Abstract
With the arrival of the precision medicine and personalized treatment era, targeted therapy that improves efficacy and reduces side effects has become the mainstream approach of cancer treatment. Antibody fragments that further enhance penetration and retain the most critical antigen-specific binding functions are considered the focus of research targeting cancer imaging and therapy. Thanks to the superior penetration and rapid blood clearance of antibody fragments, antibody fragment-based imaging agents enable efficient and sensitive imaging of tumour sites. In tumour-targeted therapy, antibody fragments can directly inhibit tumour proliferation and growth, serve as an ideal carrier for delivery of anti-tumour drugs, or manipulate the immune system to eliminate tumour cells. In this review, the excellent physicochemical properties and the basic structure of antibody fragments are expressly depicted depicted, the progress of antibody fragments in cancer therapy and imaging are thoroughly summarized, and the future development of antibody fragments is predicted.
Introduction
To date, the development of efficient cancer treatment and precise cancer diagnosis strategies remains essential. Chemotherapy is widely used in cancer clinical treatment, but long-term treatment with chemotherapy drugs can lead to multi-drug resistance, bone marrow suppression and other adverse reactions [Citation1–3]. In order to improve the survival and cured rate of cancer patients, a revolutionary targeted tumour treatment strategy is proposed [Citation4–7]. Targeted drug can effectively promote the uptake of a drug at a tumour site to improve the therapeutic effect. On the other hand, it also reduces side effects on non-targeted cells.
As a natural ligand with high specificity and affinity, antibody is one of the main research objects in targeted tumour therapy and imaging. Monoclonal antibody (mAb) is first-generation antibody drugs, and also produces exciting results in the treatment of solid tumours and haematological malignancies [Citation8–10]. In view of the outstanding therapeutic effect of mAb, the investment of antibody drug research and development is rapidly increased. Significantly, the Food and Drug Administration (FDA) has approved the clinical application of several mAbs, such as bevacizumab, ipilimumab and trastuzumab [Citation11–13]. However, after long-term clinical application and research, the defects of mAb have been gradually discovered. One major impediment is that murine mAb entering the human body cause a human anti-mouse antibody (HAMA) response [Citation14]. The HAMA response causes a strong allergic reaction and rapidly eliminates mAb in vivo resulting poor tumour uptake. Although it is feasible to alleviate the murine problem of mAbs through grafting the complementarity determining regions (CDR) of mouse antibody in human immunoglobulins, the mAb still have limitations of large volume (four polypeptide chains) and long half-life [Citation15,Citation16].
With the development of genetic engineering technology and profound understanding of antibody structure, miniaturized and modular antibody fragments are emerged. These antibody fragments not only retain important ability to specifically bind antigen but also have more ideal half-life and penetration functions than mAbs. Immunoimaging agents, which are composed of antibody fragments coupled with radionuclides, fluorescent dyes, fluorophores, and nanoparticles, can achieve high-resolution and clearly visible tumour imaging in a variety of ways [Citation17,Citation18]. Moreover, antibody fragments can also be used for targeted-tumour treatments for a variety of methods. Antibody fragments alone inhibit tumour cell proliferation and growth or induce tumour cell apoptosis by interfering with signalling pathways. Notably, antibody fragments serve as perfect carriers for the delivery of anti-tumour active drugs. In addition, antibody fragment with the capacity of manipulating the immune system is the current main research direction of tumour immunotherapy [Citation19,Citation20]. For another, with the development of antibody engineering technology, multiple of antibody fragments that meet the performance requirements can be convenient constructed [Citation21–23]. Due to the excellent physicochemical properties of antibody fragments and the diversity of targeted cancer treatment and diagnostic methods, more and more researchers and research institutions are actively exploring the application of antibody fragments in tumour therapy and imaging.
In this review, the excellent physicochemical properties and basic structure of antibody fragments are clearly depicted, the progress of antibody fragments in the field of cancer therapy and imaging are summarized and analysed, and the future development of antibody fragments is predicted.
Basic structure and characteristics of antibody fragments
Antibody is an immunoglobulin produced by the vertebrate immune system under antigenic stimulation. With the deepening of antibody research, the structure and function of antibody is gradually be understood [Citation24,Citation25]. Two identical light chains and two identical heavy chains form a conventional antibody by disulfide bonds. In general, the heavy chain is composed of one variable region and three constant regions called CH1, CH2 and CH3. The light chain makes up of one variable region and one constant region. Each variable region contains four framework regions and three CDRs. Six CDRs of the heavy and light chains together constitute the antigen-binding site, which determines the specificity of the antibody. Based on the comprehension of antibody structure and the development of immunology, molecular biology, diversified antibody fragments (as illustrated in ) are constructed through antibody engineering. All antibody fragments retain antigen-specific [Citation26]. Meanwhile, current antibody fragment preparation techniques are relatively complete and there are many methods to choose from, such as phage display technology, yeast display technology [Citation27–29].
Single chain variable fragment (scFv)
ScFv is a monovalent antibody fragment consisting of an antibody heavy chain variable region joined to an antibody light chain variable region by a flexible peptide linker. The flexible peptide linker containing a large amount of serine and glycine ensures the stability of the scFv spatial structure. The scFv of the monovalent antigen-binding structure has utter specificity for a single antigen. Currently, low immunogenicity and small volume of scFv have good prospects for cancer therapy. As an example, chimeric antigen receptor T cells comprising anti-mesothelin scFv can redirect T cells to inhibit the growth of gastric cancer [Citation30].
Nanobody
Nanobody, which is a variable region isolated from camelid heavy chain antibodies by phage display technology, is the smallest antigen binding fragment [Citation31]. Camel heavy chain antibodies are naturally occurring single chain antibodies. In addition, similar single antibodies have been found in sharks. Camel heavy chain antibodies are unable to immobilize the light chain due to the separation of CH1 during mRNA formation. Due to the lack of light chains, nanobodies are structurally different from traditional antibodies. The CDR3 of the Nanobody is longer than the conventional antibody, and the steric structure of the CDR3 is a concave structure that can help to bind the antigen. Furthermore, a part of the hydrophobic amino acid residue in the framework region is replaced with hydrophilic amino acid residue. These structural changes allow the nanobody to retain antigen-specific binding ability in the absence of the light chain and improve its hydrophilicity and stability. In another interesting aspect, there is evidence that small volumes of nanobodies can cross the blood-brain barrier for the treatment of brain diseases [Citation32,Citation33].
Fragment of antigen binding (Fab)
Fab is an antibody fragment that can be prepared by a simple enzymatic reaction and is the earliest to appear [Citation34]. A single antibody is broken down into two identical Fabs and one fragment crystallizable (Fc) under hydrolysis of papain. The Fab consisting of the heavy chain variable region, CH1 and intact light chain has a molecular weight of approximately 50 kDa and is capable of binding to a single antigenic epitope [Citation35].
Multivalent antibody fragment and multispecific antibody fragment
Multivalent antibody fragment and multispecific antibody fragments are undergoing numerous studies to further develop new tumour treatment and imaging strategies [Citation36,Citation37]. In general, structurally defective antibody fragments result in reduced affinity for antigen. The antibody fragment can be polymerized by shortening the length of the flexible peptide linker to form a multimer having multiple antigen binding sites. Multivalent antibody fragments can significantly increase the affinity and specificity for antigens.
