Abstract
Diabetic cardiomyopathy (DCM) is an important cardiac disorder in patients with diabetes. High glucose (HG) levels lead to inflammation of cardiomyocytes, oxidative stress, and long-term activation of autophagy, resulting in myocardial fibrosis and remodelling. Astragaloside-IV (AS-IV) has a wide range of pharmacological effects. This study aimed to investigate the effects of AS-IV on injury induced by HG in rat cardiomyocytes (H9C2(2-1)) and the involvement of the miR-34a-mediated autophagy pathway. An AS-IV concentration of 100 μM was selected based on H9C2(2-1) cell viability using the cell counting kit-8 (CCK-8). We found that 33 mM HG induced a morphologic change in cells and caused excessive oxidative stress, whereas AS-IV inhibited lipid peroxidation and increased superoxide dismutase activity. In terms of mRNA expression, HG increased miR-34a and inhibited Bcl2 and Sirt1, whereas AS-IV and miR-34a-inhibitor reversed the above effects. Further, LC3-GFP adenovirus infection and western blotting showed that HG increased autophagy, which was reversed synergistically by AS-IV and miR-34a-inhibitor. Bcl2 and pAKT/AKT protein expressions in the HG group was significantly lower than that in controls, but AS-IV and miR-34a-inhibitor antagonized the process. Thus, AS-IV inhibits HG-induced oxidative stress and autophagy and protects cardiomyocytes from injury via the miR-34a/Bcl2/(LC3II/LC3I) and pAKT/Bcl2/(LC3II/LC3I) pathways.
Introduction
Astragalus membranaceus has been used in Chinese traditional medicine for more than 2000 years. It has been widely used with the aim of promoting qi, detoxifying and promoting pus drainage and granulation. Medicinal astragalus is the root of A. membranaceus (Fisch.) Bunge var. membranaceus and A. membranaceus (Fisch.) Bunge var. mongholicus (Bunge) P. K. Hsiao. Astragaloside IV (AS-IV) is a highly effective triterpenoid saponin isolated and purified from A. membranaceus. The molecular formula of AS-IV is C41H68O14 (). AS-IV has been reported to show antioxidative stress properties, and to affect immune modulation, including anti-viral and anti-inflammatory effects [Citation1–3]. AS-IV has various mechanisms to protect the myocardium, including an antioxidant and free radical removal function [Citation4]. Astragalus has a detoxification and muscle regeneration role in the treatment of long-term muscle ulcers, indicating that astragalus functions as a scavenger in tissue and organ repair and regeneration. We hypothesized that one of the important pharmacological action of astragalus is to participate in cell autophagy.
Figure 1. AS-IV is a triterpenoid saponin extracted from Astragalus membranaceus. The molecular formula is C41H68O14.
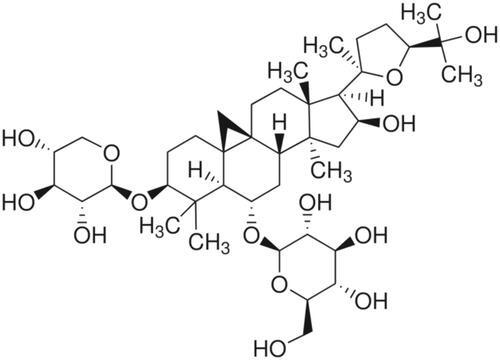
Diabetes affects the heart in three ways: coronary artery disease, cardiac autonomic neuropathy, and diabetic cardiomyopathy (DCM). DCM is an important complication that leads to mortality and morbidity. The mechanisms that cause DCM remain unclear with various factors potentially playing a role in the development of this disease. These include excessive oxidative stress of cardiomyocytes, inflammation, long-term autophagy activation, lipotoxicity, glucose toxicity, mitochondrial damage, long-term endoplasmic reticulum stress activation, changes in sarcolemmal makeup, such as lipid composition (cholesterol and triglyceride accumulation, fatty acid saturation vs. reduced desaturation, phospholipid remodelling) that contribute to modulation of caveolar domains, gap junctions, and T-tubules resulting in myocardial fibrosis and remodelling [Citation5,Citation6]. microRNAs (miRNAs) are a hot topic in the life sciences. They are small endogenous noncoding RNAs with 21–22 nucleotides. The latest data from miRbase database shows more than 2000 mature miRNA encoding human genes. Most miRNAs are expressed in the human heart, and approximately 60–70% of mature miRNAs, after multiple steps of complex biosynthesis, are closely related to the occurrence and development of myocardial fibrosis in various heart diseases. miRNA is a major regulatory factor in cardiovascular function [Citation7]. miRNA can play a role in post-transcriptional regulation by binding the 3'UTR of a target gene mRNA and inhibiting posttranscriptional translation or degradation, affecting cell proliferation and differentiation and autophagy [Citation8]. Therefore, miRNAs are key regulatory factors in myocardial fibrosis-related diseases. We hypothesized that miRNAs may serve as a new therapeutic target and potential biomarker for cardiovascular diseases [Citation9,Citation10].
