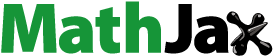
Abstract
Nowadays, the development of drug-loaded electrospun organic-inorganic composite scaffolds for tissue engineering application is an attractive approach. In this study, a composite scaffold of Poly-l-lactic acid (PLLA) incorporated dexamethasone (Dexa) loaded Mesoporous Silica Nanoparticles (MSN) coated with Chitosan (CS) were fabricated by electrospinning for bone tissue engineering application. The MSN was prepared by precipitation method. After that, Dexamethasone (Dexa) was loaded into MSNs (MSN-Dexa). In the following, CS was coated over the prepared nanoparticles to form MSN-Dexa@CS and then, were mixed to PLLA solution to form MSN-Dexa@CS/PLLA composite for electrospinning. The surface morphology, hydrophilicity, tensile strength and the bioactivity of the scaffolds were characterized. The osteogenic proliferation and differentiation potential were evaluated by MTT assay and by measuring the basic osteogenic markers: the activity of the enzyme alkaline phosphatase and the level of calcium deposition. The composite scaffolds prepared here have conductive surface property and have a better osteogenic potential than pure PLLA scaffolds. Hence, the controlled release of nanoparticle containing Dexa from composite scaffold supported the osteogenesis and made the composite scaffolds ideal candidates for bone tissue engineering application and pH-sensitive delivery of drugs at the site of implantation in tissue regeneration.
Graphical Abstract
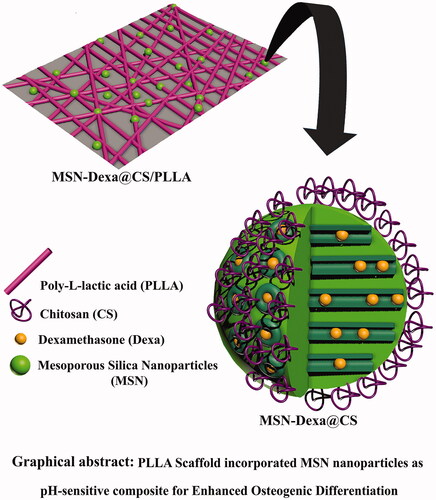
Introduction
Tissue engineering (TE) is a multidisciplinary science which brings the best alternative to conventional therapies to repair damaged tissues [Citation1]. For effective regeneration or repair of the damaged tissue, TE involves combination of various factors to mimic the natural structure of damaged tissue. The three critical components commonly referred to as the “tissue engineering triad”, are scaffolds, cells, and growth factors [Citation1,Citation2]. The scaffold is the key component in TE due to providing structural support for the cells to adhere, grow, spread, proliferate and penetrate moreover the scaffolds can trigger maturation and phenotypic expression in the cells by facilitating diffusion of nutrients and growth factors localized delivery [Citation3]. In addition, it could also be used to deliver drugs that can accelerate the regenerative and healing process [Citation4,Citation5]. Several materials of both natural and synthetic origin have been investigated for fabrication of TE scaffolds, [Citation6] including; metals, ceramics, polymers [Citation3] and composites [Citation7].
Among the synthetic polymers, Poly-l-lactic acid (PLLA) has been widely used for making scaffolds for bone tissue engineering. This is because of its superior mechanical properties, biodegradability, and biocompatibility with high spatial interconnectivity, high porosity, and controlled alignment electrospun 3 D nanofibrous matrices provide cell migration ability [Citation8,Citation9]. Moreover, it is an FDA approved polymer for various biomedical applications including drug delivery and TE [Citation10]. In spite of that; the scaffolds made of pure PLLA, have low biological activity and poor mechanical strength, hampering their applications in bone TE. In addition, it’s in vivo acidic degradation products may cause inflammatory and allergic reactions at the site of implantation [Citation11]. Besides, scaffolds made from polymers are generally flexible, can be easily fabricated to form complex shapes and structures but lack of mechanical strength and bioactive function (e.g. strong bonding to living tissue). While ceramics and glasses are known as too stiff and brittle. Hence, the development of composite materials for TE scaffolds is nowadays an attractive approach. Since scaffold properties can be modified to the particular mechanical and physiologic demands of the host tissue [Citation12,Citation13]. Currently, bioactive calcium phosphate-based biomaterials and bioceramics have been frequently used as a composite to reinforce the biopolymers including PLLA with mechanical property, bioactivity and better osteoconductivity [Citation11].