Multispecific antibodies that target multiple targets simultaneously have excellent tumour therapeutic potential [Citation38]. By targeting different targets or targeting different epitopes of the same target, multispecific antibody fragments can establish intercellular interactions or synergistic effects to exert significant tumour therapeutic effects [Citation39]. In particular, bispecific antibodies are widely used in tumour immunotherapy and have achieved numerous results. For instance, Crawford et al. constructed a bispecific antibody that activates T cells to target ovarian cancer cells [Citation40]. This bispecific antibody produces a long-lasting, sustained therapeutic effect by activating the immune system.
Application of antibody fragment in cancer imaging
Antibody that specifically binds to overexpressed antigen on the surface of the tumour can be combined with radionuclide, fluorescent substance or microbubble into complex for cancer imaging [Citation41–43]. When mAb is involved in tumour imaging, long half-life mAb requires longer imaging time and can only obtain background blurred imaging results [Citation44]. Unlike mAbs, antibody fragments with short half-life and strong permeability are more suitable for tumour imaging [Citation45,Citation46]. Antibody fragment-based tumour imaging is widely used for (1) non-invasive detection of tumours; (2) real-time guidance of surgery; (3) monitoring of tumourigenic receptor expression status, tumour development status, and tumour treatment prognosis [Citation47,Citation48]. At present, imaging diagnosis based on antibody fragments is rapidly developing and various methods have emerged. For example, positron emission tomography (PET), single photon emission computed tomography (SPECT), computed tomography (CT), optical, ultrasound and photo-acoustic imaging [Citation49–51].
Antibody fragment in nuclear imaging
Nuclear imaging plays an important role in the diagnosis of cancer. Nuclear imaging based on antibody fragments can reduce the radiation exposure time of patients compared with other nuclear imaging, so it achieves rapid development in recent years [Citation52]. In antibody fragment-based nuclear imaging, specific targeting antibody fragments and radionuclides are covalently linked by bifunctional chelator to form radioimmunoconjugate that provides clear visualization of tumour lesions. When a radioisotope-labelled antibody fragment is applied to tumour imaging, it can accumulate at the tumour site and has a high tumour to muscle ratio [Citation53]. On the other hand, tumour imaging based on antibody fragments can also evaluate the efficacy of anti-tumour drugs by measuring the absorption of the tracer at the tumour site [Citation54]. Furthermore, imaging techniques can be applied to detect the expression status of tumourigenic receptors to identify tumour subpopulations and to assist in the next step in determining treatment options [Citation55].
However, radionuclide-labelled antibody fragments exhibit high and long-lasting radioactive localization in the kidney, thereby impeding tumour imaging in the kidney region [Citation56]. This is a co-existing problem with antibody fragments for nuclear imaging. This problem is caused by the fact that after the labelled antibody is hydrolyzed by the lysosome, the radioactive metabolite is filtered by the glomerulus and reabsorbed by the renal cells, thus causing high radioactivity in the kidney region. Therefore, strategies for reducing renal absorption are needed in environments where the kidney background is problematic. In one study, a method is proposed to alleviate the problem of high absorption of imaging agents in the kidney [Citation57]. Combining the Met-Val-Lys linker of the enzyme substrate on the kidney brush border membrane with the antibody fragment can effectively reduce the absorption of the radioimmunoconjugate by the kidney. The experimental results show that the complex reduces the amount of renal absorption under maintaining high tumour uptake condition. In other ways, studies have confirmed that the new labelling reagents and the passively targeted nanoparticles as a carrier can also reduce kidney volume [Citation55,Citation58].
Antibody fragment in optical imaging
Biomolecular probes, which are constituted by a fluorescent substance labelled antibody fragment, are one of the main strategies for antibody fragment-specific molecular imaging [Citation59–61]. Half-life, permeability, and biodistribution are important considerations for constructing imaging tracers. Small volumes of antibody fragments can quickly penetrate into the tumour site for efficient tumour imaging. In addition, the deletion of the Fc avoids binding to immune cells to ensure absolute specificity of the antibody fragment. On the other hand, antibody fragments lacking the Fc are unable to bind to the Fc receptor, resulting in a decrease in blood circulation time and a low imaging background. In view of the optimization of antibody fragments in half-life, permeability, and specificity, antibody-based optical imaging has better sensitivity and specificity than conventional tumour diagnostic methods based on anatomical differences between tumour tissue and normal tissue. For instance, Tolner et al. constructed a near-infrared fluorescent tracer based on scFv. The fluorescent tracer selectively accumulates at the tumour site and has an excellent biodistribution, and can be applied to identify colorectal cancer and pancreatic cancer with good clinical transformation prospects [Citation62].
For another, real-time optical imaging has a powerful auxiliary effect, which can improve the efficiency and success rate of tumour surgical resection. An example in this area is the use of A11 Mb-IRDye800CW, a molecular probe produced by a near-infrared dye-labelled PSCA antibody, which capable of targeting prostate stem cell antigen to provide long-lasting fluorescence imaging [Citation63]. Real-time fluorescence-guided surgery can show tumour margins and lymph node metastasis, so fluorescence-guided surgery can assist tumour resection, reduce tumour recurrence rate and improve patient outcomes compared with traditional white light surgery.
Antibody fragment in dual-modality imaging
Dual-modality imaging, which provides non-invasive assessment of preoperative disease and provides real-time fluorescence imaging of tumour margins during surgery, is an important research direction in tumour imaging [Citation64]. Dual-modality imaging results of multiple imaging can be mutually verified, so the imaging results of multimodal imaging are more accurate and reliable than single-modal imaging [Citation65,Citation66]. For example, a dual-labelled bispecific antibody 64Cu-NOTA-heterodimer-ZW800 was synthesized though biorthogonal click chemistry [Citation67]. The heterodimer antibody which can target both tissue factor and CD105 has stronger specific binding ability than homodimers antibody. In addition, heterodimeric antibodies can increase the amount of uptake at the tumour site by an increase in structure. So, the increase in structure also makes the contrast between PET and NIRF imaging results higher. From another aspect, multimodal imaging that compensates for defects in a single imaging method using multiple imaging modalities results in more accurate and specific imaging. Therefore, the development of innovative multimodal imaging can further improve the accuracy of early diagnosis of tumours, help tumour staging, and enhance the precision of surgical guidance.
Antibody fragment in other imaging
Ultrasound imaging is an essential means of tumour imaging. Targeting microbubbles by attaching antibodies to microbubbles can increase the accumulation of imaging agents at the tumour site and improve the accuracy of ultrasound imaging [Citation68]. Fan et al. combine PSMA-targeted nanobody and microbubbles to form targeted nanobubbles by the biotin-streptomycin system [Citation69]. Both in vitro and in vivo results indicate that the targeted nanobubbles can specifically bind to PSMA-positive tumour cells, and the results of ultrasound imaging indicate that the targeted nanobubbles are superior to the blank nanobubbles in the xenograft model. Targeted microbubbles are enriched in tumour cells by active targeting of antibody fragments, thereby improving the accuracy of ultrasound imaging.