Autophagy is an evolutionarily conserved and strictly regulated lysosomal-dependent pathway that degrades cytoplasmic material and damaged organelles [Citation11]. Autophagy has been found to contribute to the pathogenesis of DCM. In type 2 diabetic animals, autophagy was activated in cardiomyocytes, which was closely related to the down-regulation of the PI3K-Akt pathway and the production of reactive oxygen species (ROS) [Citation12]. Therefore, autophagy may play a protective role in the pathogenesis of acute ischaemic heart disease. However, in chronic diseases, such as diabetes or heart disease, long-term autophagy may have adverse effects in the myocardium [Citation13,Citation14]. In general, glucose and lipid metabolism disorders, hyperinsulinemia, and other signalling pathway changes in DCM may affect myocardial autophagy [Citation15]. Therefore, the autophagy state of DCM is the final manifestation of numerous mechanisms. We investigated the effect of AS-IV on miR-34a-mediated autophagy in HG-induced injury of H9C2(2-1) cells.
Materials and methods
Reagents
AS-IV (>98.0% purity), D-(+)-glucose (G7021-100G) and DMEM/LOW with L-Glutamine, Sodium Pyruvate (D6046-500ML) were purchased from Sigma-Aldrich (St. Louis, MO, USA). Foetal bovine serum (FBS) (SH3007.03) and DMEM High Glucose Medium (SH30243.1) were purchased from HyClone (Logan, UT, USA). Penicillin/streptomycin (15140122) and 0.25% Trypsin (25200-072) were purchased from Gibco (Grand Island, Nebraska, USA). TriPure Isolation Reagent (11667165001) was purchased from Roche (Darmstadt, Germany). Antibodies against Bcl2 (CST 4223), Sirt1 (CST 2496 T), caspase-3 (CST 9664), and LC3B (CST 2775) were purchased from Cell Signalling Technology (Danvers, MA, USA). Antibodies against LC3 (ab192890), P62 (ab91526), Tubulin (ab7291) and Goat anti Rabbit IgG (ab6721) were purchased from Abcam (Cambridge, MA, USA). Lipofectamine 3000 (L3000015) was purchased from Thermo Fisher Scientific (Waltham, MA, USA). miR-34a mimics and inhibitor was synthesized by GenePharma (Shanghai, China).
Cell culture
H9C2(2-1) cardiomyocytes were obtained from the Cell Bank of Chinese Academy of Sciences (CTCC, Shanghai, China) and incubated in DMEM with 10% FBS and penicillin/streptomycin at 37 °C, 5% CO2. Media were replaced every 2–3 days. The cells were subcultured or subjected to experimental procedures at 80–90% confluence. The cells were serum-starved overnight and randomized into six groups: normal group (5.5 mM D-glucose, NG), high glucose group (33 mM D-glucose, HG), high glucose + AS-IV group (treated with 12.5, 25, 50, and 100 μM AS-IV, HG + AS-IV).
Cell viability assay
H9C2(2-1) cell viability was assessed using Cell Count Kit-8 (CCK-8, Biosharp, Hefei, China). In each group, H9C2(2-1) cells (100 μL) were seeded in a 96-well plate at a density of 1–2 × 104 cells/mL overnight. Subsequently, the prepared 100 μL media containing 10% CCK-8 was added through media exchange modes. The cells were cultured for 2 h. The absorbance at 450 nm was measured using a microplate reader (Thermo Fisher Scientific, Waltham, MA, USA). All experiments were performed after a 48-h incubation. Each experiment was repeated in at least three different cell preparations. The AS-IV concentration suitable for the cell viability assay was determined at 100 μM.
