Abstract
Photothermal therapy (PTT) is a rapidly developing approach for cancer therapy, which has been widely recognized to exert high efficacy as compared to chemotherapy. However, the limited tumour homing property of currently available drug delivery systems (DDSs) is the bottleneck for the efficient delivery of photothermal agents. Here in this study, we surface modified silica nanoparticles (SLN) with the cell membrane (CM) derived from 143B cells to construct a platform (CM/SLN) capable of targeting the homogenous 143B cells. In addition, indocyanine green (ICG) as a photothermal agent was encapsulated into CM/SLN to finally construct a DDS suitable for tumour-targeted PTT of osteosarcoma. Our results revealed that CM/SLN/ICG was mono-dispersed core-shell nanoparticles with advanced stability in a physiological environment. Moreover, due to the modification of CM, CM/SLN/ICG could specifically target the homogenous 143B cells both in vitro and in vivo, which demonstrated superior anticancer efficacy when compared with either SLN/ICG or free ICG. Hence, CM/SLN/ICG could be a promising DDS for tumour targeted PTT of osteosarcoma.
Introduction
Drug delivery systems (DDS) based on nanoparticles have been demonstrated to exert enhanced bioavailability while at the same time can greatly reduce undesired side effects as compared to free drugs, which has been well recognized as a promising alternative for effective cancer therapy [Citation1–3]. Therefore, different kinds of DDSs based on various materials (varying from organic to inorganic ones) have been explored [Citation1,Citation4–6]. Silica nanoparticles (SLN) as one of the most widely adopted material is emerging as a promising candidate due to its versatile merits [Citation7–9]. Therefore, many DDSs have been developed using SLN as the skeleton that resulted in preferable in vitro and in vivo outcomes [Citation10–12].
Nevertheless, the untargeted delivery remains to be one major drawback of currently available SLN based DDSs that requires further efforts [Citation13,Citation14]. To address this issue, the integration of corresponding targeting ligands into the DDS has been adopted [Citation15,Citation16]. In the past decades, targeting ligands varying from small molecules to monoclonal antibodies have been successfully integrated into DDS to improve its tumour targeting capabilities [Citation17–19]. However, some unexpected side effects, such as immunoreaction as well as cytotoxicity are raised due to the ectogenic nature of these ligands and the complicated synthetic process which might contain by-products.
Photothermal therapy (PTT) is a rapidly developing technic that employs photothermal agents to transfer NIR light into heat to exert cytotoxic on cells. As a noninvasive and harmless approach, PTT is well recognized as a preferable measure for cancer therapy [Citation20–22]. Indocyanine green (ICG) is known as a commonly adopted PTT agent both in experimental and clinical applications, which has irreplaceable advantages over other agents [Citation23]. However, ICG is also found to have some limitations, including irreversible degradation, short blood half-life as well as rapid photobleaching, which call for the aid of multifunctional DDSs [Citation24].
Cellular plasma membranes embedding various proteins in the phospholipid bilayer have been found to be involved in many vital biological processes. In addition, the CM also shows high biocompatibility and inherit the merits of the source cells [Citation25]. As a result, various cell membranes (CM) have been employed to generate bioinspired DDSs for the therapy of corresponding diseases [Citation26]. For example, erythrocyte-derived membrane was employed to modify nanoparticle which showed preference to accumulate to inflammation sites [Citation27]. On the basis of these reports, previous studies have explored the possibility of employing platelet membrane for the engineering of tumour-targeting nanoparticles for multiple carcinomas due to the interaction between platelet and tumour cells [Citation28,Citation29]. Therefore, we suggested that cancer cell-derived membranes could functionalize nanocarriers with specific tumour-homing ability to homologous cancer cells to increase the delivery efficacy.
With the aim to combine CM and ICG in one DDS for effective PTT, amine SLN with a positive surface charge was synthesized and ICG was loaded into the matrix of SLN during the synthesis process. Afterwards, negatively charged CM derived from 143B cells was coated onto the surface of ICG loaded SLN to construct a tumour-homing DDS (CM/SLN/ICG). It was anticipated that CM modification of the DDS can positively guide the CM/SLN/ICG to the homogenous 143B cells to positively increase drug accumulation within the tumour tissue and facilitate the subsequent cellular uptake. Afterwards, the released ICG molecule within cancer cells could achieve sufficient PTT of osteosarcoma upon the irritation of external NIR light.