In addition, antibody fragments can be applied to photoacoustic imaging with high sensitivity and high resolution. Photoacoustic imaging has a certain improvement in imaging depth compared to conventional optical imaging. In photoacoustic imaging, transient thermoelastic tissue expansion occurs at the imaged portion after pulsed laser irradiation, thereby producing sound waves that can be converted into images by the ultrasonic transducer. In one study, photoacoustic tracers formed by antibody fragment-coupled nanoparticles have better affinity for HER2-posvitive tumour, demonstrating the potential of antibody fragments for photoacoustic imaging [Citation70].
Application of antibody fragment in cancer therapy
The application of antibody-targeted tumour therapy is one of the important measures for clinical treatment of cancer. In order to promote the therapeutic effect of antibodies on tumours and improve the drug properties of antibodies, antibody fragments with excellent biochemical properties become the focus of antibody drug development. Initially, antibody fragments exert anticancer activity by affecting the signalling pathway [Citation71]. As the field of antibody fragment research expands, antibody fragments can also treat cancer by other means. Antibody fragments serve as vectors and targeting moieties in targeted drug delivery systems for the delivery of drugs with anti-tumour activity [Citation72,Citation73]. With the tremendous advances in immunology and oncology, antibody fragments can be applied to manipulate the immune system to treat cancer [Citation74,Citation75]. The discovery of new therapies not only enhances the diversity of antibody fragments in cancer treatment but also combines different therapies to achieve synergistic anti-cancer effects.
Intrinsic therapeutic effect of antibody fragments
Target antigen selection is extremely important when using antibody fragments for treatment, and the antigen of choice is usually associated with angiogenesis, cell growth or inhibition of apoptosis [Citation76]. Target antigen that is highly expressed on tumour cells, but is low or not expressed in normal cells can serve as an ideal attachment site for antibody fragments. For instance, a scFv that specifically binds alpha-fetoprotein (AFP), an important marker of human hepatocellular carcinoma, was constructed and synthesized [Citation77]. The scFv induces cell cycle arrest and tumour cell apoptosis by blocking internalization of AFP.
In addition to single-target antibody fragment, there is also multiple-target antibody fragment for the treatment of tumours. Recently, bispecific antibodies HD105 that inhibit angiogenesis by targeting vascular endothelial growth factor (VEGF) and Delta-like ligand 4 (DII4) are constructed [Citation78]. Both target sites promote tumour angiogenesis in a variety of tumours, and DII4 is also associated with cancer stem cells. The results of this study indicate that HD105 degrades more functional tumour blood vessels and causes more tumour cell apoptosis than targeting VEGF or DII4 alone. In addition, HD105 also avoids tumour metastasis and invasion caused by VEGF treatment. Bispecific antibodies not only synergistically block signalling pathways to improve therapeutic efficacy, but also compensate for drug resistance defects that may be caused by single-target antibody therapy. However, cancer-targeted therapies of individual antibody fragments still only inhibit the proliferation and growth of cancer cells and do not provide significant therapeutic effects. Some possible cause of this problem is the lack of Fc domain in the antibody fragment resulting in the inability to produce Fc receptor-mediated ADCC, or the tumour producing evasive resistance mechanism [Citation79].
Targeted drug delivery of antibody fragment
The delivery of drugs with anti-tumour activity to tumour sites by antibody is currently the most important tumour targeted therapeutic method [Citation80–82]. By gene fusion or chemical coupling, antibody fragments can be bind to anti-tumour active drugs to form a targeted drug delivery system [Citation83,Citation84]. Antibody fragments as vectors and targeting moieties are critical for the construction of targeted drug delivery systems. Drugs transported by targeted drug delivery systems can utilize the active targeting of antibody fragment to aggregate in tumour cells and improve pharmacokinetic properties.
Antibody fragment conjugated to biological effector molecules
The transport of biological effector molecules to cancer sites by antibody fragments is a commonly used targeted transport strategy [Citation85–87]. Biological effector molecule short interfering RNA (siRNA) with silencing gene expression is a potential cancer therapeutic biologic [Citation88,Citation89]. However, when siRNA is used alone, it is easily degraded by serum and stimulates the immune system. In addition to this, siRNA cannot target specific cells and rapid blood clearance results in low tumour uptake. siRNAs carried by antibody can increase accumulation in tumour sites and reduce systemic side effects. Therefore, antibody fragments with high specificity and excellent tumour permeability are used to transport siRNA. An example in this area, CXC chemokine receptor 4 siRNA (CXCR4si) delivered by HER2-scFv-arginine nonamer peptide fusion protein e23sFv-9R is able to target HER2 positive breast cancer cells [Citation90] CXCR4 promotes cancer cell proliferation and invasion through the CXCR4/SDF-1 signalling pathway, while also promoting angiogenesis in primary and metastatic cancers. In vitro and in vivo evaluations have shown that the e23sFv-9R to carry CXCR4si can decrease tumour metastasis, induce apoptosis through cell cycle arrest and prolong the survival of xenograft models, without causing systemic toxicity and innate immune response.
In addition to transporting siRNA, immunotoxins consisting of antibody fragments combined with protein toxins are also the focus of tumour-targeted therapeutic research [Citation86]. Toxins derived from cells or plants are highly cytotoxic, but their immunogenicity and toxic side effects on the human body are limited in the application of tumour therapy. The antibody fragment serves as a guiding module to make the cytotoxicity of the toxin specific, thereby increasing its therapeutic window and reducing systemic toxicity. For example, recombinant immunotoxins 806-PE38 were constructed by gene fusion of scFv and pseudomonas aeruginosa Exotoxin A [Citation87]. The results show that EGFR-targeted immunotoxins can significantly reduce the viability of six triple-negative breast cancer cell lines, can reduce tumour burden and improve survival time in mouse xenograft models. Immunotoxins greatly enhance the tumour therapeutic effect of toxins, but the protein toxin in the immunotoxin is highly immunogenic as a foreign protein resulting in the production of anti-toxin antibodies. Therefore, in order to further promote the clinical transformation of immunotoxins, it is necessary to reduce immunogenicity by some measures. Kaplan et al. introduced mutation sites on the immunotoxin domain and maintained the original spatial structure, thereby significantly reducing the immunogenicity of the immunotoxin [Citation91].
Antibody fragment conjugated to nanoparticles
The coupling of antibody fragments to drug-loaded nanoparticles is an emerging strategy for tumour drug delivery and shows high potential for development [Citation92,Citation93]. Antibody fragment-modified nanoparticles specifically recognize tumour surface antigens and enter the interior of tumour cells via receptor-mediated endocytic pathways, as illustrated in . An example in this field, the immune biocompatible multifunctional nanomedicine is formed by covalently bioconjugated anti-HER2 scFv with PEG coated magnetic iron oxide nanoparticle core [Citation94]. The modified nanoparticles can effectively target the tumour site, and the stability in serum is also significantly improved.
Figure 1. Schematic diagram of traditional antibody and antibody fragment. (Include: mAb; camel heavy chain antibody; nanobody; scFv; bispecific antibody; nanobody bispecific antibody; bivalent antibody fragment; triavalent antibody fragment).