H9C2(2-1) cells transfected with miR-34a inhibitor
H9C2(2-1) cells were seeded into a six-well plate and used for in vitro transfection with miR-34a inhibitor or miR-34a mimics (miR-34a-inhibitor N.C) (GenePharma, Shanghai, China) using Lipofectamine 3000 reagent. After 48 h, the cells were collected and randomised into seven groups: normal group (5.5 mM D-glucose, NG), high glucose group (33 mM D-glucose, HG), high glucose + AS-IV group (treated with 100 μM AS-IV, HG + AS-IV), high glucose + miR-34a inhibitor group (HG + miR-34a-inhibitor), high glucose + AS-IV + miR-34a inhibitor group (HG + AS-IV + miR-34a-inhibitor), high glucose + miR-34a inhibitor control group (HG + miR-34a-inhibitor N.C), high glucose + AS-IV + miR-34a inhibitor control group (HG + AS-IV + miR-34a-inhibitor N.C).
Cell morphology and biochemical index test
Cell morphology was observed using a fluorescent inverted microscope. The activity levels of superoxidase dismutase (SOD) (A001-1), malondialdehyde (MDA) (A003-1), and lactate dehydrogenase (LDH) (A020-2) were measured using commercial kits from the Institute of Jiancheng Bioengineering (Nanjing, China).
Detection of autophagy flow and autophagosome
A total of 10 μL LC3-GFP adenovirus was added to 293β5 cells with a density of about 70%, and the culture medium was changed after 48 h. When the cells fluorescence reached 100% and one-third of the cells floated, the cells were blown off with 1 ml phosphate buffer saline (PBS) and collected. The cells were repeatedly frozen and thawed at −80 °C and 37°C for four times, centrifuged at 12,000 rpm for 10 min, and the supernatant was collected under sterile conditions and filtered through a 0.22 μM filter. Seven groups of H9C2(2-1) cells with different interventions were transfected for 48 h, and after AS-IV treatment for 24 h, 100 μL LC3-GFP adenovirus was added to continue infection for 48 h. The cells with GFP dots were quantified under a fluorescence microscope (Nikon Ti-S, Japan) with 500× magnification. In addition, pre-treated H9C2(2-1) cells were washed with PBS, digested with trypsin and collected in centrifugal tubes. Centrifuged cell suspension with 2000 rpm for 10 min, and discarded supernatant. The cells were suspended in blocks using a fixative. The samples were transferred to relevant inspection personnel for electron microscope (Hitachi, Japan).
Total RNA extraction and real-time PCR
Cells were homogenized in 1 ml Tripure Isolation Reagent (Roche, Darmstadt, Germany). Total RNA was isolated based on the manufacturer’s protocol and stored at −70 °C. cDNA was synthesized from total RNA by reverse transcriptase (Fermentas) with primer oligo (dT) 18 and stored at −20 °C. mRNA expression levels were measured using real-time PCR and detected using CFX96™ (Bio-Rad). The following primers were used for PCR: Bcl2 (forward, 5′-GGTGGACAACATCGCTCTGTGG-3′; reverse, 5′-GGTATGCACCCAGAGTGATGCAGG-3′); Sirt1 (forward, 5′-TGCCATCATGAAGCCAGAGA-3′; reverse, 5′-CATCGCAGTCTCCAAGAAGC-3′); and GAPDH (forward, 5′-CACTAAAGGGCATCCTGGGCTACAC-3′; reverse, 5′-GGAGGCCATGTAGGCCATGAGG-3′). Quantitative real-time PCR was used for microRNA. U6 was chosen as control for small RNAs. Mature miR-34a primers and U6 snRNA were synthesized by GenScript Co. Ltd. (China). The following primers were used: miR-34a (forward, 5′-CGCGAATCAGCAAGTATAACTGCC-3′; reverse, 5′-CGTATCCAGTGCGTGTCGTGG-3′; reverse transcription, GTCGTATCCAGTGCGTGTCGTGGAGTCGGCAATTGCACTGGATACGACTAGGGC); U6 (forward, 5′-CTCACTTCGGCAGCACATA-3′; reverse, 5′AACTCTTCACGATTTTGTCTGTC-3′; reverse transcription, GTCGTATCCAGTGCGTGTCGTGGAGTCGGCAATTGCACTGGATACGACTCGGTTTGC). Amplification and measurement were performed with BIO-RAD CFX96 using the following conditions: denaturation at 95 °C for 10 min, 40 cycles of denaturation at 95 °C for 15 s, annealing at 60 °C for 20 s, and extension at 72 °C for 25 s.
