Abstract
Polycystic ovary syndrome (PCOS) is a heterogeneous reproductive disease. Adipose mesenchymal stem cells (AMSCs) can produce a mass of exosomes. The objective of this study was to determine the effects of exosomal miR-323-3p on cumulus cells (CCs) of PCOS patients. Exosomal miR-323-3p were collected from modified AMSCs. Real-time PCR, western blots, MTT assays, flow cytometry, luciferase reporter assays and a letrozole-induced PCOS mouse model were used to identify mechanisms of exosomal miR-323-3p on CCs. The results revealed that miR-323-3p expression was upregulated in AMSCs, exosomes and CCs. Upregulated miR-323-3p promoted cell proliferation and suppressed apoptosis in CCs, while miR-323-3p inhibitor exerted opposite roles in exosome-treated CCs. Moreover, PDCD4 was upregulated in PCOS CCs, displayed an inverse expression pattern to those of miR-323-3p, and was a direct target of miR-323-3p. Overexpression of PDCD4 reversed the effects of upregulated miR-323-3p on CCs. Serum FSH, LH and testosterone were upregulated while E2 levels were downregulated in the PCOS mice. Upregulation of miR-323-3p alleviated PCOS by suppressing CCs’ apoptosis through targeting PDCD4 in vivo. The results demonstrated that exosomal miR-323-3p promoted cell proliferation and inhibited apoptosis in CCs through targeting PDCD4 in PCOS. This study provides insight into developing new therapeutic strategies for PCOS.
Introduction
Polycystic ovary syndrome (PCOS) is a common reproductive disorder among female individuals at reproductive age with an estimated incidence of 7–9% [Citation1]. Due to its heterogeneous features, PCOS is classified into multiple phenotypes based on the absence or presence of clinical characteristics, such as ovulatory dysfunction, polycystic ovarian morphology and hyperandrogenism [Citation1,Citation2]. Moreover, PCOS is related to metabolic abnormalities including hyperinsulinemia, insulin resistance, obesity and hyperandrogenism [Citation3,Citation4]. Other obstetrical complications are also reported to be linked with PCOS, such as type 2 diabetes mellitus (T2DM), hypertension and cardiovascular diseases [Citation5]. The pathological mechanisms underlying PCOS are complicated since the clinical features are intertwined and the aetiologies vary among individuals [Citation1]. To date, many influential factors including genetics, chronic inflammation, oxidative stress, lifestyle, as well as, environmental pollution have been demonstrated to be associated with PCOS occurrence and progression [Citation6].
It has been reported that the oocyte and surrounding cumulus cells (CCs) display an interdependent relationship in the early stage of follicle development [Citation7], of which CCs are mainly responsible for secreting growth hormones and ovary steroid hormones [Citation8], suggesting that CCs play important roles in oocyte development. On the other hand, atresia is initiated by the oocyte dysfunction caused by abnormal cell proliferation or apoptosis of CCs [Citation9], which is consistent with findings that aberrant cell activities of CCs are associated with failure in follicle maturation, anovulation and infertility that are also symptoms found in PCOS patients [Citation9,Citation10]. Therefore, exploring the mechanisms underlying CCs dysfunction, especially apoptosis, would have great clinical and scientific values.
MicroRNAs (miRNAs), 21–24 nucleotides in length, are a cluster of non-coding RNAs that are important for regulating gene expression at the post-transcriptional level through binding to 3’UTR of target miRNAs, thereby silencing mRNAs [Citation11]. Thanks to their widespread roles in gene expression, it is well documented that miRNAs play essential roles in the regulation of various physiological and pathological processes, for example, apoptosis, cell proliferation and tumorigenesis [Citation11]. In recent years, miRNAs have been reported to be involved in the aberrant follicular function and development in patients with PCOS. Sirotkin et al. reported that human granulosa cells (GCs) transfection with 11 miRNAs show an enhanced apoptosis level and apoptotic protein Bax [Citation12]. As a potential diagnostic marker, miR-26 is elevated in follicular atresia, along with increased apoptosis of GCs [Citation13]. Moreover, miR-483 and miR-486 are downregulated in PCOS patients and are associated with the inhibition of insulin resistance and promotion of CCs proliferation, respectively [Citation14]. Thus, miRNAs play important roles in PCOS occurrence and progression, suggesting that an understanding of miRNA-mediated mechanisms would dramatically expand the knowledge of PCOS.
Exosomes are specialized nano-sized vesicles of endocytic origin secreted by many cell types [Citation15]. Exosomes are important mediators in intercellular communication by transferring functional miRNAs and proteins [Citation16]. Growing evidence reveals that exosomes derived from multiple cell types can carry and deliver functional miRNAs to recipient cells in vitro [Citation17,Citation18]. Adipose tissue-derived mesenchymal stem cells (AMSCs) are capable of producing abundant exosomes, suggesting that AMSCs may be applied as a mediator for transferring miRNAs in exosome-mediated intercellular interactions [Citation19]. In addition, AMSCs can communicate with brain parenchymal cells and deliver miR-133b through exosomes to regulate neurite outgrowth [Citation20]. Exosomes generated by miR-181-5P modified AMSCs suppress hepatic fibrosis in hepatic stellate cells [Citation21]. Furthermore, exosomes derived from miR-122-engineered AMSCs cause enhanced sensitivity to chemotherapeutic treatment in hepatocellular carcinoma cells [Citation22]. Hitherto, the exosomes-mediated communicative approach is rarely reported in PCOS.