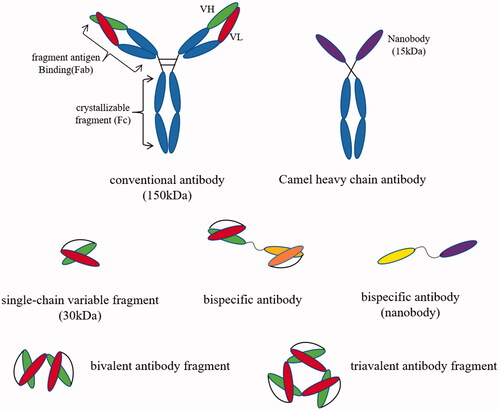
Figure 2. Targeted drug delivery system based on antibody fragments. Targeted delivery complexes that composed of antibody fragments coupled with drug-loaded nanoparticles enter tumour cells by antibody fragment-mediated endocytosis. Under the degradation of lysosomes, the complex releases anti-tumour activity drugs to eradicate tumour cells.
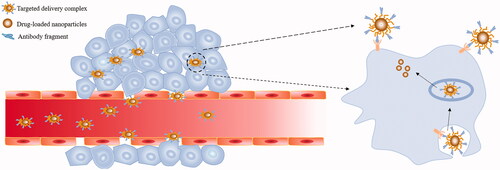
Figure 3. Antibody fragments apply to tumour immunotherapy. (a) Bispecific antibodies help to construct immune synapses between immune cells and T cells, thereby activating T cells to eradicate tumour cells. (b) After the scFv-based CAR-modifies T cells, T cell-deficient recognition of tumour antigens can be avoided and T cell activation is promoted. Engineered CAR-T cells can specifically and efficiently kill tumour cells.
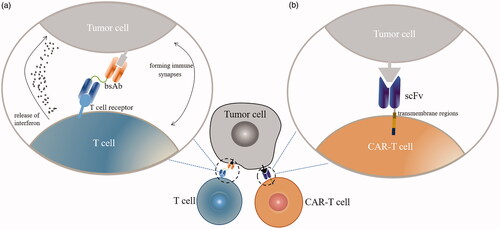
Antibody fragments can modify a variety of nano-carrier system, such as liposomes, micelles, artificial cells, and nanogels [Citation95,Citation96,Citation97]. Li et al. synthesized PH-sensitive immunoliposomes using anti-HER2 Fab fragments to modify PH-sensitive liposomes [Citation98]. The immunoliposomes have no effect on the properties of the Fab fragments and liposomes and are combined with antibody-specific active targeting, passive targeting, and pH sensitivity to greatly enhance tumour targeting function. The results showed that the pH-sensitive immunoliposomes increased the uptake of doxorubicin at the tumour site by antibody specificity and antibody-induced endocytosis, and enhanced the tumour suppressive effect on the tumour-bearing nude mouse model. The difference in tumour cell drug uptake between liposomes modified by antibody fragments and unmodified liposomes suggests that active targeting is more effective than passive targeting.
Antibody fragment-modified targeted drug delivery systems can enhance the anti-tumour effects of drugs through synergistic effects, and construction of targeted drug delivery is currently the most effective cancer treatment strategy for antibody fragments. However, multiple requirements must be met in order to build an impeccable targeted drug delivery system. First, the basic structure and functions of the various components in the targeted drug delivery system cannot be changed much after chemical or biological coupling. In addition, linker with high stability is required to avoid off-targeting in targeted drug delivery systems. Finally, the constructed targeted drug delivery system requires better pharmacokinetics properties.
Immunotherapy of antibody fragment
The immune barrier of tumour cells is composed of fibrous stroma and immunosuppressive cells present in the tumour microenvironment. Tumour cells through blocking antigen presentation and up-regulating the number of regulatory T cells lead to an immune escape, causing tumour cells fail to be detected and removed by the immune system [Citation99–101]. Immunotherapy, which reverses the immune escape mechanism of tumour cells, is the most advanced cancer treatment [Citation102]. At present, antibody fragments can be utilized to cancer immunotherapy by different methods (as illustrated in ), and have an enduring therapeutic effect on both solid tumours and haematological malignancies [Citation103–105].
Immunotherapy through bispecific antibodies
Bispecific antibody produced by antibody engineering techniques can target two different targets simultaneously. Based on this property, bispecific antibodies can be applied to tumour immunotherapy through targeted tumour-associated antigens and receptors on immune cells [Citation106]. For instance, the bispecific antibody CS1-NKG2D for immunotherapy was constructed [Citation107]. CS1-NKG2D is capable of simultaneously targeting the tumour-associated antigen CS-1 with high expression of multiple myeloma and the potent activating receptor NKG2D on the surface of most immune cells. CS1-NKG2D can bind to NKG2D-positive immune cells and then activates various immune cells to produce cytotoxicity by phosphorylating downstream proteins to form protein complexes. The results indicate that CS1-NKG2D can form immunological synapses between CS-1 positive tumour cells and NKG2D positive immune cells in vitro. The immune synapse can direct immune cells to target the removal of cancer cells and significantly prolong the survival time of the mouse xenograft model. Bispecific antibodies can also circumvent the intrinsic or acquired resistance of tumour cells to a subset of targets by directly constructing intercellular linkages. As an example, Satta et al. constructed a bispecific antibody that directly manipulates T cells to target the removal of resistant TRAIL-R2 positive tumours [Citation108]. This method addresses the problem of inadequate therapeutic effect due to drug resistance when targeting TRAIL-R2 alone. In addition, its anti-tumour therapeutic effect can be further enhanced by adjusting the bispecific antibody format. For example, a dimerized bispecific antibody constructed by a humanized dimer tag can prolong the blood circulation time of the antibody fragment to improve the therapeutic effect [Citation109].
Modification of immune cells through antibody fragments
The chimeric antigen receptor (CAR) to modify T cells is also one of the main directions of immunotherapy research [Citation110]. ScFv and nanobody are typically used as the extracellular antigen binding domain of CAR-T cells to specifically recognize antigen. In addition to the extracellular antigen binding domain, CAR-T cells also have hinge regions, transmembrane regions, and costimulatory molecules, and sometimes contain genes that enhance T cell function. As an example, Golubovskaya et al. designed CAR-T cells that bind specifically to CD47 [Citation111]. In vitro experiments showed that CD47-CAR-T cells can express CD47-associated cytokines at high levels and have a killing effect on various tumour cells, and intratumoural injection of CD47-CAR-T cells can significantly inhibit tumours in mouse tumour-bearing models growing. The natural resistance of malignant tumours to NK cells can also be overcome by CAR-modified immune cells. An example of this region is that anti-CD19-CAR modified NK-92 cells are effective in eliminating the resistance of B cell malignancies to NK cells and effectively lysing tumour cells [Citation112]. These indicate that CAR to modify immune cells has potential for reversing tumour resistance.