Protein extraction and Western blot analysis
The cells cultured in 24-well plate were lysed in RIPA buffer (50 mM Tris pH 7.5, 150 mM NaCl, 1% TritonX-100, 0.1–1% sodium deoxycholate, 0.1% SDS) on ice for 30 min. The lysates were centrifugated at 4 °C for 15 min at 13,000 rpm. The extracted protein concentrations were analyzed using the bicinchoninic acid (BCA) protein assay (Beyotime Institute of Biotechnology, China). Equal amounts of protein were loaded per lane using SDS-PAGE and transferred onto PVDF membranes. Non-fat milk in tris-buffered saline supplemented with Tween 20 (TBST) buffer (5%) was used to block non-specific binding for 1 h. Blots were incubated overnight at 4 °C with primary antibodies at the dilutions recommended by the manufacturer. The secondary antibodies conjugated with horseradish peroxidase were added for 1 h, and ECL reagent was added to visualize the immunostained proteins. The band intensities were analyzed using Image J software.
Statistical analysis
Data are expressed as mean ± SD. Quantitative data were collected from at least six experiments and expressed as numbers and percentages (%). Statistical significance was determined using one-way analysis of variance, followed by Tukey’s test. Multi-group analysis was performed using an F test. Statistical significance was defined as p < .05.
Results
Effect of as-IV on the viability of H9C2(2-1) cells
In DMEM with 10% FBS, the optical density (OD) value in the HG group was significantly higher than in NG group (p<.001), indicating that the HG level significantly increased the hyperactivity of H9C2(2-1) cells. Compared with the HG group, the OD value of AS-IV treated groups gradually decreased in a dose-dependent manner (), showing that AS-IV inhibited HG-induced hyperactivity of H9C2(2-1) cells. The inhibitory effect of AS-IV on HG-induced hyperactivity was weakest at a low concentration (12.5 μM), and strongest at 50–100 μM. The OD value was not significantly different between the HG + miR-34a-inhibitor N.C. and HG groups, whereas the OD value in the HG + miR-34a-inhibitor group was lower than that in the HG group, suggesting that miR-34a-inhibitor inhibited the hyperactivity of H9C2(2-1) cells induced by HG levels. The OD value in the HG + AS-IV + miR-34a-inhibitor group was the lowest, indicating that the combination of 100 μM AS-IV and miR-34a-inhibitor synergistically inhibited HG-induced hyperactivity of H9C2(2-1) cells ().
Figure 2. (a) The effect of different concentrations of AS-IV on the viability of HG-damaged H9C2(2-1) cell. X axis: normal group (NG, 5 mM), HG group (HG, 33 mM) and HG combined with different AS-IV concentrations; Y axis: H9C2(2-1) cell viability, absorbance (OD 450 value) by CCK-8 assays; ***p < .001. (b) The effect of miR-34a and AS-IV on the viability of HG-damaged H9C2(2-1) cells. X axis: different intervention groups of H9C2(2-1) cells; Y axis: H9C2(2-1) cell viability, absorbance (OD450 value) by CCK-8 assays; *p < .05, **p < .01.
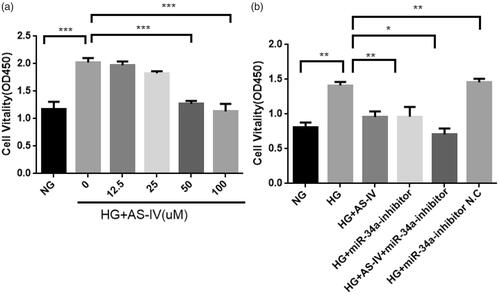
Effect of culture conditions on H9C2(2-1) cell morphology
H9C2(2-1) cells in the NG group were regularly arranged, full-shaped and with an intact cell membrane and good refractivity. Cells adhered firmly to the wall showing active proliferation (). After incubation in HG level (33 mM) for 48 h, the H9C2(2-1) cells in the HG and HG + miR-34a-inhibitor N.C. groups showed morphologic variation, poor refractivity, slight cell oedema and an irregularly arranged form (). HG-induced H9C2(2-1) cell damage improved after intervention in the HG + AS-IV, HG + miR-34a-inhibitor and HG + AS-IV + miR-34a-inhibitor groups ().