MiR-323-3p participates in inflammatory and immune activities and plays important roles in regulating several disorders, such as ectopic pregnancy, coronary syndromes and rheumatoid arthritis [Citation23,Citation24]. Recently, miR-323-3p is reported to be involved in regulating the steroidogenesis and apoptosis in CCs in women with PCOS [Citation25]. Therefore, this study was aimed to determine the mechanisms underlying miR-323-3p-related activities in CCs and whether AMSCs-derived exosomes can be used as an effective mediator to deliver miR-323-3p to CCs.
Materials and methods
Clinical sample collection
The CCs samples were obtained from 10 female patients diagnosed with PCOS and 10 healthy females at the Capital Institute of Pediatrics from April 2014 to April 2015. All protocols of this study were approved by the ethics committee of Capital Institute of Pediatrics, and informed consent was obtained from each participant. The diagnosis was based on Rotterdam 2003 criteria [Citation26]. Before enrolling in this study, all patients did not take any hormonal drugs and were not pregnant.
CCs isolation
During the ovulation induction, follicular development was monitored through transvaginal ultrasound. Post 36-h hCG injection, the cumulus–oocyte complex (COC) retrieval was conducted following ultrasound echo-guidance. After COC retrieval, CCs were collected using a sharp needle and CCs were cultured in DMEM/F12 medium (Nissui Pharmaceutical, Tokyo, Japan) containing 10% FBS (Gibco BRL, Grand Island, NE) and 1% antibiotic–antimycotic.
AMSCs isolation and identification
Subcutaneous adipose tissues were obtained from 4 female patients (24, 32, 38 and 41 years old, respectively) at Capital Institute of Pediatrics. Adipose tissue was collected as previously described [Citation27] and cultured in MesenPRO® RS™ Medium containing 1% antibiotic–antimycotic (Gibco BRL, Grand Island, NE). The identification of AMSCs (passages 3 to 6) was determined by western blots of AMSCs surface markers: human leukocyte antigen-DR (HLA-DR) and PE-labeled cluster designation 29 (CD29), CD31, CD44, CD45, CD73, CD90, CD105 (BD Bioscience Pharmingen, San Diego, CA). The differentiation of AMSCs to adipocytes was evaluated using the adipogenesis differentiation kit (Gibco BRL, Grand Island, NE). Differentiated adipocytes were stained with Oil Red O as previously described [Citation28].
Plasmids and AMSC transfection
AMSCs were cultured in DMEM containing 10% FBS (Gibco BRL, Grand Island, NE) and 1% antibiotic–antimycotic. Protein aggregates and bovine exosomes were removed by ultracentrifugation at 100,000 g for 16 h at 4 °C. Next, AMSCs (1 × 106) were seeded in the DMEM mentioned above for 16 h. Then, AMSCs were transfected with plasmids of has-miR-323-3p mimic (forward 5′-CACAUUACACGGUCGACCUCU-3′ and reverse 5′-AGGUCGACCGUGUAAUGUGUU-3′) or cel-miR-67 (control) by using the Lipofectamine™ 2000 kit in accordance with the manufacturer’s instructions (Invitrogen, San Fransisco, CA).
AMSC exosomes isolation and identification
Post 48-h transfection, exosomes were isolated from the AMSCs supernatant by using the ExoQuick-TC Kit in accordance with the manufacturer’s instructions (System Biosciences, Palo Alto, CA). The identification of AMSC exosomes was evaluated by western blots of exosome surface markers: CD9, CD63 and CD81(BD Bioscience Pharmingen, San Diego, CA). Per centrifugal filters, precipitates from AMSCs-conditioned DMEM were used as a negative control. Exosome pellets were resuspended in PBS solution at 5 μg/μL protein concentration.
Quantitative real-time PCR
Total RNA was isolated from AMSCs, exosomes, or GCs using the Trizol Reagent kit in accordance with the manufacturer’s instructions (Invitrogen, Carlsbad, CA). The first strand of cDNAs was synthesized using the High Capacity cDNA Reverse Transcription kit (Applied Biosystems, Carlsbad, CA). The mRNA expressions were detected using the Fast SYBR Green Master Mix (Applied Biosystems, Carlsbad, CA) on a 7500 Fast Real‐Time PCR System (Applied Biosystems, Carlsbad, CA). Real‐time PCR data were analyzed using the ΔΔCT method with glyceraldehyde 3-phosphate dehydrogenase (GAPDH) as the reference gene. MiR-323-3p expression was detected using the EzOmics miRNA qPCR Detection Primer Set (Biomics, Santa Barbara, CA). The primer sequences were as following: programmed cell death protein 4 (PDCD4) forward, 5′-TGGATTAACTGTGCCAACCA-3′, reverse 5′-TCTCAAATGCCCTTTCATCC-3′; GAPDH forward 5′-TATGATGATATCAAGAGGGTAGT-3′, reverse 5′-TGTATCCAAACTCATTGTCATAC-3′.