Although antibody fragment targeted therapy has a beneficial therapeutic effect, however, there are still some problems to be solved. Since immunotherapy exerts an anti-cancer effect through the immune system, it is extremely important to select a target, otherwise, it will lead to immune-related adverse reactions [Citation113]. More than just immunotherapy, the choice of targeted antigens for all tumour treatments based on antibody fragments has a crucial impact on therapeutic outcomes. Furthermore, too faint an immunotherapeutic effect may result in an ineffective treatment effect, and an excessive immunotherapy may result in cytokine release storm. Hence, exploring a balance between treatment and side effects is a key issue in immunotherapy. Finding true tumour-specific antigens to replace tumour-associated antigens can fundamentally solve these problems, so the depth of tumour research and the discovery of novel targets are critical for the development of antibody fragments.
Conclusion and perspectives
Targeted therapy is a revolutionary change in cancer treatments. Antibody fragments not only retain antigen-specific binding functions but also remove the drawbacks of mAb with long circulating half-life and poor clinical reproducibility. In addition, antibody fragments have more desirable biochemical properties. In recent years, antibody fragments have achieved encouraging successes in the field of tumour imaging and treatment, and have become a new direction in the development of antibody drugs. There is no doubt that antibody fragments have the great promising drug development platform but still face some unsolved issues to be resolved at this stage. First and foremost, due to the absence of the partial structure, the affinity of antibody fragment for bind to the antigen is reduced and the half-life is too short. On the other hand, when the antibody fragment is used alone, its anti-tumour effect is not obvious due to the lack of the Fc region. Hence, antibody fragments are more suitable for constructing targeted drug delivery systems. The construction of a targeted drug delivery system can take full advantage of the strong penetrability and specificity of antibody fragments while decreasing the effects of structural defects. Even when compared to traditional antibodies that are commonly used in clinical practice, antibody fragments are still in the stage of exploration and development. However, based on the development trend of antibody drugs and the excellent biochemical characteristics of antibody fragments, antibody fragments will become the main measures for cancer treatment and diagnosis in the future.
Disclosure statement
No potential conflict of interest was reported by the authors.
Additional information
Funding
References
- Gao P, Mei C, He L, et al. Designing multifunctional cancer-targeted nanosystem for magnetic resonance molecular imaging-guided theranostics of lung cancer. Drug Deliv. 2018;25:1811–1825.
- Kovacs N, Szigeti K, Hegedus N, et al. Multimodal PET/MRI imaging results enable monitoring the side effects of radiation therapy. Contrast Media Mol Imaging. 2018;2018:5906471.
- Zhou Y, Zhen M, Guan M, et al. Amino acid modified [70] fullerene derivatives with high radical scavenging activity as promising bodyguards for chemotherapy protection. Sci Rep. 2018;8:16573.
- Movahedi MM, Mehdizadeh A, Koosha F, et al. Investigating the photo-thermo-radiosensitization effects of folate-conjugated gold nanorods on KB nasopharyngeal carcinoma cells. Photodiagnosis Photodyn Ther. 2018;24:324–331.
- Roskoski R. Jr. Small molecule inhibitors targeting the EGFR/ErbB family of protein-tyrosine kinases in human cancers. Pharmacol Res. 2018;139:395–411.
- Wang X, Ouyang X, Chen J, et al. Nanoparticulate photosensitizer decorated with hyaluronic acid for photodynamic/photothermal cancer targeting therapy. Nanomedicine (Lond). 2018;14:151–167.
- Warda W, Larosa F, Neto Da Rocha M, et al. CML hematopoietic stem cells expressing IL-1RAP can be targeted by chimeric antigen receptor (CAR)-engineered T cells. Cancer Res. 2018;79:663–675.
- Cai Y, Wang F, Liu Q, et al. A novel humanized anti-PD-1 monoclonal antibody potentiates therapy in oral squamous cell carcinoma. Invest New Drugs. 2018.
- Xu L, Wan C, Du J, et al. Synthesis, characterization, and in vitro evaluation of targeted gold nanoshelled poly(d,l-lactide-co-glycolide) nanoparticles carrying anti p53 antibody as a theranostic agent for ultrasound contrast imaging and photothermal therapy. J Biomater Sci Polym Ed. 2017;28:415–430.
- Koczera P, Appold L, Shi Y, et al. PBCA-based polymeric microbubbles for molecular imaging and drug delivery. J Control Release. 2017;259:128–135.
- Herbst RS, Redman MW, Kim ES, et al. Cetuximab plus carboplatin and paclitaxel with or without bevacizumab versus carboplatin and paclitaxel with or without bevacizumab in advanced NSCLC (SWOG S0819): a randomised, phase 3 study. Lancet Oncol. 2018;19:101–114.
- Tawbi HA, Forsyth PA, Algazi A, et al. Combined nivolumab and ipilimumab in melanoma metastatic to the brain. N Engl J Med. 2018;379:722–730.
- von Minckwitz G, Huang CS, Mano MS, et al. Trastuzumab emtansine for residual invasive HER2-positive breast cancer. N Engl J Med. 2019;380:617–628.
- Khoshtinat Nikkhoi S, Rahbarizadeh F, Ahmadvand D, et al. Multivalent targeting and killing of HER2 overexpressing breast carcinoma cells with methotrexate-encapsulated tetra-specific non-overlapping variable domain heavy chain anti-HER2 antibody-PEG-liposomes: in vitro proof-of-concept. Eur J Pharm Sci. 2018;122:42–50.
- He XX, Du S, Gao SQ, et al. Humanization of fibroblast growth factor 1 single-chain antibody and validation for its antitumorigenic efficacy in breast cancer and glioma cells. J Cell Mol Med. 2018;22:3259–3263.
- Hirasawa S, Kitahara Y, Okamatsu Y, et al. Facile and efficient chemoenzymatic semisynthesis of Fc-fusion compounds for half-life extension of pharmaceutical components. Bioconjug Chem. 2019.
- Shi S, Hong H, Orbay H, et al. ImmunoPET of tissue factor expression in triple-negative breast cancer with a radiolabeled antibody Fab fragment. Eur J Nucl Med Mol Imaging. 2015;42:1295–1303.
- Zhang M, Kobayashi N, Zettlitz KA, et al. Near-infrared dye-labeled anti-prostate stem cell antigen minibody enables real-time fluorescence imaging and targeted surgery in translational mouse models. Clin Cancer Res. 2019;25:188–200.
- Leoh LS, Kim YK, Candelaria PV, et al. Efficacy and mechanism of antitumor activity of an antibody targeting transferrin receptor 1 in mouse models of human multiple myeloma. JI. 2018;200:3485–3494.
- Xin L, Cao J-Q, Liu C, et al. Evaluation of rMETase-loaded stealth PLGA/liposomes modified with anti-CAGE scFV for treatment of gastric carcinoma. J Biomed Nanotechnol. 2015;11:1153–1161.
- Min HS, Kim HJ, Ahn J, et al. Tuned density of anti-tissue factor antibody fragment onto siRNA-loaded polyion complex micelles for optimizing targetability into pancreatic cancer cells. Biomacromolecules. 2018;19:2320–2329.
- Xing J, Lin L, Li J, et al. BiHC, a T-cell-engaging bispecific recombinant antibody, has potent cytotoxic activity against Her2 tumor cells. Transl Oncol. 2017;10:780–785.