Materials and methods
Materials
Methyl thiazolyl tetrazolium (MTT), ICG, N-(2-aminoethyl)-3-aminopropyltrimethoxysilane (AEAPS), Triton X-100 and tetraethyl orthosilicate (TEOS) were produced by Sigma-Aldrich (St. Louis, MO, USA). Chemicals and reagents otherwise stated were purchased from Aladdin Co., Ltd (Shanghai, China) and were of analytical purity.
Cells and animals
The 143B cell line (human osteosarcoma carcinoma) was a gift from American Type Culture Collection and cultured in standard SPFII condition as reported previously [Citation30].
Female Balb/c nude mice (6 ∼ 8 weeks) were obtained from the MARC of Nanjing University (Nanjing, China) and the 143B tumour xenograft model was established as previously reported [Citation31]. All animal experiments were reviewed and approved by the Institutional Ethics Committee of the Shanghai Sixth People’s Hospital.
Construction of CM/SLN/ICG
The ICG loaded amine SLN was prepared in a water-in-oil microemulsion using previously reported protocol [Citation23]. Briefly, a water-in-oil microemulsion containing 100 μg ICG was prepared under gentle agitation for 0.5 h. Afterwards, the proper amount of TEOS, AEAPS and NH4OH were successively added into the microemulsion to initiate the reaction. After being stirred for 24 h at room temperature, the solution was charged with additional ethanol, followed by centrifugation (3000 rpm, 10 min, CR21, Hitachi, Japan) to obtain ICG loaded amine SLN as a precipitate. The product was washed with ethanol and water, respectively, and stored at 4 °C until usage.
The isolation of CM from 143B cells was performed according to a previous report [Citation32]. In brief, the 143B cells were seeded into a culture dish (200 mm) and allowed to grow to 90% confluence. Afterwards, cells were washed with PBS, detached with tyrosin and concentrated in 3 mL of dispersion buffer. The cells were centrifuged at 1000 rpm for 5 min, and the collected pellet was homogenized in 1 mL of extracting buffer. Afterwards, the mixture was further centrifuged at 10,000 rpm for 10 min. The supernatant was again ultracentrifuged (100,000 rpm for 60 min). The CM pellet was resuspended and stored. The protein in CM was quantified by BCA kit (Beyotime, Shanghai, China).
The coating of CM onto SLN/ICG was performed as previously reported. Briefly, 250 μL of SLN/ICG (1 mg/mL) was mixed with different volume 143B CM solution under vortex. Afterwards, the mixture was subjected to probetype sonication (100 W, 5 min). The mixture was further centrifuged (10,000 g, 10 min) to collect CM/SLN/ICG.
The ICG content was determined by UV spectrophotometer (V1100D, MAPADA, China) at 781 nm. To calculate the drug loading content (DLC) of CM/SLN/ICG, the DDS was firstly merged in methanol for 48 h. After being centrifuged at 10,000 rpm for 30 min, and the DLC was determined by calculating the difference between charged ICG and the ICG in the supernatant.
Characterization
The size distribution of CM/SLN/ICG was explored by size analyzer (ZS90, Malvern, UK). The morphology was observed using a transmission electron microscope (TEM, JEM-2100, JEOL, Japan).
Total protein from the CM was extracted using RIPA lysis buffer (Thermo Fisher, USA) following the manufacturer’s instructions, followed by concentration quantification using the BCA kit. Afterwards, the western blot analysis was performed strictly according to the previous report [Citation33]. Briefly, samples were resolved by gel electrophoresis, transferred onto PVDF membrane, stained with corresponding first antibodies (Abcam, UK) and followed by incubation with IRDyeR680CW-labeled second antibody (Abcam, UK). Finally, the blots were observed using densitometer (E-Gel Imager, Thermo-Fisher, USA).
Stability
The CM/SLN/ICG diluted in PBS or mouse plasma was monitored for 48 h. The changes in particle size were recorded. In addition, the fluorescence intensity of CM/SLN/ICG against ICG in solution was also monitored using fluorescence spectrophotometer (F-7000, Hitachi, Japan) for 6 days [Citation24].