Figure 3. The effect of AS-IV on cell morphology, autophagy flow and autophagosome in HG-damaged H9C2(2-1) cells. (a–f) Changes in cell morphology under different conditions. (g) Representative images of LC3-GFP adenovirus infection monitored the autophagy flow in H9C2(2-1) cells under fluorescent inverted microscope (500× magnification). (h) Representative images of autophagosome obtained by electron microscope. The red arrows refer to the autophagosomes (First row:Scale bar 1 μm, 18500× magnification; Second row:Scale bar 200 nm, 68000× magnification). (i) Statistical graph of LC3-GFP dots/cell for each group of H9C2(2-1) cells. Each group counts autophagosomes in at least 20 cells. Data are shown as mean ± SEM. ***p < .001, ****p < .0001.
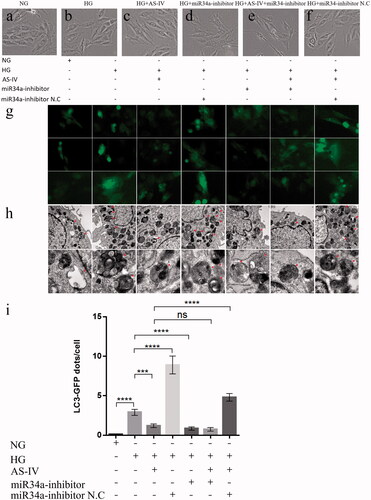
Effect of as-IV on the HG-induced autophagy flow of H9C2(2-1) cells
To determine that AS-IV inhibited autophagy in cells via miR-34a, H9C2(2-1) cells were transfected with the LC3-GFP fluorescence autophagy indicator to label and monitor the changes of LC3 and autophagy flow. The vacuoles were formed in the centre of the cells, and green fluorescence was observed using a fluorescence microscope, indicating that the adenovirus was successfully amplified (). The statistical results of indicate that HG-induced LC3-GFP dots formation was increased in H9C2(2-1) cells compared to the NG group (p < .001). Compared with the HG group, the LC3-GFP dots of HG + miR-34a-inhibitor N.C. were significantly increased (p < .001), and the LC3-GFP dots were significantly decreased after the intervention of AS-IV and miR-34a-inhibitor, respectively (p < .001). Further, the H9C2(2-1) cells were taken for observation of autophagosomes by electron microscope. As shown in , autophagosomes increased in both the HG group and the HG + miR-34a-inhibitor N.C. group, and the addition of AS-IV or miR-34a-inhibitor caused the reduction of autophagosomes. In conclusion, these results indicate that overexpression of miR-34a will increase autophagy damage, whereas AS-IV can rescue this situation, demonstrating the role of AS-IV in miR-34a-mediated autophagy.
As-IV reduced HG-induced excessive oxidative stress of H9C2(2-1) cell
Compared with the NG group, the HG group had lower levels of LDH and MDA (p < .001) and higher level of total (T)-SOD (p < .05), indicating HG-induced excessive oxidative stress and damage. Compared with the HG group and HG + AS-IV group, the HG + miR-34a-inhibitor and HG + AS-IV + miR-34a-inhibitor groups had lower levels of LDH and MDA (p < .01) and higher level of T-SOD (p < .01) (, ), indicating that AS-IV inhibited lipid peroxidation and enhanced the antioxidant enzyme activity in the cells.
Figure 4. The effect of AS-IV on oxidative stress factors in HG-damaged H9C2(2-1) cells. X axis: different intervention groups of H9C2(2-1) cells; Y axis: LDH, MDA and SOD contents in cell culture supernatant; *p < .05, **p < .01, ***p < .001.
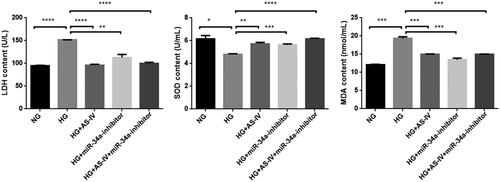
Table 1. Effect of AS-IV on oxidative stress index of H9C2(2-1) cell injury induced by high glucose (Mean ± SEM).