- Zhang Y, Guo J, Zhang XL, et al. Antibody fragment-armed mesoporous silica nanoparticles for the targeted delivery of bevacizumab in ovarian cancer cells. Int J Pharm. 2015;496:1026–1033.
- Razzaqi M, Rasaee MJ, Paknejad M. A critical challenge in the development of antibody: selecting the appropriate fragment of the target protein as an antigen based on various epitopes or similar structure. Mol Immunol. 2019;111:128–135.
- Benschop RJ, Chow CK, Tian Y, et al. Development of tibulizumab, a tetravalent bispecific antibody targeting BAFF and IL-17A for the treatment of autoimmune disease. MAbs. 2019;11:1175.
- Zettlitz KA, Tavare R, Tsai WK, et al. 18)F-labeled anti-human CD20 cys-diabody for same-day immunoPET in a model of aggressive B cell lymphoma in human CD20 transgenic mice. Eur J Nucl Med Mol Imaging. 2019;46:489–500.
- Wingler LM, McMahon C, Staus DP, et al. Distinctive activation mechanism for angiotensin receptor revealed by a synthetic nanobody. Cell. 2019;176:479–490.e12.
- Li C, Wang Y, Liu T, et al. An E. coli-produced single-chain variable fragment (scFv) targeting hepatitis B virus surface protein potently inhibited virion secretion. Antiviral Res. 2019;162:118–129.
- Xavier S, Gopi Mohan C, Nair S, et al. Generation of humanized single-chain fragment variable immunotherapeutic against EGFR variant III using baculovirus expression system and in vitro validation. Int J Biol Macromol. 2019;124:17–24.
- Lv J, Zhao R, Wu D, et al. Mesothelin is a target of chimeric antigen receptor T cells for treating gastric cancer. J Hematol Oncol. 2019;12:6380000.
- Groof Twm D, Mashayekhi V, Fan TS, et al. Nanobody-targeted photodynamic therapy selectively kills viral GPCR-expressing glioblastoma cells. Mol Pharm. 2019;16:3145–3156.
- Li T, Vandesquille M, Koukouli F, et al. Camelid single-domain antibodies: a versatile tool for in vivo imaging of extracellular and intracellular brain targets. J Control Release. 2016;243:1–10.
- Vandesquille M, Li T, Po C, et al. Chemically-defined camelid antibody bioconjugate for the magnetic resonance imaging of Alzheimer's disease. MAbs. 2017;9:1016–1027.
- Volz J, Mammadova-Bach E, Gil-Pulido J, et al. Inhibition of platelet GPVI induces intratumor hemorrhage and increases efficacy of chemotherapy in mice. Blood. 2019;133:2696–2706.
- Liu W, Zhao W, Bai X, et al. High antitumor activity of Sortase A-generated anti-CD20 antibody fragment drug conjugates. Eur J Pharm Sci. 2019;134:81–92.
- Beha N, Harder M, Ring S, et al. IL-15-based trifunctional antibody-fusion proteins with costimulatory TNF-superfamily ligands in the single-chain format for cancer immunotherapy. Mol Cancer Ther. 2019;18:1278.
- Lu L, Liu N, Fan K, et al. A tetravalent single chain diabody (CD40/HER2) efficiently inhibits tumor proliferation through recruitment of T cells and anti-HER2 functions. Mol Immunol. 2019;109:149–156.
- Iizuka A, Nonomura C, Ashizawa T, et al. A T-cell-engaging B7-H4/CD3-bispecific Fab-scFv antibody targets human breast cancer. Clin Cancer Res. 2019;25:2925–2934.
- Kapelski S, Cleiren E, Attar RM, et al. Influence of the bispecific antibody IgG subclass on T cell redirection. MAbs. 2019;26:1–13.
- Crawford A, Haber L, Kelly MP, et al. A Mucin 16 bispecific T cell-engaging antibody for the treatment of ovarian cancer. Sci Transl Med. 2019;11:eaau7534.
- Haylock AK, Spiegelberg D, Mortensen AC, et al. Evaluation of a novel type of imaging probe based on a recombinant bivalent mini-antibody construct for detection of CD44v6-expressing squamous cell carcinoma. Int J Oncol. 2016;48:461–470.
- El-Sayed A, Bernhard W, Barreto K, et al. Evaluation of antibody fragment properties for near-infrared fluorescence imaging of HER3-positive cancer xenografts. Theranostics. 2018;8:4856–4869.
- Alric C, Herve-Aubert K, Aubrey N, et al. Targeting HER2-breast tumors with scFv-decorated bimodal nanoprobes. J Nanobiotechnol. 2018;16:18.
- Ueda M, Hisada H, Temma T, et al. Gallium-68-labeled anti-HER2 single-chain Fv fragment: development and in vivo monitoring of HER2 expression. Mol Imaging Biol. 2015;17:102–110.
- Tsai WK, Zettlitz KA, Tavare R, et al. Dual-modality immunoPET/fluorescence imaging of prostate cancer with an anti-PSCA Cys-minibody. Theranostics. 2018;8:5903–5914.
- Boogerd LSF, Boonstra MC, Prevoo H, et al. Fluorescence-guided tumor detection with a novel anti-EpCAM targeted antibody fragment: preclinical validation. Surg Oncol. 2019;28:1–8.
- Knowles SM, Tavare R, Zettlitz KA, et al. Applications of immunoPET: using 124I-anti-PSCA A11 minibody for imaging disease progression and response to therapy in mouse xenograft models of prostate cancer. Clin Cancer Res. 2014;20:6367–6378.
- Zhang M, Kobayashi N, Zettlitz KA, et al. Near-infrared dye-labeled anti-prostate stem cell antigen minibody enables real-time fluorescence imaging and targeted surgery in translational mouse models. Clin Cancer Res. 2019;25:188–200.
- Solomon VR, Gonzalez C, Alizadeh E, et al. Tc(CO)3(+) labeled domain I/II-specific anti-EGFR (scFv)2 antibody fragment for imaging EGFR expression. Eur J Med Chem. 2018;157:437–446.
- Vaidyanathan G, McDougald D, Choi J, et al. Preclinical evaluation of 18F-labeled Anti-HER2 nanobody conjugates for imaging HER2 receptor expression by immuno-PET. J Nucl Med. 2016;57:967–973.
- Gao Y, Hernandez C, Yuan HX, et al. Ultrasound molecular imaging of ovarian cancer with CA-125 targeted nanobubble contrast agents. Nanomedicine. 2017;13:2159–2168.
- Long NE, Sullivan BJ, Ding H, et al. Linker engineering in anti-TAG-72 antibody fragments optimizes biophysical properties, serum half-life, and high-specificity tumor imaging. J Biol Chem. 2018;293:9030–9040.
- Rios X, Compte M, Gomez-Vallejo V, et al. Immuno-PET imaging and pharmacokinetics of an anti-CEA scFv-based trimerbody and its monomeric counterpart in human gastric carcinoma-bearing mice. Mol Pharmaceutics. 2019;16:1025–1035.