Photothermal conversion and in vitro release experiments
The photothermal conversion ability of CM/SLN/ICG was studied using free ICG and PBS as controls. Briefly, 2 mL of different samples were irradiated by 1 W/cm2 of 808 nm laser for 300 s and the changes in solution temperature during this process was monitored.
The ICG release from CM/SLN/ICG was studied using previously reported protocol [Citation34]. In brief, CM/SLN/ICG was loaded into dialysis bag, immersed in 25 mL of PBS (pH of 7.4 and 5.5), and fixed in a thermostatic shaker (SY-2230; Crystal Technology, Texas, USA). At different time points, the aliquot buffer was withdrawn to determine the drug concentration using UV spectrophotometer as mentioned above.
Cytotoxicity assay
Drug-free nanoparticles (5–100 μg/mL) or CM/SLN/ICG (ICG concentration, 0.25–5 μg/mL) were cultured with 143B cells for 48 h and then subjected to a standard MTT assay. The irradiation wavelength was 808 nm at 1 W/cm2 for 5 min in all ICG containing groups [Citation35].
Cellular uptake
In order to observe the internalization profile of CM/SLN/ICG in 143B cells. Cells were firstly seeded in 6-well plates with 70% confluence and then cultured with free ICG, SLN/ICG and CM/SLN/ICG at the ICG concentration of 2 μg/mL. For competitive binding assay, cells were pretreated with excessive CM for 2 h prior to the addition of formulations. At prearranged time intervals, cells were rinsed with fresh HBSS and then subjected to confocal laser scanning microscope (CLSM, BX61W1-FV1000, Olympus, Japan) observation. In addition, cells were harvested and analyzed by flow cytometer (FCM, Attune NxT, Thermo Fisher, USA) [Citation36].
In vivo drug localization
Mice bearing 143B tumour were intravenously administered with SLN/ICG and CM/SLN/ICG (ICG concentration: 5 μg/mouse). After 48 h of injection, mice were sacrificed and the fluorescence intensity of both formulations in tumour tissues and major organs was assessed using a fluorescence imaging system (ZEWTON 7.0, Vilber, France).
In vivo study of the antitumour ability
143B tumour bearing mice were randomly divided into 4 groups (n = 6) and administered with saline (as control), free ICG, SLN/ICG and CM/SLN/ICG (5 mg/kg ICG). The irradiation wavelength was 808 nm at 2 W/cm2 for 5 min in all ICG containing groups. The administration and other protocols followed the previous report [Citation37].
Results and discussion
CM/SLN/ICG characterization
In order to combine decent drug-loading as well as high biocompatibility in one DDS, SLN as a widely adopted biomaterial was adopted for the construction of the core material using a previously reported water-in-oil microemulsion method. Afterwards, the optimal ratio between CM protein and SLN/ICG was explored by mixing SLN/ICG with different ratios of CM (SLN/ICG to CM protein, w/w). The particle size and zeta potential of different ratios were recorded. As indicated in , the SLN/ICG had a hydrodynamic size of 85.7 ± 0.7 nm and a zeta potential of +35.4 ± 1.8 mV. After coating with CM, the particle size of the product gradually increased as the amount of CM increased while the zeta potential decreased. This phenomenon suggested that SLN/ICG were successfully coated with CM. Interestingly, when the mass ratio exceeds 5, both the particle size and zeta potential of CM/SLN/ICG reached a plateau with additional CM, resulting in significant changes. In detail, the hydrodynamic diameter of the DDS became 105.4 ± 3.2 nm and the surface charge changed to −31.6 ± 2.5 mV (similar to that of the PM vesicles), suggesting that the surface deposition of CM reached a balance. The negative surface charge of CM/SLN/ICG was expected to protect the nanoparticles from nonspecific protein absorption, which in turn ensured the stability of CM/SLN/ICG within circulation [Citation38].
Figure 1. (A) Varaiation of particle size and zeta potential as a function of CM ratio (SLN/ICG to CM protein, w/w). Each sample was repeated in triplicate and shown as mean ± SD (B) Western blot analysis of two proteins (AT1R and CXCR4) in CM and CM/SLN/ICG. (C) TEM images of SLN/ICG, CM and CM/SLN/ICG. Scale bar: 100 nm.