Effect of as-IV on mRNA levels of HG-induced autophagy related factors
Compared with the NG group, HG levels significantly increased miR-34a mRNA transcription and decreased mRNA transcription of Bcl2 and Sirt1 in the HG group. Compared with the HG group, AS-IV and miR-34a-inhibitor decreased miR-34a mRNA transcription and increased Bcl2 and Sirt1 mRNA transcription in the HG + AS-IV, HG + miR-34a-inhibitor, and HG + AS-IV + miR-34a-inhibitor groups in a synergistic manner (). Our results showed that HG levels induced the activation of the Bcl2 and Sirt1 signalling pathway mediated by miR-34a mRNA. Inhibition of Bcl2 and Sirt1 expression at the mRNA level by miR-34a might promote autophagy in a HG state. AS-IV and miR-34a-inhibitor inhibited the over-expression of HG-induced miR-34a at the mRNA level and promoted Bcl2 and Sirt1 expression, that may regulate downstream pathways with antioxidant stress effects and inhibition of autophagy.
Effect of as-IV on the level of autophagy related factor protein
Compared with the NG group, the ratio of LC3II/LC3I in the HG group was higher and the P62 was lower, indicating that HG induced H9C2(2-1) cell autophagy. Compared with HG group, the ratio of LC3II/LC3I in HG + AS-IV group, HG + miR-34a-inhibitor group and HG + AS-IV + miR-34a-inhibitor group was lower, and P62 was higher. Our results showed that AS-IV and miR-34a-inhibitor inhibited H9C2(2-1) cell autophagy induced by HG level (). Compared with the NG group, Bcl2 protein and mRNA expression was significantly decreased, whereas miR-34a expression was increased in the HG group indicating that HG levels promoted H9C2(2-1) cell autophagy through the miR-34a/Bcl2/(LC3II/LC3I) pathway (). Compared with the HG group, Bcl2 expression was increased and miR-34a expression was decreased in the HG + AS-IV, HG + miR-34a-inhibitor, and HG + AS-IV + miR-34a-inhibitor groups, suggesting that AS-IV inhibited H9C2(2-1) cell autophagy induced by HG levels through the miR-34a/Bcl2/(LC3II/LC3I) pathway (). Compared with the NG group, the ratio of pAKT/AKT and Bcl2 protein expressions in the HG group was decreased, indicating that HG levels might inhibit pAKT and Bcl2 protein expression through the pAKT/Bcl2 pathway to promote H9C2(2-1) cell autophagy. Compared with the HG and HG + AS-IV groups, pAKT/AKT and Bcl2 expressions were increased and Caspase 3 protein level was decreased in the HG + miR-34a-inhibitor and HG + AS-IV + miR-34a-inhibitor groups ()), indicating that AS-IV might regulate H9C2(2-1) cell autophagy induced by HG levels via the pAKT/Bcl2 pathway.
Figure 6. The effect of AS-IV on autophagy-related factor protein levels. (a,b) Western blot analysis showing the levels of the autophagy-related factors LC3I, LC3II, P62, Bcl-2, pAKT, AKT, Sirt1 and caspase3. Tubulin was used as loading control. (c,d) Histograms showed the relative proteins expression of LC3II/LC3I ratio and P62 in each intervention group; *p < .05, **p < .01, ***p < .001.