- Zhang X, Liu C, Hu F, et al. PET imaging of VCAM-1 expression and monitoring therapy response in tumor with a (68)Ga-labeled single chain variable fragment. Mol Pharmaceutics. 2018;15:609–618.
- Zhou Z, McDougald D, Devoogdt N, et al. Labeling single domain antibody fragments with fluorine-18 using 2,3,5,6-tetrafluorophenyl 6-[(18)F]fluoronicotinate resulting in high tumor-to-kidney ratios. Mol Pharmaceutics. 2019;16:214–226.
- Zettlitz KA, Tsai WK, Knowles SM, et al. [(89)Zr]A2cDb immuno-PET of prostate cancer in a human prostate stem cell antigen knock-in (hPSCA KI) syngeneic model. Mol Imaging Biol. 2019;
- Uehara T, Yokoyama M, Suzuki H, et al. A Gallium-67/68-labeled antibody fragment for immuno-SPECT/PET shows low renal radioactivity without loss of tumor uptake. Clin Cancer Res. 2018;24:3309–3316.
- Chen F, Ma K, Madajewski B, et al. Ultrasmall targeted nanoparticles with engineered antibody fragments for imaging detection of HER2-overexpressing breast cancer. Nat Commun. 2018;9:4141.
- Bauerschlag D, Meinhold-Heerlein I, Maass N, et al. Detection and specific elimination of EGFR(+) ovarian cancer cells using a near infrared photoimmunotheranostic approach. Pharm Res. 2017;34:696–703.
- Yuan X, Yang M, Chen X, et al. Characterization of the first fully human anti-TEM1 scFv in models of solid tumor imaging and immunotoxin-based therapy. Cancer Immunol Immunother. 2017;66:367–378.
- Mazzocco C, Fracasso G, Germain-Genevois C, et al. In vivo imaging of prostate cancer using an anti-PSMA scFv fragment as a probe. Sci Rep. 2016;6:23314.
- Boonstra MC, Tolner B, Schaafsma BE, et al. Preclinical evaluation of a novel CEA-targeting near-infrared fluorescent tracer delineating colorectal and pancreatic tumors. Int J Cancer. 2015;137:1910–1920.
- Sonn GA, Behesnilian AS, Jiang ZK, et al. Fluorescent image-guided surgery with an anti-prostate stem cell antigen (PSCA) diabody enables targeted resection of mouse prostate cancer xenografts in real time. Clin Cancer Res. 2016;22:1403–1412.
- Zettlitz KA, Waldmann CM, Tsai WK, et al. A dual-modality linker enables site-specific conjugation of antibody fragments for (18)F-immunoPET and fluorescence imaging. J Nucl Med. 2019.
- Luo H, Hernandez R, Hong H, et al. Noninvasive brain cancer imaging with a bispecific antibody fragment, generated via click chemistry. Proc Natl Acad Sci USA. 2015;112:12806–12811.
- Guo Y, Wang XY, Chen YL, et al. A light-controllable specific drug delivery nanoplatform for targeted bimodal imaging-guided photothermal/chemo synergistic cancer therapy. Acta Biomater. 2018;80:308–326.
- Luo H, England CG, Goel S, et al. ImmunoPET and near-infrared fluorescence imaging of pancreatic cancer with a dual-labeled bispecific antibody fragment. Mol Pharmaceutics. 2017;14:1646–1655.
- Hernot S, Unnikrishnan S, Du Z, et al. Nanobody-coupled microbubbles as novel molecular tracer. J Control Release. 2012;158:346–353.
- Fan X, Wang L, Guo Y, et al. Ultrasonic nanobubbles carrying anti-PSMA nanobody: construction and application in prostate cancer-targeted imaging. PLoS One. 2015;10:e0127419.
- Kanazaki K, Sano K, Makino A, et al. Development of anti-HER2 fragment antibody conjugated to iron oxide nanoparticles for in vivo HER2-targeted photoacoustic tumor imaging. Nanomedicine. 2015;11:2051–2060.
- Lei G, Xu M, Xu Z, et al. A novel fully human agonistic single chain fragment variable antibody targeting death receptor 5 with potent antitumor activity in vitro and in vivo. Int J Mol Sci. 2017;18:2064.
- Rabenhold M, Steiniger F, Fahr A, et al. Bispecific single-chain diabody-immunoliposomes targeting endoglin (CD105) and fibroblast activation protein (FAP) simultaneously. J Control Release. 2015;201:56–67.
- Yang W, Hu Q, Xu Y, et al. Antibody fragment-conjugated gemcitabine and paclitaxel-based liposome for effective therapeutic efficacy in pancreatic cancer. Mater Sci Eng C Mater Biol Appl. 2018;89:328–335.
- Kim M, Pyo S, Kang CH, et al. Folate receptor 1 (FOLR1) targeted chimeric antigen receptor (CAR) T cells for the treatment of gastric cancer. PLoS One. 2018;13:e0198347.
- Rafiq S, Yeku OO, Jackson HJ, et al. Targeted delivery of a PD-1-blocking scFv by CAR-T cells enhances anti-tumor efficacy in vivo. Nat Biotechnol. 2018;36:847–856.
- Pearce AK, Fuchs AV, Fletcher NL, et al. Targeting nanomedicines to prostate cancer: evaluation of specificity of ligands to two different receptors in vivo. Pharm Res. 2016;33:2388–2399.
- Ji X, Shen Y, Sun H, et al. A novel anti-alpha-fetoprotein single-chain variable fragment displays anti-tumor effects in HepG2 cells as a single agent or in combination with paclitaxel. Tumor Biol. 2016;37:10085–10096.
- Lee D, Kim D, Choi YB, et al. Simultaneous blockade of VEGF and Dll4 by HD105, a bispecific antibody, inhibits tumor progression and angiogenesis. MAbs. 2016;8:892–904.
- Ahn HM, Ryu J, Song JM, et al. Anti-cancer activity of novel TM4SF5-targeting antibodies through TM4SF5 neutralization and immune cell-mediated cytotoxicity. Theranostics. 2017;7:594–613.
- Lu Y, Wang Y, Zhang M, et al. HER2-siRNA delivered by EGFR-specific single chain antibody inhibits NSCLC cell proliferation and tumor growth. Oncotarget. 2016;7:23594–23607.
- Sokolova E, Proshkina G, Kutova O, et al. Recombinant targeted toxin based on HER2-specific DARPin possesses a strong selective cytotoxic effect in vitro and a potent antitumor activity in vivo. J Control Release. 2016;233:48–56.
- Amoury M, Kolberg K, Pham AT, et al. Granzyme B-based cytolytic fusion protein targeting EpCAM specifically kills triple negative breast cancer cells in vitro and inhibits tumor growth in a subcutaneous mouse tumor model. Cancer Lett. 2016;372:201–209.
- Lv X, Zhang J, Xu R, et al. Gigantoxin-4-4D5 scFv is a novel recombinant immunotoxin with specific toxicity against HER2/neu-positive ovarian carcinoma cells. Appl Microbiol Biotechnol. 2016;100:6403–6413.
- Aubrey N, Allard-Vannier E, Martin C, et al. Site-specific conjugation of Auristatins onto engineered scFv using second generation maleimide to target HER2-positive breast cancer in vitro. Bioconjugate Chem. 2018;29:3516–3521.