Targeting drug delivery system and gene therapy have been impressed by the superior attributes of mesoporous silica nanoparticles (MSNs). The conspicuous virtues of MSN including large surface areas, high pore volumes, tunable pore diameters, the surface capability of being modified with various functional groups, and biocompatibility stand out to be a promising candidate for biomedical application [Citation14–16]. Moreover, surface functional groups of MSN can participate in specific chemical reactions such as body fluids to generate nano-sized carbonated apatite in order to ensure the integration of implanted bone by bioactive binding to natural bone [Citation17]. In addition, the type 1 collagen (COL 1) synthesis, expression of osteogenic related genes in osteoblasts and formation of mineralized nodule promoted by silicon ions released from MSNs [Citation18]. The mechanical interlocking between MSNs and a given polymer due to high porosity of MSNs could form strong bond to decrease adhesion problem with other varieties of reinforcement materials. In addition to this, the efficient stress transfer mechanism as a consequence of high surface area nature of MSNs could lead to increasing the strength of MSN/polymer composites [Citation19]. As mentioned above, bone TE has been impressed by the advantages of MSNs as implantable material and stand out to be a promising candidate [Citation18].
The chemical flexibility with the ability to load various classes of drugs highlights MSNs as incredible system for cell-targeted uptake and controlled drug delivery [Citation20–22]. MSNs incorporation in scaffolds can provide refined drug delivery system for targeted and controlled drug delivery system in biological cues. The unique features of MSN such as tunable size and shape, high pore volume and large surface area provide facilities to high drug loading. In addition, MSNs platform flexibility and the possibilities to modify surface functional groups made this kind of platform appropriate for targeted and controlled release of drugs. The advantages of the MSNs surface functionalization can be summarized as follows: circumvention of undesirable biological interaction and promotion desirable one by precise engineering, enhancement of bioavailability and cellular uptake. Apart from these, biomaterial mechanical properties, prolonged and sustained release profile and intracellular gene delivery in bone TE would be improved by MSNs. Altogether these advantages make it possible to customize therapeutic action by targeted and controlled drug delivery and tailored pharmacokinetic and release profile in tissue regeneration [Citation23].
Dexamethasone (Dexa) induces osteoblast differentiation in typical time and dose-dependent manners [Citation24]. More importantly, Dexa exerts its osteoactivity via binding to the glucocorticoid receptor within the cellular cytosol [Citation25]. Therefore, to promote osteoblast differentiation efficacy, Dexa must be transported intracellularly and then release in a controlled manner. However, the “premature release” often limits the efficacy and undesirably increases the dosage of Dexa. Simultaneously transport Dexa into the cell and further release it in the cytosol [Citation26,Citation27].
Chitosan (CS), with a strong response in near-neutral or slightly acidic pH ranges, has been used utilized as a good candidate for pH-sensitive MSNs-based drug delivery [Citation28,Citation29]. In addition, CS can stimulate osteoconductivity and enhance mineralization [Citation30]. Therefore, we hypothesize that CS coated MSNs (MSN@CS) may be a promising nanocarrier for targeted delivery of Dexa. Based on the above rationale in this work, MSN-Dexa@CS/PLLA composite electrospun scaffolds were fabricated to enhance bone regeneration. We first loaded Dexa into the MSNs (MSN-Dexa) and then, the CS was coated on the surface of MSNs (MSN-Dexa@CS) and finally, mixed with PLLA solution to form MSN-Dexa@CS/PLLA composite for electrospinning. In this ensemble, CS physically capped onto the outlet of the MSNs was as a “gate-like” ensemble to achieve pH-sensitive release of loaded Dexa within the cell. After being endocytosed into cells, with the decreasing of the pH value and the changing of dispersing states of the CS, the loaded Dexa will then release into the cytosol [Citation31]. Consequently, Dexa exerted osteoblast differentiation effects [Citation31].
Material and methods
Materials
Cetyltrimethylammonium bromide (CTAB), Tetraethyl orthosilicate (TEOS), Mesitylene and all other solvents and reagents were purchased from Merck (Darmstadt, Germany). Dexamethasone and dimethyl sulfoxide (DMSO) were from Merck (Darmstadt, Germany). MTT solution and Ascorbic acid 2-phosphate and β-glycerophosphate were purchased from Sigma-Aldrich, St. Louis, MO.
Preparation of MSN
MSNs were prepared in the presence of mesitylene based on previous procedures [Citation32,Citation33]. Briefly, 7.0 ml of mesitylene, as a swelling agent of micelles, and 1.0 g of CTAB were added to a solution containing 480 ml of deionized water and 3.5 ml of NaOH (2 M). The solution was stirred vigorously at 80 °C for 4 h and then 5.0 ml of TEOS was quickly added into the solution. After vigorous stirring at 80 °C for another 2 h, the prepared white precipitate was collected by vacuum filtrations and washed several times with ethanol. In order to form MSN as a white powder, the washed precipitation dried overnight under vacuum at 45 °C. The template CTAB (surfactant) in MSN was removed by heating the powder at 550 °C in air for 3 h. In order to verify the successful calcination of MSN, IR spectroscopy was used to reveal presence of any residual organic species.
