, the single nanochannel can be regarded as a natural single molecule detecting sensor to achieve highly sensitive and specific detection of single-molecule levels.
Human plasma contains ribonucleic acid (RNA) [Citation17]. Since the discovery of circulating RNA, people have been studying its application in the diagnosis and early warning of clinical diseases. The expression of circulating RNA is associated with a variety of cancers, and approximately 50% of the circulating RNA that is annotated is located on the genome at a fragile site associated with the tumour, and cancer can be detected by detecting specific messenger RNA (mRNA) in circulating RNA of cancer patients. Diagnostic treatment so plasma RNA as a biomarker has a good application prospect in cancer diagnosis [Citation18,Citation19]. MicroRNAs (miRNAs) are a class of non-coding small RNA molecules that are important regulators of gene expression. Many studies have demonstrated that abnormal miRNA expression is involved in the development and progression of cancer, and different types of miRNAs have different types of cancer [Citation20]. Therefore, it has important clinical guiding significance for the early diagnosis and prognosis of cancer. The study found that miRNAs are abnormally expressed in tissues of pancreatic cancer patients [Citation21], and another study shows that miRNAs in plasma are highly stable [Citation22]. Due to their special regulatory functions, miRNAs are not only in cell differentiation and biology. It plays an important role in the development process, and also plays a huge role in the development of various diseases, such as medical tests, tumours and immunity [Citation23–25]. Therefore, the detection of miRNAs has very important guiding significance in the fields of molecular biology and medicine. Studies have shown that miRNA is a new generation of tumour markers for pancreatic cancer [Citation26]. It has been reported that the occurrence and development of pancreatic cancer were clearly associated with abnormal expression of miRNA 21, miRNA 155 and miRNA 196a [Citation27].
In this study, we designed a DNA probe (Probe) that can specifically recognize the target miRNA. The probe is fully complementary to the target recognition region of the target miRNA and can be used for specific detection of miRNA. The recognition region of the probe with the miRNA ends as the guide strand enables the αHL to rapidly capture the miRNA·probe molecule [Citation28–32]. The main purpose of this study is to achieve high-efficiency detection of pancreatic cancer miRNAs using the high-sensitivity resolution of miRNA·probe complex molecules by αHL single nanochannel. The experimental results show that due to the difference of the probes sequence, the miRNA 21·probe 21, miRNA155·probe 155, miRNA 196a·probe 196a, three complex molecules form different characteristic shapes and different blocking time characteristic current signals, so the characteristic signal can be used effectively distinguish between miRNA 21, miRNA 155 and miRNA 196a.
Materials and methods
Reagents and instruments
Reagents: miRNA, α-Hemolysin (Sigma Aldrich), lecithin (Sigma Aldrich), n-pentane, hexadecane, Teflon membrane (thickness 25 μm, Goodfellow (Malvern, PA)), refer to previous literature [Citation12] in the experiment. The buffer used was prepared from 1 mol/l KCl, 10 mmol/L Tris, and the pH was adjusted to 7.5 with HCl; the H solution was configured with 1 ml of pentane and 100 μL of hexadecane; 25 mg phospholipid and 2.5 ml pentane configuration. All reagents were of analytical grade and the water used in the experiments was ultrapure water (18.2 MΩ·cm, 25 °C).
Instruments: Integrating patch clamp (Axon Instruments, Forest City, CA, USA), Digidata 1 550 series (Axon Instruments, Forest City, CA, USA), Arbitrary Function Generator (Axon Instruments, Forest City, CA, USA), high speed centrifugation Machine, refrigerator, electronic balance, numerical water bath temperature controller, automatic double pure water steaming museum, adjustable pipette and pH meter.
Preparation of samples
The miRNAs and probes used in this experiment were synthesized by Shanghai Shenggong Bioengineering Co., Ltd. and purified by HPLC. In this experiment, the miRNA and probe were mixed in equal amounts, first incubated in a 95 °C water bath for 3 min, then immediately placed in a 35 °C incubator for 1 min, and the high and low temperature was repeated three times to prepare a miRNA·probe complex.