- Tang J, Howard CB, Mahler SM, et al. Enhanced delivery of siRNA to triple negative breast cancer cells in vitro and in vivo through functionalizing lipid-coated calcium phosphate nanoparticles with dual target ligands. Nanoscale. 2018;10:4258–4266.
- Hou SC, Chen HS, Lin HW, et al. High throughput cytotoxicity screening of anti-HER2 immunotoxins conjugated with antibody fragments from phage-displayed synthetic antibody libraries. Sci Rep. 2016;6:31878.
- Amoury M, Mladenov R, Nachreiner T, et al. A novel approach for targeted elimination of CSPG4-positive triple-negative breast cancer cells using a MAP tau-based fusion protein. Int J Cancer. 2016;139:916–927.
- Dou S, Yang XZ, Xiong MH, et al. ScFv-decorated PEG-PLA-based nanoparticles for enhanced siRNA delivery to Her2(+) breast cancer. Adv Healthcare Mater. 2014;3:1792–1803.
- Su Y, Yu L, Liu N, et al. PSMA specific single chain antibody-mediated targeted knockdown of Notch1 inhibits human prostate cancer cell proliferation and tumor growth. Cancer Lett. 2013;338:282–291.
- Jiang K, Li J, Yin J, et al. Targeted delivery of CXCR4-siRNA by scFv for HER2(+) breast cancer therapy. Biomaterials. 2015;59:77–87.
- Kaplan G, Mazor R, Lee F, et al. Improving the in vivo efficacy of an Anti-Tac (CD25) immunotoxin by pseudomonas exotoxin A domain II engineering. Mol Cancer Ther. 2018;17:1486–1493.
- Geddie ML, Kohli N, Kirpotin DB, et al. Improving the developability of an anti-EphA2 single-chain variable fragment for nanoparticle targeting. MAbs. 2017;9:58–67.
- Li Y, Chen Y, Li J, et al. Co-delivery of microRNA-21 antisense oligonucleotides and gemcitabine using nanomedicine for pancreatic cancer therapy. Cancer Sci. 2017;108:1493–1503.
- Herve-Aubert K, Allard-Vannier E, Joubert N, et al. Impact of site-specific conjugation of ScFv to multifunctional nanomedicines using second generation maleimide. Bioconjugate Chem. 2018;29:1553–1559.
- Niwa T, Kasuya Y, Suzuki Y, et al. Novel immunoliposome technology for enhancing the activity of the agonistic antibody against the tumor necrosis factor receptor superfamily. Mol Pharmaceutics. 2018;15:3729–3740.
- Sarisozen C, Dhokai S, Tsikudo EG, et al. Nanomedicine based curcumin and doxorubicin combination treatment of glioblastoma with scFv-targeted micelles: In vitro evaluation on 2D and 3D tumor models. Eur J Pharm Biopharm. 2016;108:54–67.
- Chang, T M S.ARTIFICIAL CELL evolves into nanomedicine, biotherapeutics, blood substitutes, drug delivery, enzyme/gene therapy, cancer therapy, cell/stem cell therapy, nanoparticles, liposomes, bioencapsulation, replicating synthetic cells, cell encapsulation/scaffold, biosorbent/immunosorbent haemoperfusion/plasmapheresis, regenerative medicine, encapsulated microbe, nanobiotechnology, nanotechnology. Artificial Cells, Nanomedicine, and Biotechnology. 2019;47(1):997–1013. doi:10.1080/21691401.2019.1577885.
- Li T, Amari T, Semba K, et al. Construction and evaluation of pH-sensitive immunoliposomes for enhanced delivery of anticancer drug to ErbB2 over-expressing breast cancer cells. Nanomedicine. 2017;13:1219–1227.
- Bartoschek M, Oskolkov N, Bocci M, et al. Spatially and functionally distinct subclasses of breast cancer-associated fibroblasts revealed by single cell RNA sequencing. Nat Commun. 2018;9:5150.
- Libutti SK, Tamarkin L, Nilubol N. Targeting the invincible barrier for drug delivery in solid cancers: interstitial fluid pressure. Oncotarget. 2018;9:35723–35725.
- Miyazaki T, Ikeda K, Sato W, et al. Extracellular vesicle-mediated EBAG9 transfer from cancer cells to tumor microenvironment promotes immune escape and tumor progression. Oncogenesis. 2018;7:7.
- Riley, R S, June, C H, Langer, R, et al. Delivery technologies for cancer immunotherapy. Nat Rev Drug Discov. 2019;18(3):175–196. doi:10.1038/s41573-018-0006-z.
- Jiang H, Shi Z, Wang P, et al. Claudin18.2-specific chimeric antigen receptor engineered T cells for the treatment of gastric cancer. J Natl Cancer Inst. 2019;111:409–418.
- de Bruin RCG, Veluchamy JP, Lougheed SM, et al. A bispecific nanobody approach to leverage the potent and widely applicable tumor cytolytic capacity of Vgamma9Vdelta2-T cells. Oncoimmunology. 2018;7:e1375641.
- Zhang C, Oberoi P, Oelsner S, et al. Chimeric antigen receptor-engineered NK-92 cells: an off-the-shelf cellular therapeutic for targeted elimination of cancer cells and induction of protective antitumor immunity. Front Immunol. 2017;8:533.
- Harwood SL, Alvarez-Cienfuegos A, Nunez-Prado N, et al. ATTACK, a novel bispecific T cell-recruiting antibody with trivalent EGFR binding and monovalent CD3 binding for cancer immunotherapy. Oncoimmunology. 2018;7:e1377874.
- Chan WK, Kang S, Youssef Y, et al. A CS1-NKG2D bispecific antibody collectively activates cytolytic immune cells against multiple myeloma. Cancer Immunol Res. 2018;6:776–787.
- Satta A, Mezzanzanica D, Caroli F, et al. Design, selection and optimization of an anti-TRAIL-R2/anti-CD3 bispecific antibody able to educate T cells to recognize and destroy cancer cells. MAbs. 2018;10:1084–1097.
- Ahmed M, Cheng M, Cheung IY, et al. Human derived dimerization tag enhances tumor killing potency of a T-cell engaging bispecific antibody. Oncoimmunology. 2015;4:e989776.
- Krenciute G, Krebs S, Torres D, et al. Characterization and functional analysis of scFv-based chimeric antigen receptors to redirect T cells to IL13Ralpha2-positive glioma. Mol Ther. 2016;24:354–363.
- Golubovskaya V, Berahovich R, Zhou H, et al. CD47-CAR-T cells effectively kill target cancer cells and block pancreatic tumor growth. Cancers (Basel). 2017;9:139.
- Romanski A, Uherek C, Bug G, et al. CD19-CAR engineered NK-92 cells are sufficient to overcome NK cell resistance in B-cell malignancies. J Cell Mol Med. 2016;20:1287–1294.
- Rius Ruiz I, Vicario R, Morancho B, et al. p95HER2-T cell bispecific antibody for breast cancer treatment. Sci Transl Med. 2018;10:eaat1445.