Dexa loading into MSN
Dexa was loaded into the MSN according to reported procedures with some modifications [Citation33,Citation34]. Briefly, 50 mg MSN was added into 5 ml Dexa solution in distilled water at a concentration of 2 mg/ml. In order to obtain well-dispersed suspension, prepared mixture was ultrasonically dispersed for 5 min by bath sonicator and stirred at room temperature for 48 h. Afterward, the dispersion was centrifuged to collect Dexa loaded nanoparticles and then washed with deionized water. In order to calculate Dexa loading efficiency (DLE) and Dexa loading capacity (DLC), the supernatant was preserved and residual Dexa in supernatant was analyzed through plotting calibration curve of Dexa standard solutions by UV-visible spectroscopy at the wavelength of 243 nm. The Dexa loading efficiency and capacity were evaluated using following formulas:
Preparation of CS coated MSNs
We used a reported procedure with some modification to coat CS on the surface of MSN [Citation35] as follows; 125 mg CS was dispersed in 25 ml acetic acid (3%) and stirred for 24 h to obtain a solution of CS (0.5% w/v). 50 mg of Dexa loaded MSN was added in 25 ml ethanol, the mixture was dispersed for 5 min and then the pH was adjusted to 3.5–4.5 by addition of acetic acid. Consequently, 5 ml of the CS solution was added drop wisely to the above mixture of Dexa loaded MSN and stirred at room temperature for 24 h. Finally, the mixture was centrifuged at 10,000 rpm to collect CS coated MSN, washed twice with deionized water and excess ethanol, and dried overnight under vacuum at room temperature.
Electrospinning fabrication of different scaffolds
PLLA scaffolds were prepared by dissolving 0.43 g of PLLA in 6 ml of chloroform and continuously stirred under magnetic stirrer at 200 rpm until all the PLLA was completely dissolved. For composite scaffolds, 5% w/v of CS coated MSN (Dexa loaded and unloaded into two separated part) was first dissolved in 1 ml of DMF by sonication for 2 min and then added to the above PLLA solution under stirring till the bubble-free transparent solution was formed. The solution was then transferred to two 5 cc syringe with needle to automatic syringe pump. One-step two nozzles electrospinning was used to fabricate the scaffolds. The Nano Model (Tehran, Iran) with two nozzles was used to setup electrospinning experimental. The voltage applied at the tip of the needle was 20 kV. The mass flow rates were 0.5 ml/h, and distance between the tip of the needle and the collector was maintained at 15 cm. The speed of the rotary collector was 500 rpm and scanning distance was 10 cm. The nanofibrous scaffolds were air plasma treated with Diener electronic plasma cleaner (Germany).
Water contact assay
It was evaluated by using sessile drop water contact angle measurement (OCA 15 plus) Surface Analysis system. A drop of water was placed on the nanofibrous scaffolds and after 10 s the contact angle was measured at room temperature.
Tensile strength assay
In order to measure the nanofibrous scaffolds’ tensile strength, the specimens of different kind of scaffolds with 30 mm gauge length and 5 mm diameter were held on the surfaces by clips and placed into the tensile tester. The tensile measurement was done at 50 mm/min crushing speed.
Isolation and expansion of human adipose tissue-derived mesenchymal stem cell (MSCs)
Isolation of MSCs from human adipose tissue was achieved according to the previous protocol [Citation36]. The adipose tissue was taken from cosmetic liposuction of 10 different donors (age 24–40, Shariati, Hospital, Tehran, Iran) after the full consent of each person according to the guidelines of Iran’s Ministry of Health Medical Ethics Committee. The adipose tissue was then treated with collagenase 1% and trypsin 25% (GIBCO) with shaking at 37 °C in an incubator for 30 min and then centrifuged, and the cell pellet was resuspended in Dulbecco’s Modified Eagle’s Medium (DMEM) containing 10% (v/v) fetal bovine serum (FBS), transferred to the flasks and put in to an incubator until reaching 80% confluence, the cell was subcultured till passage number five.
Cell seeding
To seed cell, the scaffolds were cut in to 1.5 diameter circular pieces, put in to 24-well tissue culture plate (TCP), sterilized with 70% ethanol, washed with phosphate-buffered saline (PBS, pH 7.4) twice and finally, incubated with basal medium (DMEM supplemented with 10% FBS) including antibiotics. An initial density of 5 × 103 cells was seeded on each scaffold for assessment of cell attachment and proliferation. For osteogenic differentiation evaluations, basal medium was supplemented with 100 nM Dexa, 0.2 mM ascorbic acid 2-phosphate and 10 mM β-glycerophosphate and placed on the cell-loaded scaffolds after seeding an initial density of 104 cells followed by reaching 80% confluence. Dexa was excluded from the osteogenic media for Dexa loaded scaffold.