Experimental methods
The nanochannels single molecule detection method is to first drop a drop of about 10 μL of a mixture of pentane and hexadecane on both sides of the Teflon film near the micropores, and rapidly blow it off to spread on the film; second, in the detection pool 2 ml of 1.0 mol/l KCl, 10 mmol/L Tris, pH 8.0 buffer were added to each of the two chambers; then 20 μL of phospholipid pentane solution was added to the two chambers to form a phospholipid bilayer; The system applies a + 180 mV transmembrane voltage, adds a certain amount of αHL at the cis end of the detection cell, self-assembles into a stable single nanochannel; finally, the analyte is added at the cis end to record the signal. The current signal uses a 5 kHz low-pass filter with a sampling frequency of 50 kHz. The resulting data were analyzed using ClampFit 10.4 (Molecular Devices, ForestCity, CA, USA) and OriginLab 8.5.
Experimental results and analysis
Nanochannel detection miRNA detection principle
Nano-single channel detection of miRNAs is based on the principle of DNA hybridization (see ). At a certain voltage, the falling pulse current generated by different DNA or RNA molecules passing through the nanochannel is different [Citation33,Citation34]. The width of the pulse and the blocking time are related to the number of bases, and the number of pulses is related to the concentration. The length of the microRNA is usually 21–23 nucleotides and the lengths of the various microRNAs are similar. When the probe and the targeting microRNA pass through the channel separately, the molecules are smaller than the nanopore, so they pass through the channel very quickly and the signal is relatively short; when the probe is paired with the target, since the molecule is larger than the nanopore, it takes longer to pass through the channel and the signal is long. Therefore, the length of binding of the probe to the targeted microRNA and the number of bases are the key to determining the dwell time. When the Probe is paired with the Target, but there is a base mismatch, the blocking time through the channel is also shorter than the full pairing time, and the signal is relatively short.
Comparative experiments of different miRNAs
is a signal diagram of Probe (GAA TAG TCT GAC TAC A), miRNA 21 and miRNA 21·probe 21 when passing through a single nanochannel and its corresponding scatter analysis. is the average blocking time value of several nucleic acids mentioned above. Amplitude is the magnitude of the pulse current. It can be seen that the pulse currents of different nucleic acids pass through the nanochannels are different, and the blocking time is different. The pulse current and blocking time of probe, miRNA 31 and miRNA 31·probe 31 increase sequentially. And, the experimental results showed that the pulse current and the blocking time of the miRNA when it passed through the nanochannels were related to the length of the chain and the number of bases.
Figure 2. Nanochannels detection of various nucleic acid samples and corresponding scatter diagrams.
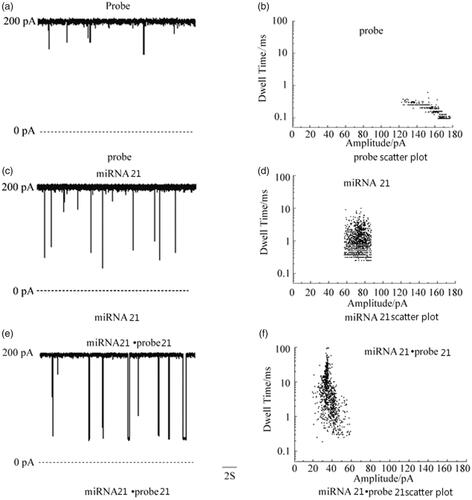
Figure 3. Mean residence time values of various nucleic acid samples. Amplitude is the magnitude of the pulse current. It can be seen that the pulse currents of different nucleic acids pass through the nanochannels are different, and the blocking time is different. The pulse current and blocking time of probe, miRNA 31 and miRNA 31·probe 31 increase sequentially.