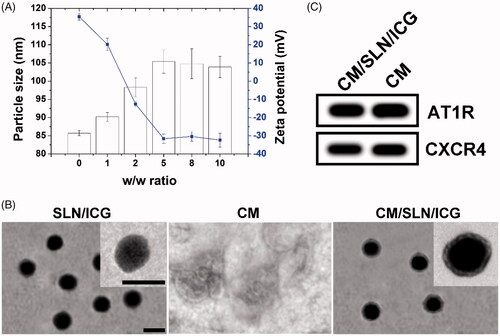
The formation of CM/SLN/ICG from SLN/ICG was also stepwise verified using TEM. As shown in , TEM revealed that SLN/ICG exhibited uniform and spherical nanostructure with homogeneous diameter dispersion at around 80 nm. Single CM showed the irregular shape of particles with a diameter ranging from nanometer to micrometer. The finally obtained hybrid CM/SLN/ICG was observed to be spherical nanoparticle and has a core-shell structure with clearly observed lipid bilayer on the surface.
It has been demonstrated that proteins on CM are essentially responsible for the tumour-targeting capability of the modified nanoparticles [Citation39]. As a result, we selected two different membrane proteins (AT1R and CXCR4) and compared their expression pattern in both CM and CM/SLN/ICG using western blot assay. As displayed in , the expression levels of both proteins in both groups were comparative, which suggested that CM/SLN/ICG inherited the integrated protein profiles of CM without lost or degradation during the coating process. It was also considered as a solid evidence for the verification of successful preparation of CM/SLN/ICG.
The DLC of ICG in CM/SLN/ICG determined by UV spectrophotometer was 18.95%.
Stability assay
The stability of nanoparticles in physiological environment is generally considered as one of the basic requirements if a DDS is intended to successfully deliver the cargos. According to the previous report, the in vivo fate of the DDS is deeply related to its size. As a result, the capability to maintain its size without significant variation is vital for DDS to bypass the complex extracellular barriers before arriving at the targeted sites [Citation40]. Herein, the size change of CM/SLN/ICG in PBS (pH 7.4) and mouse plasma was monitored for 48 h to assess its colloidal stability. As shown in , the size of CM/SLN/ICG in both PBS and plasma at all tested time points showed insignificant changes, which suggested that CM/SLN/ICG might be able to withstand the irritation during circulation. On the other hand, ICG is prone to degrade upon light irritation in aqueous solution, which was reflected as photobleaching. The DDS was expected to retard this process and increase the resistant of ICG to light irritation. As a proof of concept, the comparative fluorescence stability of CM/SLN/ICG and free ICG, under sunlight irritation for 6 days, was studied. As displayed in , free ICG showed immediate photobleaching as the fluorescence intensity dropped dramatically to 68.4% only 1-day post irritation, which continuously declined to 19.8% at the end of the test. In contrast, the fluorescence intensity in CM/SLN/ICG group showed much more retarded decrease as only 21.3% of the ICG suffered from photobleaching at the end of the test. These results suggested that CM/SLN/ICG was capable of protecting the encapsulated ICG from light-induced degradation, which is favourable for CM/SLN to be developed as a stable system to satisfy further applications [Citation41].
Photothermal conversion and in vitro release
The photothermal conversion efficiency of CM/SLN/ICG was reflected as the ability to increase the solution temperature upon laser irradiation. As shown in , under laser irradiation (1 W/cm2) for 5 min, the significant increase in temperature was observed both in of CM/SLN/ICG and free ICG groups. Compared to PBS, which only increased from room temperature (25 °C) to 29.1 °C, the final temperature in CM/SLN/ICG and free ICG groups was 57.93 and 57.21 °C, respectively, which indicated that the ICG molecule within CM/SLN/ICG was well protected to exert comparable photothermal conversion capability to free ICG.
Figure 3. (A) The comparative photothermal conversion profile of CM/SLN/ICG. PBS was employed as control. (B) The drug release of CM/SLN/ICG under different pH conditions (7.4 and 5.5). Each sample was repeated in triplicate and shown as mean ± SD.