Cell proliferation
The affinity of the cell for the new nanofiber scaffold which is defined with adhesion and proliferation of cultured stem cells on the scaffold was investigated through MTT assay. For this purpose, the incubated cell-loaded scaffolds were refreshed with DMEM including 10% MTT solution after 24 h for cell adhesion and on days 1, 4, and 7 for cell proliferation assay and incubated again for 3–4 h in order to oxidize this substrate in the living cells by mitochondrial dehydrogenases. Then the supernatant was replaced with DMSO as the solvent of the dark blue formazan crystals formed in the live cells and finally the absorbance of purple solution was measured via spectrophotometer (Bioteck, Winooski, VT) at 570 nm.
Alkaline phosphatase activity (ALP)
ALP activity is an early marker of osteogenic differentiation of human MSCs. ALP activity was determined by a colorimetric method using Phosphatase Substrate Kit which contains P-Nitro Phenyl Phosphate (PNPP) as a substrate. Briefly, 250 ml Radioimmunoprecipitation assay buffer (RIPA buffer) and protease inhibitor were used in order to extract the total protein of cells and prevent the extracted proteins from degradation. The lysate was shaken at 4 °C and centrifuged at 15,000 rpm for 15 min to sediment cell debris. Finally the supernatant was collected and the ALP assay kit (Parsazmoon, Tehran, Iran) was used to measure the activity of ALP. The activity of enzyme (IU/L) was normalized against total protein (mg/dl).
Calcium content assay
To determine the extent of mineralization: first, the cell loaded scaffolds were homogenized in 0.6 N HCl and then shaken at 4 °C for 1 h in order to extract the calcium deposited. Finally, the calcium content was calculated through a calcium content assay kit (Parsazmoon, Tehran, Iran) and cresolphthalein complexone interaction. Furthermore, the standard curve of OD versus concentration was estimated by using a serial dilution of standard solution in the kit.
Statistical analysis
All experiments were conducted at least three times, and the values were reported as the mean ± standard deviation. Statistical analysis was conducted by two-way analysis of variance (two-way ANOVA), a value of p < .05 was considered as statistically significant.
Characterization
Fourier transform infrared spectroscopy (FTIR) were taken by FT-IR Magna 550, Nicolet using the KBr plates to investigate functional groups of nanoparticles. Powder XRD patterns were collected on STOE Theta-theta Powder Diffraction System, Radiation: 1.54060 Cu, Generator: 40 kV, 40 mA. UV–visible spectra results were obtained using UV–visible Jasco-530 spectrophotometer and via spectrophotometer (Bioteck, Winooski, VT). The morphology of the samples was investigated by transmission electron microscope (HRTEM, Philips CM30, 300 kV, Netherlands) and field emission scanning electron microscope (FE-SEM, Tescan/Mira, Czech Republic). Scanning electron microscope (SEM) was used to determine the surface morphology of the nanofibrous scaffolds. Thermogravimetric analysis (TGA) were taken by Thermogravimetric analyzer METTLER TOLEDO from room temperature to 800 °C under nitrogen flow to investigate thermal decomposition experiments. The mechanical strength of the nanofibrous scaffolds was assessed by a tensile testing machine (INSTRON-5566, Elancourt, France). Nano Model (Tehran, Iran) with two nozzles was used to electrospinning experimental setup. Optical contact angle measuring instrument OCA 15 plus model, was used to water contact assay.
Results and discussion
This study mainly focused on enhanced Osteogenic Differentiation of Human Adipose Tissue Stem Cells by pH-sensitive delivery of dexamethasone and investigation of Poly-l-lactic acid Scaffold incorporated Chitosan coated Mesoporous Silica Nanoparticles as pH-sensitive composite on the ability of the nanofiber scaffolds to support and facilitate osteogenic differentiation of MSCs. Scheme 1 shows schematic representation for the Poly-l-lactic acid Scaffold incorporated Dexa loaded Chitosan coated Mesoporous Silica Nanoparticles.
Characterization of MSNs
FT-IR analysis
As shown in , FT-IR spectra of MSN showed absorption peak at around 1060 cm−1 is attributed to Si–O–Si (νSi–O–Si) bond and absorption peaks around 963 cm−1 and 800 cm−1 are attributed to the stretching vibration of Si–OH (νSi–OH) and Si–O (νSi–O), respectively. The peak appeared at around 2900 cm−1 is relating to the C–H stretching vibrations (νCH) which disappeared after calcination of MSN. The peak appeared in the spectrum of CS coated MSNs at around 2900 cm−1 is attributed to C–H stretching vibrations (νCH) and could be ascribed to CS coating layer on the surface of CS coated MSN. In the spectrum of chitosan, the band appeared at around 2900 cm−1 is attributed to the stretching vibration of C–H and all other bands are the same with the FTIR spectrum of CS [Citation31].