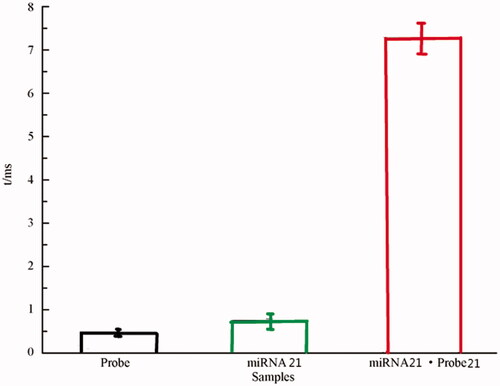
Comparative experiment with different pairing positions of miRNA 21
The miRNA 21·probe 21 paired between the Probe and miRNA 21 designed in Experiment 2.2, in order to better verify that the design method is more conducive to the analysis of the later results, a comparative experiment with the position of the different positions of the miRNA 21 was performed. shows a single-channel signal and scatter analysis of the pairing and front-end pairing of Probe and miRNA 21, respectively. It can be seen from the results that both the post-matching (probe: ATC GAA TAG) and the pre-matching (probe: TAC AAC T) of the miRNA have two signals because the order of the positions of the miRNAs through the channels is different. When the tail-paired miRNA enters the channel, if the front end passes first, because the front end part is single-strand, the passage is fast, the blocking time is short, the pulse current is small, and the signal is short. The short signal in the figure may be the tail-end paired miRNA. The signal when the front end passes through the channel, but it may also be the unpaired Target and Probe signals; if the tail end enters the channel first, because the tail end is double-chain, the blocking time is long, the pulse current is large, and the signal is long, then the long signal in the figure is the tail pair is paired with the signal when the miRNA tail first enters the channel. On the contrary, the miRNAs paired with the front end are also the same. The results demonstrate that no matter which end pairing has two signal results through the nanochannel and increase the difficulty of the result analysis, we select the Probe that exactly matches the intermediate position of miRNA 21.
Effective differentiation of three miRNAs and probes
Since the probe–probe can only be completely complementary to the recognition region of the target miRNA, different miRNA probes have different characteristic signals when passing through the nanochannels, so that various miRNAs can be effectively distinguished [Citation35]. Based on this, probe 155(GAT TAG CAC) was designed to detect miRNA 155, and probe 196a(CCA ACA ACA TGA AAC TAC) was designed to detect miRNA 196a. The blocking time of miRNA 155·probe 155 was t = 1.21 ± 0.14 ms (see ), which was about 1/6 of the blocking time of miRNA 21·probe 21 of 7.13 ± 0.34 ms (see ). The blocking time of miRNA 196a·probe 196a was t = 17.25 ± 2.13 ms (see ), which was greater than the blocking time of miRNA 21·probe 21. Therefore, by regulating the probe sequence, the three complex molecules of miRNA 21·probe21, miRNA155·probe 155 and miRNA 196a·probe 196a can have different characteristic signals and blocking time, so that miRNA 21, miRNA 155 and miRNA 196a can be effectively distinguished miRNAs.
Nanochannels detection of mixed miRNAs
Find five other cancer miRNAs: lung cancer miRNA 25, breast cancer miRNA 21, ovarian cancer miRNA 215, gastric cancer miRNA 106a, prostate cancer miRNA 141. The miRNAs of these five cancers were mixed with miRNA 21·probe 21, and then miRNA 21·probe 21 was detected by nanochannels (see ). The results of the analysis showed that there were two types of signals in the mixture: short signals were the other five cancers. The miRNA, long signal is the signal of miRNA 21·probe 21, which can effectively detect miRNA 21·probe 21 and is consistent with the signal of miRNA 21·probe 21 alone, indicating that the method can be quickly synthesized from complex samples. Efficient detection of miRNA 21 also indicates that if the probes of the other five cancers are designed, the method can also detect the respective miRNAs.