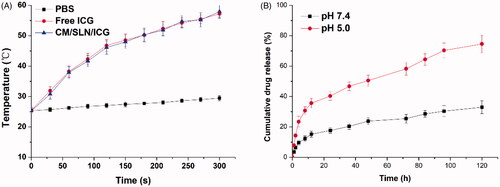
Afterwards, the drug release pattern of CM/SLN/ICG was evaluated in two different pH conditions (pH 7.4 and pH 4.5) to mimic the extracellular physiological environment and intracellular endolysosomal compartment. As illustrated in , after 120 h of incubation, the accumulated drug release of CM/SLN/ICG at pH 7.4 was relatively slower (32.96%) compared to that of pH 5.5 (74.61%). It was inferred that the acidic environment could facilitate the drug release from CM/SLN/ICG, which was beneficial for CM/SLN/ICG to serve as a responsive platform for advanced drug delivery.
In vitro anticancer assay
The standard MTT assay was adopted in our study to investigate the in vitro anticancer efficiency of CM/SLN/ICG. The viability of 143B cells exposed to drug-free CM/SLN still over 90% at the highest concentration (), which suggested that CM/SLN hold preferable biocompatibility with negligible cytotoxicity. The following cytotoxicity assay using drug loaded formulation was shown in . It was observed that under laser irritation, CM/SLN/ICG showed much more superior anticancer efficacy than the other two groups at all tested concentrations. To further verify this conclusion, the apoptosis-related proteins (normal and cleaved caspase-3) in three tested groups (ICG concentration: 5 μg/mL) were assessed. As shown in the inserted images, as expected, the level of cleaved caspase-3 (responsible for the initiating of apoptosis) was higher in CM/SLN/ICG treated cells while normal caspase-3 was much lower than other groups, which further confirms the preferable anticancer efficacy of CM/SLN/ICG.
Figure 4. (A) Biocompatibility of drug free CM/SLN in 143B cells. (B) Cytotoxicity of free ICG, SLN/ICG and CM/SLN/ICG (with laser irritation) at different ICG concentrations against 143B cells after 48 h incubation. Inserted images demonstrated the normal and cleaved caspase-3 levels in three groups (ICG concentration: 5 μg/mL). Each sample was repeated in triplicate and shown as mean ± SD.
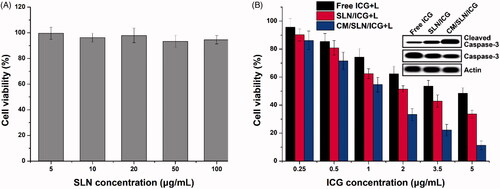
Cellular uptake assay of CM/SLN/ICG
In order to reveal the potential reasons responsible for the discriminative anticancer efficacy of different formulations, in vitro cellular uptake assay was performed to investigate if 143B CM modification could increase the internalization of CM/SLN/ICG to 143B cells [Citation27,Citation39].
As displayed in , the increase of fluorescence signal positively related to the incubation time in both groups. However, free ICG showed the most inferior intracellular accumulation compared to both nanoparticles, which was in consistency with the previous report that nanoparticles can improve the uptake of drugs into cells [Citation42]. In addition, higher ICG signals were also observed in CM/SLN/ICG group than SLN/ICG under all tested conditions. In order to reveal the role of CM in cellular uptake, cells were pretreated with excess CM prior to the addition of nanoparticles. Interestingly, it was observed that the cellular ICG intensity in CM/SLN/ICG group suffered a significant decline while SLN/ICG group showed insignificant variations. These results suggested that the internalization of CM/SLN/ICG into 143B cells was positively related to CM modification, possibly via CM mediated endocytosis.
In vivo drug localization
143B CM modification was expected to assist the tumour-homing of CM/SLN/ICG to the isogenous 143B cells to increase the accumulation of nanoparticles in the tumour tissue. In order to verify the hypothesis, the fluorescence distribution of tumour and major organs obtained by ex vivo imaging was recorded and shown in . It was observed that due to the poor tumour targetability of SLN/ICG, the ICG signal in this group was shown to be largely located in liver and kidney. In contrast, the distribution profile of CM/SLN/ICG is significantly changed alleviate with less accumulation in liver and kidney while enhanced homing to tumour tissue.