Morphological analysis of MSN
The Morphological analysis of MSN and MSN@CS are shown in Spherical shape and proper size distribution of prepared MSN could be seen clearly. Presence of the CS layer on the surface of MSN has been shown in . Classified pores and hexagonal array MSN networks could be seen in the TEM image (. As shown in , CS layer around the MSN core could be perceived.
XRD analysis
The XRD pattern of MSN and MSN@CS after remove template confirmed the crystalline structure of MSN and MSN@CS. As shown in XRD pattern (), the presence of CS on the surface of MSN could preserve a slight decrease in the intensity in comparison with MSN. The results are in accordance with published reports [Citation29,Citation31,Citation33] and confirmed the formation of hexagonal structure of MSN.
Thermogravimetric analysis (TGA)
In order to gain some structural information about surface modification and determination of percentage of CS coated layer on the surface of MSNs, TGA analysis under a constant N2 flow was performed. As shown in , two-step of losing weight have been seen over the thermogram of CS coated MSN. First one in the range of 25–150 °C indicating the evaporation of residual solvent and adsorbed water that led to a weight loss percentage of about 15% and the another one in the range of 150–500 °C could be assigned to the removal of CS coating layer on the surface of MSNs that led to a total weight loss percentage of about 25%.
Dexa loading into MSN
In order to investigate Dexa loading efficiency (DLE) and DOX loading capacity (DLC) as two more important parameters of capability of the carrier. UV-visible spectroscopy was used to calculate amount of Dexa loaded in MSN pores by using Standard calibration curve of Dexa in DI water (. The drug loading in MSN is directly influenced by the pore size [Citation37]. The calculated result showed 2.4 and 14.4% for DLC and DLE, respectively.
Morphological analysis of scaffolds
As shown in both PLLA and MSN-Dexa@CS/PLLA scaffolds are porous, with interconnected pore between the nanofibers. The image analysis of the nanofibers by Image J software showed a mean diameter of 565 ± 353 nm for PLLA and 783 ± 342 nm for MSN-Dexa@CS/PLLA scaffolds. This shows that the incorporation of the MSN-Dexa@CS/PLLA to PLLA significantly changed the fiber diameter of the nanofibers. However, it couldn’t bring any significant change in the porosity and pore size of the scaffold. The change in fiber diameter was attributed to change in solution property of the electrospun solution after the addition of MSN-Dexa@CS/PLLA, which increases the viscosity and decreased the electric conductivity of the solution [Citation38].
Tensile strength
The tensile strength of a nanofibrous scaffold is one of the most common parameters for evaluation of scaffolds used in tissue engineering. Since the scaffolds need to resist stress imposed during in vitro cell culture and in vivo implantation [Citation39]. As shown in , Pure PLLA scaffolds here have a tensile stress of 2.8 ± 0.2 MPa and a breaking tensile strain of 193%, which has been changed to 5.2 ± 0.2 MPa and 83% in MSN-Dexa@CS/PLLA. Hence, addition of MSN-Dexa@CS significantly increased the mechanical strength of pure PLLA scaffolds.
Surface hydrophilicity
The water contact angle measurement is the method used to determine the surface hydrophilicity of the nanofibrous scaffolds. In this work, to improve the surface hydrophilic the fabricated scaffolds were air plasma treated. The water contact angle of the scaffolds was changed from 130 ± 1.8 to 42.0 ± 2.2° for PLLA scaffolds and to 24 ± 1.6° after the addition of MSN-Dexa@CS. Hence, addition of MSN-Dexa@CS changed the surface hydrophilicity of pure PLLA scaffolds. The surface wetting of scaffolds used in TE plays key role in determining the overall performance of their applications; because, it affects the cell attachment and proliferation to great extent. The water contact angle of the surface depends upon the topographic configurations and chemical composition of the scaffolds [Citation40]. Hence, the presence of MSN-Dexa@CS had a great impact on the hydrophilicity of the scaffolds.