Conclusion
1. This paper uses nanochannel technology to study the early detection of pancreatic cancer. In the experiment, a special sequence of probe was designed to bind to the target miRNA, and the miRNA·probe complex molecule was highly sensitive and rapidly detected by the biological nanopore single channel technology, and the high-efficiency detection of pancreatic cancer miRNAs was realized.
2. Three complex molecules form of miRNA 21·probe 21, miRNA155·probe 155, miRNA 196a·probe 196a can have different signal patterns and different clogging time characteristic signals when passing through the nanochannel due to different probe sequences. The effective differentiation of these three miRNAs is finally achieved.
3. Nanochannel technology provides an effective platform for miRNA detection without labelling, amplification, high resolution and high sensitivity. It also has broad prospects for early diagnosis and treatment of cancer.
Ethical approval and consent to participate
This article does not contain any studies with animals performed by any of the authors. All methods are carried out in accordance with relevant guidelines and regulations.
Author contribution statement
Jiasheng Xu: research design and drafting the manuscript.
Kialo Liao: literature search and helping to draft the manuscript.
Zhonghua Fu: help modify articles and collate references.
Zhenfang Xiong: review and revision of the manuscript and writing guidance.
Acknowledgements
Zhenfang Xiong and Zhonghua Fu are the co-corresponding authors of this article; Xiong Zhenfang is the first of the corresponding authors and Fu Zhonghua is the second of the corresponding authors.
Data availability
All data are available. Please contact us to access if it is needed.
Disclosure statement
No potential conflict of interest was reported by the authors.
Reference
- Park JL, Park SM, Kwon OH, et al. Microarray screening and qRT-PCR evaluation of microRNA markers for forensic body fluid identification. Electrophoresis. 2014;35:3062–3068.
- Varallyay E, Burgyan J, Havelda Z. MicroRNA detection by northern blotting using locked nucleic acid probes. Nat Protoc. 2008;3:190–196.
- Kasianowicz JJ, Brandin E, Branton D, et al. Characterization of individual polynucleotide molecules using a membrane channel. Proc Natl Acad Sci USA. 1996;93:13770–13773.
- Cao C, Liao DF, Ying YL, et al. Detection of single oligonucleotide by an aerolysin nanochannels. Acta Chim Sinica. 2016;74:734–737.
- Wang S, Zhao Z, Haque F, et al. Engineering of protein nanochannelss for sequencing, chemical or protein sensing and disease diagnosis. Curr Opin Biotechnol. 2018;51:80–89.
- Manrao EA, Derrington IM, Laszlo AH, et al. Reading DNA at single-nucleotide resolution with a mutant MspA nanochannels and phi29 DNA polymerase. Nat Biotechnol. 2012;30:349–353.
- Nicole R. Disruptive nanochannelss. Nat Methods. 2013;10:35.
- Stefureac RI, Long YT, Kraatz HB, et al. Transport of α-Helical peptides through α-Hemolysin and aerolysin pores. Biochemistry. 2006;45:9172–9179.
- Sutherland TC, Long YT, Stefureac RI, et al. Structure of peptides investigated by nanochannels analysis. Nano Lett. 2004;4:1273–1277.
- Wang HY, Ying YL, Li Y, et al. nanochannels analysis of β-amyloid peptide aggregation transition induced by small molecules. Anal Chem. 2011;83:1746–1752.
- Hu ZL, Du JH, Ying YL, et al. Single-molecule analysis of colorectal cancer-associated MicroRNAs via a biological nanochannels. Acta Chim Sinica. 2017;75:1087–1090.
- Jasinski D, Haque F, Binzel DW, et al. Advancement of the emerging field of RNA nanotechnology. ACS Nano. 2017;11:1142–1164.
- Smith JA, Braga A, Verheyen J, et al. RNA nanotherapeutics for the amelioration of astroglial reactivity. Mol Ther Nucleic Acids. 2018;10:103–121.