In vivo study of the antitumour ability
In vivo anticancer efficacy of CM/SLN/ICG was assessed. The tumour growth profiles of different formulations were displayed in . It was observed that free ICG or SLN/ICG could exert certain suppression effects on tumour growth. By contrast, the anticancer effect of CM/SLN/ICG was much more potent than the others with a final tumour volume of 32 ± 12 mm3 at the end of the test. Moreover, body weight variations of mice in different groups also revealed some interesting results. As shown in , no significant decline in body weight was observed in CM/SLN/ICG group, suggesting that the preferable tumour homing of CM/SLN/ICG could increase its anticancer efficacy while reducing the undesired toxic effects [Citation43]. In contrast, the untargeted distribution of SLN/ICG caused systematic toxicity to mice which was reflected by the time-dependent decrease in body weight. Moreover, TUNEL assay was conducted to assess the apoptosis profile of tumour tissues treated by different formulations (). Compared to the insignificant apoptosis in the saline group, significantly increased apoptosis was triggered in other groups with the most serious one observed in CM/SLN/ICG group, which was consistent with the above-mentioned results. In a word, the CM/SLN/ICG holds great potential to be a preferable tumour targeting DDS for effective PTT of osteosarcoma.
Figure 6. The in vivo antitumour assay of CM/SLN/ICG. (A) and (B) represent the time-dependent tumour volume and body weight, respectively, of mice treated with different formulation. (C) represents the TUNEL staining (200×) of tumour tissue at the end of therapy. Each sample was repeated in sextuplicate and shown as mean ± SD.
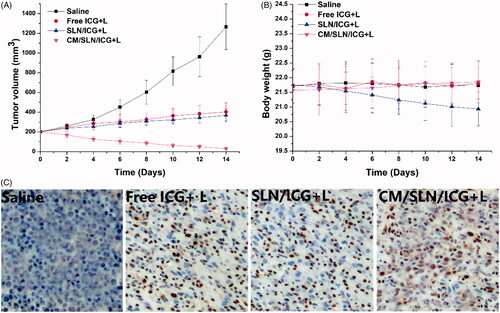
Conclusion
In this work, we successfully developed an ICG loaded and tumour-targeting DDS using SGC7901 CM modified SLN (CM/SLN/ICG). In this platform, we combined the tumour targeting nature of CM and the decent loading capacity of SLN. Experimental results revealed that CM/SLN/ICG was able to specifically deliver ICG to isogenous 143B cells. Furthermore, the CM/SLN/ICG was able to release the loaded drug in a pH-dependent manner. It was noted that CM/SLN/ICG showed superior tumour targeting ability with greatly enhanced anticancer efficacy than unmodified SLN and free drug both in vitro and in vivo.
Disclosure statement
No potential conflict of interest was reported by the authors.
Correction Statement
This article has been republished with minor changes. These changes do not impact the academic content of the article.
Additional information
Funding
Reference
- Da SP, de Freitas ES, Bernegossi J, et al. Nanotechnology-based drug delivery systems for treatment of tuberculosis–a review. J Biomed Nanotechnol. 2016;12:241–260.
- Wang C, Liu X, Chen S, et al. Facile preparation of phospholipid–amorphous calcium carbonate hybrid nanoparticles: toward controllable burst drug release and enhanced tumour penetration. Chem Commun. 2018;54:13080–13083.
- Xiong H, Du S, Zhang P, et al. Primary tumour and pre-metastatic niches co-targeting “peptides-lego” hybrid hydroxyapatite nanoparticles for metastatic breast cancer treatment. Biomater Sci. 2018;6:2591–2604.
- Huang H, Shi H, Liu J, et al. Co-delivery of all-trans-retinoic acid enhances the anti-metastasis effect of albumin-bound paclitaxel nanoparticles. Chem Commun. 2017;53:212–215.
- Wang C, Chen S, Wang Y, et al. Lipase-triggered water-responsive “Pandora’s Box” for cancer therapy: toward induced neighboring effect and enhanced drug penetration. Adv Mater. 2018;30:1706407.