CCs transfection
MiR-323-3p inhibitor and negative control were obtained from GenePharma (GenePharma Co., Ltd, Shanghai, China). The transfection of miR-323-3p inhibitor into CCs was performed according to the manufacturer’s instructions using the Lipofectamine™ 2000 Transfection Reagent (Invitrogen, Waltham, MA). After 24 h of transfection, the CCs were used for subsequent experiments.
Lentivirus construction, virus packaging, titration and transfection
The oligonucleotide sequences of miR-323-3p or anti-miR-323-3p expression vectors and pEGFP-C3 vectors were synthesized in accordance with the manufacturer’s instructions (BD Biosciences, San Jose, CA). Lentiviral vectors pLL3.7- miR-323-3p and pLL3.7-anti-miR-323-3p and constructed lentivirus plasmids were created in accordance with the manufacturer’s instructions (GenePharma Co., Ltd., Shanghai, China). The virus packaging was conducted using the Lipofectamine 2000 (Invitrogen, Waltham, MA). The supernatants containing lentivirus were collected and viral titer determination was conducted using the double dilution method. The CCs were cocultured with viral supernatant (1 ml/well) on the day of injection. After 48 h, these CCs were treated with puromycin to obtain the stable lentivirus-transfected CCs.
Flow cytometry and MTT assay
Cell cycle distribution and apoptosis were evaluated by flow cytometry analysis as previously described [Citation29]. The results were analyzed using ModFit software and FACSCaliber (BD Bioscience, San Jose, CA). Cell proliferation was evaluated using the MTT assay as previously described [Citation30]. Absorbance at a wavelength of 490 nm was detected using an ELx808 plate reader (BioTek Instruments, Inc., Winooski, VT).
Luciferase reporter assay
The 3’UTR PDCD4 containing the predicted wild-type or mutated miR-323-3p binding sites were amplified by PCR and cloned into the firefly luciferase reporter gene in pMIR (Ambion, Austin, TX). The engineered luciferase reporter plasmids PDCD4-wt or PDCD4-mut were transfected with miR-323-3p or miR-control into CCs using the Lipofectamine™ 2000 kit in accordance with the manufacturer’s instructions (Invitrogen, San Fransisco, CA). Post 48-h transfection, the luciferase activity was evaluated using the Dual-Luciferase Reporter Assay System kit (Promega, Fitchburg, WI).
Western blot
Total proteins were extracted from cultured AMSCs and CCs using the cell lysis buffer (Beyotime Institute of Biotechnology, Shanghai, China). The detailed protocol was performed as previously described [Citation31]. The primary antibodies included: PDCD4, proliferating cell nuclear antigen (PCNA), caspase-3, Bax, Bcl-2 and GAPDH obtained from Santa Cruz Biotechnology (Santa Cruz Biotechnology, Santa Cruz, CA). Quantification of optical density was evaluated using the Uvitec Alliance software (Eppendorf, Germany).
Animals and experimental protocols
The animal protocols were performed in accordance with the criteria for the Care and Use of Laboratory Animals and approved by the Ethics Committee of Capital Institute of Pediatrics. This study used 36 female C57BL/6 mice (7 weeks old, weighing 23.4 ± 1.6 g; Jackson Labs, Bar Harbor, WA), which were housed in a customized 12 h light/dark cycle at 24 °C. Food and water were provided ad libitum. Mice were divided into five groups, including a sham and four PCOS groups (lenti- miR-323-3p, lenti-miR-control, lenti-anti-miR-323-3p, or lenti-anti-miR-control). The letrozole-induced [Citation32] PCOS mouse model was established as previously described [Citation32]. After 5 weeks of inoculation, the mice were killed and freshly dissected ovaries were subjected to subsequent experiments.
ELISA assay
After 10 h of fasting, mouse blood samples were collected. The serum levels of follicle-stimulating hormone (FSH), luteinizing hormone (LH), testosterone and estradiol (E2) were detected using ELISA Kits in accordance with the manufacturer’s instructions (Yanhui, Shanghai, China).
Statistical analysis
Data were presented as means ± SEM. The sample size was replicated at least four times in each experiment. The statistical analyses were performed using SPSS 13.0 software (SPSS, Chicago, IL). The differences between groups were calculated using one-way ANOVA or Student’s t-test. Differences were considered statistically significant at p < .05.
Results
Identification of AMSCs and exosomes
After isolation and culturing AMSCs, multiple CD molecules were used to identify AMSCs. The results showed that CD31, CD34, CD45 and HLA-DR were downregulated while CD29, CD44, CD73, CD90 and CD105 were upregulated in AMSCs (). After subjecting to adipogenic induction treatment for 14 days, Oil Red O positive staining was imaged (), indicating the AMSCs had normal differentiation potential and could be used for subsequent experiments. In addition, the exosomal markers, CD9, CD63 and CD81 were upregulated in the AMSCs-derived exosomes ().
Figure 1. Identification of human adipose tissue-derived mesenchymal stem cells (AMSCs) and exosomes. (A) Western blot analysis of the surface markers in AMSCs. (B) Representative images of Human AMSCs. (D) Oil Red O straining in cumulus cells (CCs) treated with adipogenesis kit (original magnification × 100, Scale bar = 50 μm). (D) Western blot analysis of CD9, CD63 and CD81 expression in AMSC exosomes. “Exo-control” denotes precipitates from AMSCs, and DMEM were used as a negative control. “Exo-323-3p” denotes exosomes derived from modified AMSCs.