Cell attachment and proliferation
The affinity of the cell for the fabricated scaffolds; which is defined as adhesion and proliferation of cultured stem cells was examined using MTT assay. As shown in , the cell attachment and proliferation was performed for 7 days: at day 1 for cell attachment and at day 1, day 4 and day 7 for cell proliferation. The results demonstrated that all scaffolds were conductive and biocompatible. Moreover, similar trend of cell growth was observed in all the tested dates for all the scaffolds: cell number gradually increased with the culture period from 1 to 7 days for all the scaffolds. However, MSN@CS/PLLA composite scaffolds showed significantly the largest number of cells than PLLA and MSN-Dexa@CS/PLLA scaffolds (p < .05). But, there was no significant change in cells population grown between MSN@CS/PLLA and MSN-Dexa@CS/PLLA scaffolds in all the tested dates (p > .05).
Osteogenic differentiation
The ability of the nanofiber scaffolds (PLLA, MSN@CS/PLLA and MSN-Dexa@CS/PLLA) to support and facilitate osteogenic differentiation of MSC was investigated by measuring the quantity of bone-related markers such as alkaline phosphatase activity and calcium deposition (mineralization). Herein, the effect of nanoparticle containing Dexa released from the composite scaffold (MSN-Dexa@CS/PLLA) on MSCs after 7, 14 and 21-day cultures was investigated (), and the results were compared with pure PLLA scaffolds and composite scaffolds without Dexa (MSN@CS/PLLA). ALP activity is an early marker of osteogenic differentiation of human MSCs. It increases but then decreases when the culture becomes well-mineralized. ALP activity of MSCs cultured in osteogenic conditions usually peaks after 7–14 days, depending on cell sources and culture conditions [Citation41]. ALP activity of adipose tissue-derived MSCs seeded on the scaffolds and TCP were conducted for 21 days (at the 7th, 14th and 21st day of culture). As shown in an increase in ALP activity was observed for cells cultured on the TCP and the 3 scaffolds (PLLA, MSN@CS/PLLA and MSN-Dexa@CS/PLLA) and from day 7 till day 14. But, then decrease at the 21st date of study.
The level of calcium deposition was measured for 21 days to further evaluate the osteogenic differentiation on the different scaffolds (PLLA, MSN@CS/PLLA and MSN-Dexa@CS/PLLA). As shown in , a similar trend of increase in calcium level deposition was observed from day 7 to day 14 and then day 21. On day 7 and 14, the level of calcium deposition in all the scaffolds was significantly greater than that of TCP. However, on the 21st day, MSN@CS/PLLA and MSN-Dexa@CS/PLLA scaffolds showed a comparable level of calcium deposition with TCP; but, significantly greater than pure PLLA scaffolds (p < .05). Hence, the composite scaffolds exhibited statistically significant (p < .05) level of calcium deposition which strongly supported the osteogenic differentiation of MSCs, which also indicated that there was a controlled release of nanoparticle containing Dexa from the composite scaffolds that revealed enhanced osteoblast differentiation capacity of the targeted delivery system. For the regeneration of bone, bioactive inorganic phases have been attractive through providing chemical and/or physical bone bioactive cues to the cells involved in osteogenic processes. However, Inorganic nanofibers have poor mechanical stability needing significant improvement to be effectively used as cell supporting matrices. On the other hand, polymeric nanofibers provide adequate structural support, but their surface needs to be improved to favor biological reactions, such as cellular recognition and osteogenesis [Citation31]. The addition of chitosan-coated MSN significantly changed the physicochemical and mechanical properties of PLLA in a way that brings favorable interfaces for MSCs proliferation and osteogenic differentiation. This was mainly possible due to the presence of a bunch of highly negatively charged silanol groups introduced on the surface, which strongly attract calcium ions followed by subsequent deposition of phosphate ions to form calcium phosphate minerals. Not only the chemical charge effect but also the highly enhanced surface area due to mesoporosity can account for the substantially enhanced surface bone bioactivity of the nanofibers. While the composite scaffolds played an effective role as a matrix for stem cell behavior toward bone, they also showed additional important performance in targeted drug delivering at the implantation site [Citation42]. According to the previous studies, this good MSCs proliferation and enhanced in vitro osteoinductivity of the composite scaffolds may also be related to the natural properties of CS [Citation31].
Conclusion
In this study, we successfully fabricated a composite nanofibrous scaffold of MSN-Dexa@CS/PLLA for pH-sensitive delivery of Dexa to enhance bone regeneration. The fabricated scaffolds have appropriate surface characteristics and mechanical properties with enhanced MSCs proliferation and osteogenic differentiation compared with pure PLLA nanofibrous scaffolds. Hence, this scaffolds can be used simultaneously for targeted drug delivery in bone tissue engineering application. However, further in vivo study is required to demonstrate their application in the ideal condition.
fiber_diameter_of_MSN-Dexa_CS-PLLA__b_.xls
Download ()fiber_diameter_of_pure_PLLA__a_.xls
Download ()Acknowledgements
This work was supported by the Tehran University of Medical Sciences, Tehran, Iran.