- Zhao X, Tang D, Yang T, et al. Facile preparation of biocompatible nanostructured lipid carrier with ultra-small size as a tumour-penetration delivery system. Coll Surf B: Biointerf. 2018;170:355–363.
- Song Y, Li Y, Xu Q, et al. Mesoporous silica nanoparticles for stimuli-responsive controlled drug delivery: advances, challenges, and outlook. IJN. 2016;12:87–110.
- Wei Y, Gao L, Wang L, et al. Polydopamine and peptide decorated doxorubicin-loaded mesoporous silica nanoparticles as a targeted drug delivery system for bladder cancer therapy. Drug Deliv. 2017;24:681.
- Xie X, Li F, Zhang H, et al. EpCAM aptamer-functionalized mesoporous silica nanoparticles for efficient colon cancer cell-targeted drug delivery. Euro J Pharma Sci. 2016;83:28–35.
- Chen X, Sun H, Hu J, et al. Transferrin gated mesoporous silica nanoparticles for redox-responsive and targeted drug delivery. Coll Surf B: Biointerf. 2017;152:77.
- Li X, Zhao W, Liu X, et al. Mesoporous manganese silicate coated silica nanoparticles as multi-stimuli-responsive T1-MRI contrast agents and drug delivery carriers. Acta Biomaterialia. 2016;30:378–387.
- Zhao J, He Z, Li B, et al. AND logic-like pH- and light-dual controlled drug delivery by surface modified mesoporous silica nanoparticles. Mater Sci Eng C Mater Biol Appl. 2017;73:1–7.
- Tang D, Zhao X, Yang T, et al. Paclitaxel prodrug based mixed micelles for tumour-targeted chemotherapy. RSC Adv. 2018;8:380–389.
- Xiong H, Ni J, Jiang Z, et al. Intracellular self-disassemble polysaccharide nanoassembly for multi-factors tumour drug resistance modulation of doxorubicin. Biomater Sci. 2018;6:2527–2540.
- Peng S, Wang Y, Li N, et al. Enhanced cellular uptake and tumour penetration of nanoparticles by imprinting the “hidden” part of membrane receptors for targeted drug delivery. Chem Commun. 2017;53:11114–11117.
- Zhu D, Tao W, Zhang H, et al. Docetaxel (DTX)-loaded polydopamine-modified TPGS-PLA nanoparticles as a targeted drug delivery system for the treatment of liver cancer. Acta Biomaterialia. 2016;30:144–154.
- Lin C, Zhang X, Chen H, et al. Dual-ligand modified liposomes provide effective local targeted delivery of lung-cancer drug by antibody and tumour lineage-homing cell-penetrating peptide. Drug Delivery. 2018;25:256.
- Xin L, Zhu X, Qiu LJAB. Constructing aptamer anchored nanovesicles for enhanced tumour penetration and cellular uptake of water soluble chemotherapeutics. Acta Biomaterialia. 2016;35:269–279.
- Yan G, Wang J, Hu L, et al. Stepwise targeted drug delivery to liver cancer cells for enhanced therapeutic efficacy by galactose-grafted, ultra-pH-sensitive micelles. Acta Biomaterialia. 2017;51:363.
- Chen Q, Xu L, Liang C, et al. Photothermal therapy with immune-adjuvant nanoparticles together with checkpoint blockade for effective cancer immunotherapy. Nat Commun. 2016;7:13193.
- Yang RM, Fu CP, Fang JZ, et al. Hyaluronan-modified superparamagnetic iron oxide nanoparticles for bimodal breast cancer imaging and photothermal therapy. IJN. 2016;12:197–206.
- Zheng T, Li GG, Zhou F, et al. Gold-nanosponge-based multistimuli-responsive drug vehicles for targeted chemo-photothermal therapy. Adv Mater. 2016;28:8218–8226.
- Gu L, Shi T, Sun Y, et al. Folate-modified, indocyanine green-loaded lipid-polymer hybrid nanoparticles for targeted delivery of cisplatin. J Biomater Sci Polym Ed. 2017;28:690–702.
- Ding X, Xu X, Zhao Y, et al. Tumour targeted nanostructured lipid carrier co-delivering paclitaxel and indocyanine green for laser triggered synergetic therapy of cancer. RSC Adv. 2017;7:35086–35095.