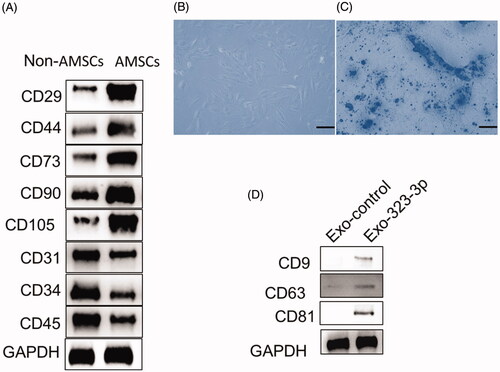
MiR-323-3p-modified AMSCs and exosomes
AMSCs were transfected with miR-323-3p-encoding plasmids and control miRNA plasmids containing cel-miR-67 for 48 h, respectively. The miR-323-3P expression level was detected by real-time PCR in AMSCs and exosomes. The results revealed that the miR-323-3P expression was upregulated in both miR-323-3p-transfected AMSCs () and exosomes (), respectively, suggesting miR-323-3p was successfully loaded into exosomes secreted by miR-323-3p-modified AMSCs.
Figure 2. Upregulation of miR-323-3p promoted cell growth and inhibited apoptosis in cumulus cells (CCs). Detection of miR-323-3p expression in (A) human adipose tissue-derived mesenchymal stem cells (AMSCs), (B) AMSC exosome and (C) CCs. (D) Cell proliferation. (E) Cell cycle distribution. (F) Bax Bcl-2, and caspase-3 protein expression in CCs transfected miR-323-3p-modified exosomes. (G) Apoptosis level in CCs transfected miR-323-3p-modified exosomes. (*) Denotes differences from the control group (p < .05). Values are means ± SEM. For each experiment, at least 4 samples were available for the analysis.
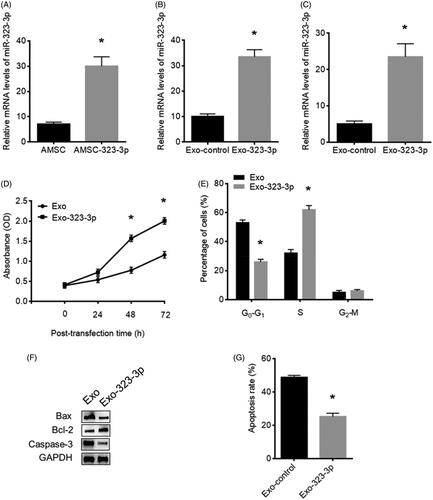
Upregulation of miR-323-3p in CCs cocultured with exosomes
After cocultured with exosomes, miR-323-3p expression was increased in the CCs compared with those cocultured with the control exosomes (), suggesting that exosomes derived from miR-323-3p-transfected AMSCs efficiently transferred miR-323-3p into CCs in vitro.
MiR-323-3p upregulation promoted cell growth and inhibited apoptosis in CCs
The MTT assay was used to determine the influence of upregulated miR-323-3p on CCs’ proliferation. The results showed that increased miR-323-3p expression promoted CCs’ proliferation compared with those transfected with control exosomes (). Also, upregulation of miR-323-3p led to reduced cell populations at G0–G1 phase while increased cell population at the S phase compared with the control group (). Furthermore, the decreased protein level of Bax and caspase-3 and elevated Bcl-2 expression demonstrated that miR-323-3p restoration also caused reduced CCs apoptosis (), which is consistent with results from the apoptosis assay ().
MiR-323-3p-inhibitor reversed effects of upregulation of miR-323-3p on CCs
To further confirm the function of miR-323-3p in CCs growth and apoptosis, the miR-323-3p-inhibitor was applied to transfect with exosome-treated CCs. The expression of miR-323-3p was decreased () after the treatment of the miR-323-3p inhibitor. The overall effects of upregulated miR-323-3p on CCs were abolished by the miR-323-3p inhibitor. Compared with the untreated groups, the CCs proliferation () and apoptosis () were suppressed and promoted, respectively. Meanwhile, the proportions of CCs at G0–G1 and S phase were also elevated and reduced, respectively (). Collectively, miR-323-3p plays important roles in CCs’ growth and apoptosis.
Figure 3. MiR-323-3p inhibitor reversed effect of miR-323-3p on cumulus cells (CCs). (A) miR-323-3p expression in CCs transfected with miR-323-3p inhibitor. (B) Cell proliferation, (C) cell cycle distribution and (D) apoptosis level in CCs transfected with the miR-323-3p inhibitor. (*) Denotes differences from the control group (p < .05). Values are means ± SEM. For each experiment, at least 4 samples were available for the analysis.