Disclosure statement
No potential conflict of interest was reported by the authors.
References
- Chan B, Leong K. Scaffolding in tissue engineering: general approaches and tissue-specific considerations. Eur Spine J. 2008;17:467–479.
- Shadjou N, Hasanzadeh M. Bone tissue engineering using silica-based mesoporous nanobiomaterials: recent progress. Mater Sci Eng: C. 2015;55:401–409.
- Ravichandran R, Sundaramurthi D, Gandhi S, et al. Bioinspired hybrid mesoporous silica–gelatin sandwich construct for bone tissue engineering. Micropor Mesopor Mater. 2014;187:53–62.
- Lee S-H, Shin H. Matrices and scaffolds for delivery of bioactive molecules in bone and cartilage tissue engineering. Adv Drug Deliv Rev. 2007;59:339–359.
- Motamedian SR, Hosseinpour S, Ahsaie MG, et al. Smart scaffolds in bone tissue engineering: A systematic review of literature. WJSC. 2015;7:657.
- Ravichandran R, Gandhi S, Sundaramurthi D, et al. Hierarchical mesoporous silica nanofibers as multifunctional scaffolds for bone tissue regeneration. J Biomater Sci, Polymer Ed. 2013;24:1988–2005.
- Mieszawska AJ, Fourligas N, Georgakoudi I, et al. Osteoinductive silk–silica composite biomaterials for bone regeneration. Biomaterials. 2010;31:8902–8910.
- Khajavi R, Abbasipour M, Bahador A. Electrospun biodegradable nanofibers scaffolds for bone tissue engineering. J Appl Polym Sci. 2016;133:42883.
- Ahmadi M, Seyedjafari E, Zargar SJ, et al. Osteogenic differentiation of mesenchymal stem cells cultured on PLLA scaffold coated with Wharton's Jelly. EXCLI J. 2017;16:785.
- Birhanu G, Akbari Javar H, Seyedjafari E, et al. An improved surface for enhanced stem cell proliferation and osteogenic differentiation using electrospun composite PLLA/P123 scaffold. Artif Cells Nanomed Biotechnol. 2018;46:1274–1281.
- Xie L, Yu H, Yang W, et al. Preparation, in vitro degradability, cytotoxicity, and in vivo biocompatibility of porous hydroxyapatite whisker-reinforced poly (L-lactide) biocomposite scaffolds. J Biomater Sci, Polymer Ed. 2016;27:505–528.
- Sowjanya J, Singh J, Mohita T, et al. Biocomposite scaffolds containing chitosan/alginate/nano-silica for bone tissue engineering. Coll Surf B Biointerf. 2013;109:294–300.
- Lou T, Wang X, Song G, et al. Fabrication of PLLA/β-TCP nanocomposite scaffolds with hierarchical porosity for bone tissue engineering. Int J Biol Macromol. 2014;69:464–470.
- Feng W, Zhou X, He C, et al. Polyelectrolyte multilayer functionalized mesoporous silica nanoparticles for pH-responsive drug delivery: layer thickness-dependent release profiles and biocompatibility. J Mater Chem B. 2013;1:5886–5898.
- Feng W, Nie W, He C, et al. Effect of pH-responsive alginate/chitosan multilayers coating on delivery efficiency, cellular uptake and biodistribution of mesoporous silica nanoparticles based nanocarriers. ACS Appl Mater Interf. 2014;6:8447–8460.
- Zhou X, Feng W, Qiu K, et al. BMP-2 derived peptide and dexamethasone incorporated mesoporous silica nanoparticles for enhanced osteogenic differentiation of bone mesenchymal stem cells. ACS Appl Mater Interfaces. 2015;7:15777–15789.
- Wang Y, Zhao Q, Han N, et al. Mesoporous silica nanoparticles in drug delivery and biomedical applications. Nanomed: Nanotechnol, Biol Med. 2015;11:313–327.
- Luo Z, Deng Y, Zhang R, et al. Peptide-laden mesoporous silica nanoparticles with promoted bioactivity and osteo-differentiation ability for bone tissue engineering. Coll Surf B: Biointerf. 2015;131:73–82.
- Li K, Sun H, Sui H, et al. Composite mesoporous silica nanoparticle/chitosan nanofibers for bone tissue engineering. RSC Adv. 2015;5:17541–17549.
- Rosenholm JM, Mamaeva V, Sahlgren C, et al. Nanoparticles in targeted cancer therapy: mesoporous silica nanoparticles entering preclinical development stage. Nanomedicine. 2012;7:111–120.
- Mamaeva V, Sahlgren C, Lindén M. Mesoporous silica nanoparticles in medicine—Recent advances. Adv Drug Deliv Rev. 2013;65:689–702.