- Haque F, Pi F, Zhao Z, et al. RNA versatility, flexibility, and thermostability for practice in RNA nanotechnology and biomedical applications. Wires RNA. 2018;9:e1452.
- Wang G, Zhao Q, Kang X, et al. Probing mercury(II)-DNA interactions by nanochannels stochastic sensing. J Phys Chem B. 2013;117:4763–4769.
- Wang L, Han Y, Zhou S, et al. nanochannels biosensor for label-free and real-time detection of anthrax lethal factor. ACS Appl Mater Interfaces. 2014;6:7334–7339.
- Kamm RC, Smith AG. Nucleic acid concentrations in normal human plasma. Clin Chem. 1972;18:519–522.
- Xu C, Haque F, Jasinski DL, et al. Favorable biodistribution, specific targeting and conditional endosomal escape of RNA nanoparticles in cancer therapy. Cancer Lett. 2018;414:57–70.
- Pi F, Zhang H, Li H, et al. RNA nanoparticles harboring annexin A2 aptamer can target ovarian cancer for tumor-specific doxorubicin delivery. Nanomedicine. 2017;13:1183–1193.
- Lu J, Getz G, Miska EA, et al. MicroRNA expression profiles classify human cancers. Nature. 2005;435:834–838.
- Lorio MV, Ferracin M, Liu CG. MicroRNA gene expression deregulation in human breast cancer. Cancer Res. 2005;65:7065–7070.
- Chen X, Ba Y, Ma L, et al. Characterization of microRNAs in serum: A novel class of biomarkers for diagnosis of cancer and other diseases. Cell Res. 2008;18:997–1006.
- Yuan Y, Ru Q. Progress of microRNA detection technology and its application in laboratory medicine. Med Information. 2011;8:3809–3809.
- Shi M, Qiu X, Fan H. Advances in plasma miRNA detection in tumor clinical applications. J Southeast Univ (Med Sci Edi). 2012;21:122–125.
- Xiao CC, Rajewsky K. MicroRNA control in the immune system: basic principles. Cell. 2009;136:26–36.
- Zhao H, Shen J, Medico L, et al. A pilot study of circulating miRNAs as potential biomarkers of early stage breast cancer. PLoS ONE. 2010;5:e13735.
- Xueying Z, Linlin Z, Xuedong HAN, et al. Circulating miR-21 in plasm of patient with breast cancer. Modern Med J. 2012;40:133–137.
- Ivica J, Williamson PTF, de Planque MRR. Salt gradient modulation of microRNA translocation through a biological nanopore. Anal Chem. 2017;89:8822–8829.
- Ayub M, Stoddart D, Bayley H. Nucleobase recognition by truncated α-hemolysin pores. ACS Nano. 2015;9:7895–7903.
- Howorka S, Cheley S, Bayley H. Sequence-specific detection of individual DNA strands using engineered nanopores. Nat Biotechnol. 2001;19:636–639.
- Perera RT, Fleming AM, Peterson AM, et al. Unzipping of A-form DNA-RNA, A-form DNA-PNA, and B-form DNA-DNA in the α-hemolysin nanopore. Biophys J. 2016;110:306–314.
- Japrung D, Henricus M, Li Q, et al. Urea facilitates the translocation of single-stranded DNA and RNA through the alpha-hemolysin nanopore. Biophys. J. 2010;98:1856–1863.
- Long Z, Zhan S, Gao P, et al. Recent advances in solid nanopore/channel analysis. Anal Chem. 2018;90:577–588.
- Gao P, Ma Q, Ding D, et al. Distinct functional elements for outer-surface anti-interference and inner-wall ion gating of nanochannels. Nat Commun. 2018;9:4557.
- Ding D, Pengcheng G, Qun M, et al. Biomolecule-functionalized solid-state ion nanochannels/nanopores: features and techniques. Small. 2019;undefined:e1804878.