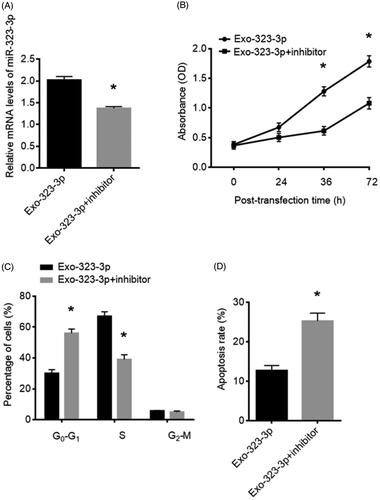
MiR-323-3p targeted PDCD4 in CCs
Through prediction using the bioinformatics tools: PicTar (http://pictar.mdc-berlin.de/) and TargetScan (www.targetscan.org/index.html), a binding sequence of miR-323-3p was found in the 3’UTR of PDCD4 (). To investigate the function of PDCD4 in CCs and the relationship with miR-323-3p, the protein expression of PDCD4 in CCs of PCOS patients were detected and the results showed that PDCD4 was highly expressed in CCs of patients with PCOS (). Also, cotransfection with miR-323-3p-loaded exosomes significantly reduced PDCD4 protein level (), suggesting that the miR-323-3p level had an inverse relationship with PDCD4 abundance. To further confirm this, the luciferase reporter assay showed that luciferase activity was reduced in miR-323-3p-transfected CCs with wild-type 3’UTR reporter, while no luciferase activity change was found in miR-323-3p-transfected CCs with the mutant 3’UTR reporter (), suggesting that the PDCD4 was a direct target of miR-323-3p in CCs.
Figure 4. MiR-323-3p directly targets PDCD4 in CCs. (A) PDCD4 protein expression in PCOS CCs. (B) PDCD4 protein expression in CCs transfected with exosomes loaded with miR-323-3p. (C) PDCD4 protein expression in CCs transfected with miR-323-3p-motified exosomes and miR-323-3p inhibitor. (D) Sequence information about the wildtype and mutant miR-323-3p binding sites in the 3’UTR of PDCD4. (E) Relative luciferase activity. (*) Denotes differences from the control group (p < .05). Values are means ± SEM. For each experiment, at least 4 samples were available for the analysis.
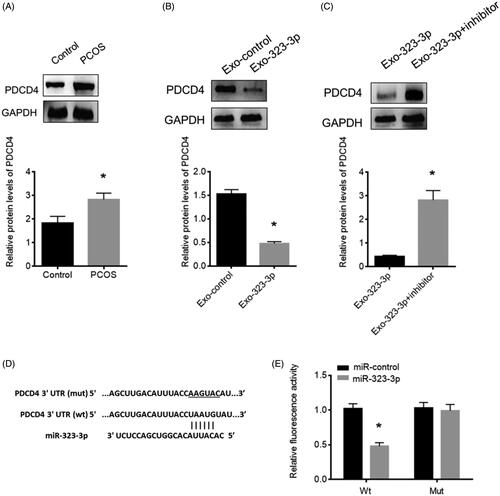
Overexpression of PDCD4 reversed effects of upregulation of miR-323-3p on CCs
To investigate whether PDCD4 functionally participated in the effect of miR-323-3p on CCs, exosome-treated CCs were transfected with PDCD4-pcDNA. The MTT assay results showed that PDCD4 overexpression attenuated the stimulatory effect of miR-323-3p on CCs’ proliferation (). Also, PDCD4-pcDNA treatment significantly increased the CCs population at G0–G1 phase while reduced CCs at the S phase compared with the control groups (). Furthermore, the apoptosis level was increased following PDCD4 overexpression in CCs with forced expression of miR-323-3p (). Collectively, the results showed that PDCD was not only the direct target of miR-323-3p, but also may mediate its biological roles in CCs’ growth and apoptosis.
Figure 5. MiR-323-3p promoted cell growth and inhibited apoptosis in cumulus cells (CCs) by targeting PDCD4. CCs were transfected with miR-323-3p and miR-323-3p + PDCD4-pcDNA. (A) Cell proliferation. (B) Cell cycle distribution. (D) Apoptosis level. (*) Denotes differences from the control group (p < .05). Values are means ± SEM. For each experiment, at least 4 samples were available for the analysis.
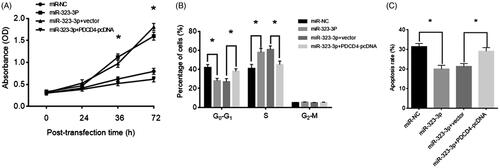
Upregulation of miR-323-3p ameliorated PCOS in vivo
To further confirm the effects of miR-323-3p on PCOS of CCs in vivo, letrozole was applied to establish a PCOS mouse model [Citation32]. In this model, the serum levels of FSH, LH and testosterone were upregulated while the E2 level was downregulated compared with those of the sham group (), which indicated the PCOS mouse model was successful and could be used for subsequent experiments. After 5 weeks of inoculation, miR-323-3p expression was increased in lenti-miR-323-3p treated mice and decreased in lenti-anti-miR-323-3p-treated mice compared with those of the control group (). Nevertheless, the changing pattern of PDCD expression was opposite to those of miR-323-3p (). Also, lenti-miR-323-3p treatment resulted in elevated PCNA and reduced caspase-3 levels, respectively, (), suggesting that miR-323-3p overexpression facilitated CCs growth while inhibited apoptosis in PCOS in vivo. Together, the results showed that effects of miR-323-3p on PCOS may be mediated by targeting PDCD4 in vivo.