- Perez R, El-Fiqi A, Park J-H, et al. Therapeutic bioactive microcarriers: co-delivery of growth factors and stem cells for bone tissue engineering. Acta biomaterialia. 2014;10:520–530.
- Rosenholm JM, Zhang J, Linden M, et al. Mesoporous silica nanoparticles in tissue engineering–a perspective. Nanomedicine. 2016;11:391–402.
- Mikami Y, Omoteyama K, Kato S, et al. Inductive effects of dexamethasone on the mineralization and the osteoblastic gene expressions in mature osteoblast-like ROS17/2.8 cells. Biochem Biophys Res Commun. 2007;362:368–373.
- Santo VE, Gomes ME, Mano JF, et al. Controlled release strategies for bone, cartilage, and osteochondral engineering—part I: recapitulation of native tissue healing and variables for the design of delivery systems. Tiss Eng Part B: Rev. 2013;19:308–326.
- Du L, Song H, Liao S. A biocompatible drug delivery nanovalve system on the surface of mesoporous nanoparticles. Micropor Mesopor Mater. 2012;147:200–204.
- Tang Y, Teng Z, Liu Y, et al. Cytochrome C capped mesoporous silica nanocarriers for pH-sensitive and sustained drug release. J Mater Chem B. 2014;2:4356–4362.
- Chen Z, Li X, He H, et al. Mesoporous silica nanoparticles with manipulated microstructures for drug delivery. Coll Surf B Biointerf. 2012;95:274–278.
- Popat A, Liu J, Lu GQM, et al. A pH-responsive drug delivery system based on chitosan coated mesoporous silica nanoparticles. J Mater Chem. 2012;22:11173–11178.
- Zhao L, Burguera EF, Xu HH, et al. Fatigue and human umbilical cord stem cell seeding characteristics of calcium phosphate–chitosan–biodegradable fiber scaffolds. Biomaterials. 2010;31:840–847.
- Gan Q, Zhu J, Yuan Y, et al. A dual-delivery system of pH-responsive chitosan-functionalized mesoporous silica nanoparticles bearing BMP-2 and dexamethasone for enhanced bone regeneration. J Mater Chem B. 2015;3:2056–2066.
- Zhang J, Zhao S, Zhu Y, et al. Three-dimensional printing of strontium-containing mesoporous bioactive glass scaffolds for bone regeneration. Acta biomaterialia. 2014;10:2269–2281.
- Daryasari MP, Akhgar MR, Mamashli F, et al. Chitosan-folate coated mesoporous silica nanoparticles as a smart and pH-sensitive system for curcumin delivery. Rsc Adv. 2016;6:105578–105588.
- Shen J, He Q, Gao Y, et al. Mesoporous silica nanoparticles loading doxorubicin reverse multidrug resistance: performance and mechanism. Nanoscale. 2011;3:4314–4322.
- Gulfam M, Chung BG. Development of pH-responsive chitosan-coated mesoporous silica nanoparticles. Macromol Res. 2014;22:412–417.
- Ramezanifard R, Seyedjafari E, Ardeshirylajimi A, et al. Biomimetic scaffolds containing nanofibers coated with willemite nanoparticles for improvement of stem cell osteogenesis. Mater Sci Eng: C. 2016;62:398–406.
- Szegedi A, Popova M, Goshev I, et al. Controlled drug release on amine functionalized spherical MCM-41. J Solid State Chem. 2012;194:257–263.
- Rungswang W, Kotaki M, Shimojima T, et al. Role of surfactant on inducing specific microdomains of block copolymer: an example case from polystyrene-b-poly (ethylene-co-1-butene)-b-polystyrene (SEBS) electrospun thermoplastic-elastomer fiber containing polyethylene glycol lauryl ether (PGLE). Polymer. 2014;55:2068–2076.
- Shao J, Chen C, Wang Y, et al. Structure and surface nanomechanics of poly (l-lactide) from thermally induced phase separation process. Appl Surf Sci. 2012;258:6665–6671.
- de Luca AC, Terenghi G, Downes S. Chemical surface modification of poly‐ε‐caprolactone improves Schwann cell proliferation for peripheral nerve repair. J Tissue Eng Regen Med. 2014;8:153–163.
- Declercq H, Van den Vreken N, De Maeyer E, et al. Isolation, proliferation and differentiation of osteoblastic cells to study cell/biomaterial interactions: comparison of different isolation techniques and source. Biomaterials. 2004;25:757–768.
- Singh RK, Jin G-Z, Mahapatra C, et al. Mesoporous silica-layered biopolymer hybrid nanofibrous scaffold: a novel nanobiomatrix platform for therapeutics delivery and bone regeneration. ACS Appl Mater Interfaces. 2015;7:8088–8098.