- Yang N, Ding Y, Zhang Y, et al. Surface functionalization of polymeric nanoparticles with umbilical cord-derived mesenchymal stem cell membrane for tumour-targeted therapy. ACS Appl Mater Interf. 2018;10:22963–22973.
- Fang RH, Yao J, Fang JC, et al. Cell membrane-derived nanomaterials for biomedical applications. Biomaterials 2017;128:69–83.
- Hu C-MJ, Zhang L, Aryal S, et al. Erythrocyte membrane-camouflaged polymeric nanoparticles as a biomimetic delivery platform. Proc Nat Acad Sci USA. 2011;108:10980–10985.
- Gao C, Lin Z, Jurado-Sánchez B, et al. Stem cell membrane-coated nanogels for highly efficient in vivo tumour targeted drug delivery. Small 2016;12:4056–4062.
- Yurkin ST, Wang ZJN. Cell membrane-derived nanoparticles: emerging clinical opportunities for targeted drug delivery. Nanomedicine 2017;12:2007–2019.
- Xiong H, Wu Y, Jiang Z, et al. pH-activatable polymeric nanodrugs enhanced tumor chemo/antiangiogenic combination therapy through improving targeting drug release, J. Colloid Interface Sci. 2019;536:135–148.
- Wang C, Han M, Liu X, et al. Mitoxantrone-preloaded water-responsive phospholipid-amorphous calcium carbonate hybrid nanoparticles for targeted and effective cancer therapy. IJN. 2019; 14:1503–1517.
- Suski JM, Magdalena L, Aleksandra W, et al. Isolation of plasma membrane-associated membranes from rat liver. Nat Protoc. 2014;9:312–322.
- Cao Y, Song J, Ge J, et al. MicroRNA-100 suppresses human gastric cancer cell proliferation by targeting CXCR7. Oncol Lett. 2018;15:453–458.
- Wang C, Chen S, Yu Q, et al. Taking advantage of the disadvantage: employing the high aqueous instability of amorphous calcium carbonate to realize burst drug release within cancer cells. J Mater Chem B. 2017;5:2068–2073.
- Wang C, Wang Z, Zhao X, et al. DOX loaded aggregation-induced emission active polymeric nanoparticles as a fluorescence resonance energy transfer traceable drug delivery system for self-indicating cancer therapy. Acta Biomaterialia. 2019;85:218–228.
- Zhang X, Li Y, Wei M, et al. Cetuximab-modified silica nanoparticle loaded with ICG for tumour-targeted combinational therapy of breast cancer. Drug Deliv. 2019;26:129–136.
- Meng LX, Ren Q, Meng Q, et al. Trastuzumab modified silica nanoparticles loaded with doxorubicin for targeted and synergic therapy of breast cancer. Artif Cell Nanomed B. 2018;46:S556–S563.
- Wu L, Ni C, Zhang L, et al. Surface charge convertible and biodegradable synthetic zwitterionic nanoparticles for enhancing cellular drug uptake. Macromol Biosci. 2016;16:308–313.
- Fang R, Hu C-MJ, Luk BT, et al. Cancer cell membrane-coated nanoparticles for anticancervaccination and drug delivery. Nano Letters 2014;14:2181–2188.
- Hashemi M, Yadegari A, Yazdanpanah G, et al. Functionalized R9-reduced graphene oxide as an efficient nano-carrier for hydrophobic drug delivery. RSC Adv. 2016;6:74072–74084.
- Sheng Y, Chang L, Kuang T, et al. PEG/heparin-decorated lipid–polymer hybrid nanoparticles for long-circulating drug delivery. Rsc Adv. 2016;6:23279–23287.
- Wang Y, Wang C, Ding Y, et al. Biomimetic HDL nanoparticle mediated tumour targeted delivery of indocyanine green for enhanced photodynamic therapy. Coll Surf B: Biointerf. 2016;148:533–540.
- Wang C, Bao X, Ding X, et al. A multifunctional self-dissociative polyethyleneimine derivative coating polymer for enhancing the gene transfection efficiency of DNA/polyethyleneimine polyplexes in vitro and in vivo. Polym Chem. 2015;6:780–796.