Figure 6. MiR-323-3p promoted cell growth and inhibited apoptosis in vivo. The serum levels of (A) FSH, (B) LH, (C) testosterone and (D) E2. (E) MiR-323-3p expression. (F) PDCD4 protein expression. (G) Protein expressions of PCNA, PDCD4 and caspase-3. (*) Denotes differences from the control group (p < .05). Values are means ± SEM. For each experiment, at least 4 samples were available for the analysis.
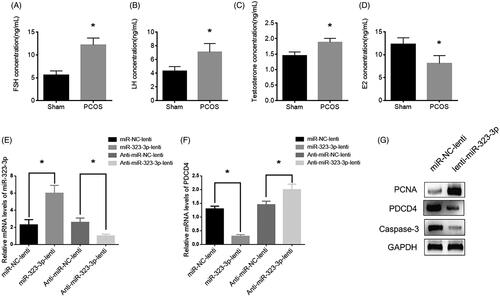
Discussion
One of the most common endocrine diseases, PCOS, affects females of reproductive age [Citation33]. In addition, PCOS is characterized by ovulatory dysfunction, hyperandrogenism, as well as, polycystic ovarian morphology (PCOM), which leads to substantial psychological, social and economic burdens for patients and their families [Citation1]. Due to its heterogeneity in presentation, it is still controversial to define PCOS across multiple disciplines, such as gynecology, endocrinology and psychiatry [Citation34]. Thus, determining the pathogenesis of PCOS and classifying its pathological manifestation would be a challenge for both basic scientific and clinical research fields [Citation34]. In this study, AMSCs and AMSCs-derived exosomes were greatly advantageous in researching a stem cell-based therapeutic strategy for PCOS in both in vitro and in vivo. Also, exogenous miR-323-3p delivered by exosomes promoted proliferation and inhibited apoptosis in CCs by targeting PDCD4.
Nanometer-size exosomes are endocytic origin vesicles secreted by various cell types [Citation15]. To date, exosomes have been widely used as mediators for intercellular communication through transferring small molecules, including proteins, DNAs and RNAs. This application is also regarded as an emerging approach to deliver functional factors to regulate disease progression [Citation17]. Furthermore, increasing evidence demonstrates that AMSCs is capable of producing mass exosomes [Citation35], which may exert essential roles in the exosome-based therapy in both scientific and clinical practices through the paracrine mechanism [Citation19].
In this study, modified AMSCs-originated exosomes through upregulation of miR-323-3p were delivered to CCs, thereby elevating the expression of miR-323-3p in CCs. The results showed that increased miR-323-3p level significantly suppressed CCs apoptosis, suggesting AMSC exosomes were an efficient way to deliver functional molecules, such as miRNAs, in the intervention of PCOS. More importantly, this has been applied successfully in the xenograft model in the present study. Recently, multiple studies have shown that manipulation of MSCs and their exosomes by upregulation of certain miRNAs are an effective approach to regulate the cell properties. For example, exosomes originated from MSCs enriched with miRNA-let7c can attenuate renal fibrosis [Citation36]. Exosomes with highly expressing miR-146 cause M2 polarization and increase survival in sepsis in mice [Citation37]. Highly miR-221-expressing exosomes secreted from bone marrow mesenchymal stem cells (BM-MSCs) effectively influenced gastric cancer aggressiveness and tumor microenvironments [Citation38]. Taken together, exosomes from miRNA-engineered MSCs provide therapeutic potential for PCOS in future basic and clinical research. Meanwhile, these approaches should be used in caution until more mechanisms can be further elucidated.
As a well-documented biomarker, miR-323-3p participates in immune and inflammatory processes and exerts essential roles in the pathogenesis of multiple diseases, for example, ectopic pregnancy and Alzheimer’s disease [Citation39]. Zhao et al. reported that circulating miR-323-3p can be a diagnostic marker to diagnose ectopic pregnancy in the early stages [Citation23]. Feng et al. found that there was a downregulation of miR-323-3p in the follicular fluid of patients with low-quality embryos [Citation40]. Also, miR-323-3p has been detected in the maternal plasma during pregnancy with a time-dependent pattern [Citation41]. In the present study, upregulated miR-323-3p in AMSCs-derived exosomes could elevate miR-323-3p level in CCs, thereby suppressing apoptosis and facilitating cell proliferation, which suggests that miR-323-3p may be an important mediator for CCs’ viability and plays critical roles in the regulation of PCOS progression.
On the basis of previous studies, low-expression of miR-323-3p was found in CCs of patients with PCOS [Citation25]. Thus, the present study used online tools to predict the targeting genes of miR-323-3p and identified PDCD4 as the targeting gene of miR-323-3p in the regulation of CCs’ activities. Moreover, PDCD4 has been identified as a key factor highly expressing in the apoptosis process [Citation42] and plays roles as a protein translation inhibitor by suppressing RNA-helicase activity through binding to the translation initiation factor eIF4A [Citation43]. The aberrant expression of PDCD4 gives rise to a series of diseases and cell dysfunctions [Citation44]. With regards to PCOS, growing evidence suggests that PDCD4 is involved in PCOS pathogenesis. For example, PDCD4 is upregulated in PCOS patients while knockdown of PDCD4 reduces apoptosis of GCs in females with PCOS [Citation45]. In the present study, there was a high expression of PDCD4 in patients with PCOS compared with the control group and the expression of PDCD4 could be suppressed by exogenous miR-323-3p transferred by AMSCs-derived exosomes. Since CCs apoptosis is elevated in PCOS to induce premature follicular atresia [Citation46], the results suggest that upregulated PDCD4 contributes to premature apoptosis in CCs of PCOS and its pro-apoptotic effect could be abolished by miR-323-3p.
In conclusion, the present study demonstrated that upregulation of miR-323-3p suppressed CCs apoptosis by targeting PDCD4 in PCOS and that the delivery of miR-323-3p through AMSC exosomes was an effective approach for the regulation of PCOS progression. These findings may provide a novel insight into developing the therapeutic application of PCOS.
Disclosure statement
No potential conflict of interest was reported by the authors.
References
- Azziz R, Carmina E, Chen Z, et al. Polycystic ovary syndrome. Nat Rev Dis Primers. 2016;2(1):16057.
- Diamanti-Kandarakis E, Spritzer PM, Sir-Petermann T, et al. Insulin resistance and polycystic ovary syndrome through life. CPD. 2012;18(34):5569–5576.
- Diamanti-Kandarakis E, Dunaif A. Insulin resistance and the polycystic ovary syndrome revisited: an update on mechanisms and implications. Endocr Rev. 2012;33(6):981–1030.
- Vink JM, Sadrzadeh S, Lambalk CB, et al. Heritability of polycystic ovary syndrome in a Dutch twin-family study. J Clin Endocrinol Metab. 2006;91(6):2100–2104.
- Azziz R, Marin C, Hoq L, et al. Health care-related economic burden of the polycystic ovary syndrome during the reproductive life span. J Clin Endocrinol Metab. 2005;90(8):4650–4658.
- Pasquali R, Stener-Victorin E, Yildiz BO, et al. PCOS Forum: research in polycystic ovary syndrome today and tomorrow. Clin Endocrinol (Oxf). 2011;74(4):424–433.
- Das M, Djahanbakhch O, Hacihanefioglu B, et al. Granulosa cell survival and proliferation are altered in polycystic ovary syndrome. J Clin Endocrinol Metab. 2008;93(3):881–887.
- Gilchrist RB, Lane M, Thompson JG. Oocyte-secreted factors: regulators of cumulus cell function and oocyte quality. Hum Reprod Update. 2008;14(2):159–177.
- Salehi E, Aflatoonian R, Moeini A, et al. Apoptotic biomarkers in cumulus cells in relation to embryo quality in polycystic ovary syndrome. Arch Gynecol Obstet. 2017;296(6):1219–1227.
- Shalev E, Goldman S, Ben-Shlomo I. The balance between MMP-9 and MMP-2 and their tissue inhibitor (TIMP)-1 in luteinized granulosa cells: comparison between women with PCOS and normal ovulatory women. Mol Human Reprod. 2001;7(4):325–331.
- Flynt AS, Lai EC. Biological principles of microRNA-mediated regulation: shared themes amid diversity. Nat Rev Genet. 2008;9(11):831–842.
- Sirotkin AV, Laukova M, Ovcharenko D, et al. Identification of microRNAs controlling human ovarian cell proliferation and apoptosis. J Cell Physiol. 2010;223(1):49–56.
- Sang Q, Yao Z, Wang H, et al. Identification of microRNAs in human follicular fluid: characterization of microRNAs that govern steroidogenesis in vitro and are associated with polycystic ovary syndrome in vivo. J Clin Endocrinol Metab. 2013;98(7):3068–3079.
- Shi L, Liu S, Zhao W, et al. miR-483-5p and miR-486-5p are down-regulated in cumulus cells of metaphase II oocytes from women with polycystic ovary syndrome. Reprod Biomed Online. 2015;31(4):565–572.
- Zomer A, Vendrig T, Hopmans ES, et al. Exosomes: fit to deliver small RNA. Commun Integrat Biol. 2010;3(5):447–450.
- Pegtel DM, Cosmopoulos K, Thorley-Lawson DA, et al. Functional delivery of viral miRNAs via exosomes. Proc Nat Acad Sci USA. 2010;107(14):6328–6333.
- Zhang X, Yuan X, Shi H, et al. Exosomes in cancer: small particle, big player. J Hematol Oncol. 2015;8:83.
- O’Loughlin AJ, Woffindale CA, Wood MJ. Exosomes and the emerging field of exosome-based gene therapy. Curr Gene Ther. 2012;12(4):262–274.
- Yeo RW, Lai RC, Zhang B, et al. Mesenchymal stem cell: an efficient mass producer of exosomes for drug delivery. Adv Drug Deliv Rev. 2013;65(3):336–341.
- Xin H, Li Y, Buller B, et al. Exosome-mediated transfer of miR-133b from multipotent mesenchymal stromal cells to neural cells contributes to neurite outgrowth. Stem Cells. 2012;30(7):1556–1564.
- Qu Y, Zhang Q, Cai X, et al. Exosomes derived from miR-181-5p-modified adipose-derived mesenchymal stem cells prevent liver fibrosis via autophagy activation. J Cell Mol Med. 2017;21(10):2491–2502.
- Lou G, Song X, Yang F, et al. Exosomes derived from miR-122-modified adipose tissue-derived MSCs increase chemosensitivity of hepatocellular carcinoma. J Hematol Oncol. 2015;8:122.
- Zhao Z, Zhao Q, Warrick J, et al. Circulating microRNA miR-323-3p as a biomarker of ectopic pregnancy. Clin Chem. 2012;58(5):896–905.
- Xu T, Huang C, Chen Z, et al. MicroRNA-323-3p: a new biomarker and potential therapeutic target for rheumatoid arthritis. Rheumatol Int. 2014;34(5):721–722.
- Wang T, Liu Y, Lv M, et al. miR-323-3p regulates the steroidogenesis and cell apoptosis in polycystic ovary syndrome (PCOS) by targeting IGF-1. Gene. 2019;683:87–100.
- Revised 2003 consensus on diagnostic criteria and long-term health risks related to polycystic ovary syndrome. Fertil Steril. 2004;81(1):19–25.
- Liu Y, Yan X, Sun Z, et al. Flk-1+ adipose-derived mesenchymal stem cells differentiate into skeletal muscle satellite cells and ameliorate muscular dystrophy in mdx mice. Stem Cells Develop. 2007;16(5):695–706.
- Ullah M, Stich S, Notter M, et al. Transdifferentiation of mesenchymal stem cells-derived adipogenic-differentiated cells into osteogenic- or chondrogenic-differentiated cells proceeds via dedifferentiation and have a correlation with cell cycle arresting and driving genes. Differentiation. 2013;85(3):78–90.
- Zhang FZ, Ho DH, Wong RH. Triptolide, a HSP90 middle domain inhibitor, induces apoptosis in triple manner. Oncotarget. 2018;9(32):22301–22315.
- Zhang Y, Chao T, Li R, et al. MicroRNA-128 inhibits glioma cells proliferation by targeting transcription factor E2F3a. J Mol Med. 2009;87(1):43–51.
- Pulito C, Mori F, Sacconi A, et al. Metformin-induced ablation of microRNA 21-5p releases Sestrin-1 and CAB39L antitumoral activities. Cell Discov. 2017;3(1):17022.
- Kelley ST, Skarra DV, Rivera AJ, et al. The gut microbiome is altered in a letrozole-induced mouse model of polycystic ovary syndrome. PLoS One. 2016;11(1):e0146509.
- Ehrmann DA. Polycystic ovary syndrome. N Engl J Med. 2005;352(12):1223–1236.
- Norman RJ, Dewailly D, Legro RS, et al. Polycystic ovary syndrome. Lancet. 2007;370(9588):685–697.
- Eirin A, Riester SM, Zhu XY, et al. MicroRNA and mRNA cargo of extracellular vesicles from porcine adipose tissue-derived mesenchymal stem cells. Gene. 2014;551(1):55–64.
- Wang B, Yao K, Huuskes BM, et al. Mesenchymal Stem Cells Deliver Exogenous MicroRNA-let7c via Exosomes to Attenuate Renal Fibrosis. Mol Ther: J Am Soc Gene Ther. 2016;24(7):1290–1301.
- Song Y, Dou H, Li X, et al. Exosomal miR-146a contributes to the enhanced therapeutic efficacy of interleukin-1beta-primed mesenchymal stem cells against sepsis. Stem Cells. 2017;35(5):1208–1221.
- Ma M, Chen S, Liu Z, et al. miRNA-221 of exosomes originating from bone marrow mesenchymal stem cells promotes oncogenic activity in gastric cancer. OTT. 2017;10:4161–4171.
- Xu T, Li L, Huang C, et al. MicroRNA-323-3p with clinical potential in rheumatoid arthritis, Alzheimer’s disease and ectopic pregnancy. Expert Op Therap Targets. 2014;18(2):153–158.
- Feng R, Sang Q, Zhu Y, et al. MiRNA-320 in the human follicular fluid is associated with embryo quality in vivo and affects mouse embryonic development in vitro. Sci Rep. 2015;5(1):8689.
- Miura K, Miura S, Yamasaki K, et al. Identification of pregnancy-associated microRNAs in maternal plasma. Clin Chem. 2010;56(11):1767–1771.
- Lankat-Buttgereit B, Goke R. Programmed cell death protein 4 (pdcd4): a novel target for antineoplastic therapy? Biol Cell. 2003;95(8):515–519.
- Yang HS, Cho MH, Zakowicz H, et al. A novel function of the MA-3 domains in transformation and translation suppressor Pdcd4 is essential for its binding to eukaryotic translation initiation factor 4A. Mol Cell Biol. 2004;24(9):3894–3906.
- Jurisicova A, Latham KE, Casper RF, et al. Expression and regulation of genes associated with cell death during murine preimplantation embryo development. Mol Reprod Dev. 1998;51(3):243–253.
- Ding L, Gao F, Zhang M, et al. Higher PDCD4 expression is associated with obesity, insulin resistance, lipid metabolism disorders, and granulosa cell apoptosis in polycystic ovary syndrome. Fertil Steril. 2016;105(5):1330.e3–1337.e3.
- Cataldo NA, Dumesic DA, Goldsmith PC, et al. Immunolocalization of Fas and Fas ligand in the ovaries of women with polycystic ovary syndrome: relationship to apoptosis. Human Reprod. 2000;15(9):1889–1897.