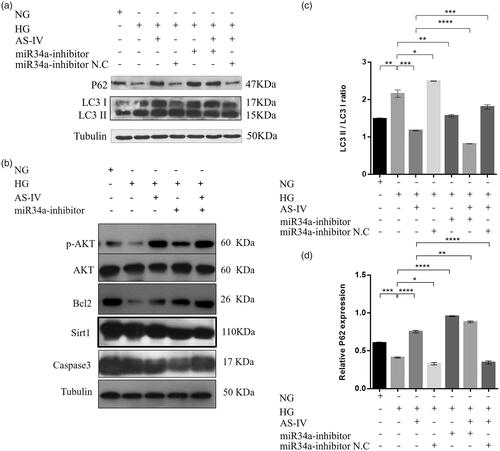
Discussion
miRNAs, as endogenous non-coding RNAs, play an important role in many physiological and pathological mechanisms. miRNAs have become new biomarkers, gene expression regulators, and therapeutic targets for disease because they can regulate cellular metabolic processes [Citation16–18]. miR-34a was originally discovered in retinoblastoma and brain [Citation19] and was found to be involved in immune regulation, cell proliferation and apoptosis [Citation20,Citation21]. The inhibition of miR-34a expression in the heart of adult mice significantly improved cardiac repair and remodelling after myocardial infarction, whereas the miR-34a expression was increased during heart regeneration in neonatal mice [Citation22]. Recently, the miR-34a expression was shown to be increased in prediabetic and diabetic patients [Citation23]. Boon et al. [Citation24] reported that changes in heart miRNAs expression during aging resulted in a decrease in cardiac function, and miR-34a family was significantly up-regulated in the heart of aging mice. MiR-34a gene silencing or knockout reduced aging-related myocardial cell death. The inhibition of miR-34a reduced cell death and fibrosis after acute myocardial infarction and promoted the recovery of myocardial function. Through novel targeting of PNUTS (or PPP1R10) by miR-34a, PPP1R10 mRNA expression could be down-regulated in an age-dependent manner. PNUTS cooperates with telomerase regulator telomeric repeat-binding factor 2 (TRF2) to reduce telomere shortening, DNA damage, and cardiomyocyte apoptosis, and promote the recovery of cardiac function after acute myocardial infarction. These results suggest that inhibiting miR-34a over-expression can regulate the aging process of the heart and promote recovery of cardiac function after myocardial infarction. In vitro and in vivo studies showed that in myocardial cells, including cardiac progenitor cells (CPCs) exposed to the anticancer drug doxorubicin (DOXO), the miR-34a levels were increased. miR-34a silencing reduced miRNA release in DOXO-exposed rat CPCs and this had negative paracrine effects on other neighbouring rat cardiac cells by increasing prosurvival miR-34a and targeting Bcl2 and Sirt1. miR-34a is considered as a circulating biomarker for anthracycline-induced cardiac damage [Citation25]. Our results revealed that miR-34a expression was increased in HG-induced H9C2(2-1) cells damage, suggesting its involvement in HG-induced cardiac cellular damage, whereas AS-IV and miR-34a-inhibitor inhibited miR-34a expression.
In physiological conditions, low levels of autophagy engulf damaged organelles and abnormal proteins to maintain normal cardiac function, whereas cardiac autophagy is significantly increased in a wide range of pathological conditions or in response to stimuli. Thus, regulation of abnormal autophagy may be a potentially important therapeutic target for cardiovascular disease and heart failure [Citation22]. During autophagy, Atg4 cleaves LC3 in the cytoplasm to form cytoplasmic soluble LC3-I, which then conjugates to phosphatidylethanolamine (PE) for the generation of autophagosome membrane-binding LC3-II. LC3-II-/LC3-I is a marker that reflects the degree of autophagy [Citation26–28]. P62, a ubiquitin-binding protein encoded by SQSTML on the autophagosome membrane, selectively recruits ubiquitin-binding proteins and binds to LC3 during autophagy, and transports them for autophagic degradation [Citation29]. P62 markedly accumulates in autophagy-deficient cells, confirming that it is one of the marker proteins that reflect autophagy activity, and its content indirectly reflects the level of autophagosome clearance [Citation30]. In addition, autophagy flow, briefly explained as the process of autophagy, becomes a new indicator to evaluate autophagy [Citation31]. In our experiments, we analyzed the autophagy flow and western blot analysis of autophagy-related proteins. The results showed that LC3-GFP dots and autophagy protein LC3II/LC3I ratio was significantly increased and P62 was significantly decreased in the presence of HG and miR-34a overexpression, while AS-IV can reduce the aggregation of autophagosomes and inhibit the expression of autophagy proteins.
Typically, autophagy (referred to as macroautophagy) is mediated by signalling pathways. miR-34a-mediated autophagy is activated by three downstream target genes: Bcl2, ATG9, and Sirt1 [Citation32,Citation33]. In physiological conditions, Bcl2 protein binds to Beclin 1, but under starvation or oxidative stress, Bcl2 and Beclin1 are separated to release Beclin1-induced autophagy. In breast cancer tissue, miR-34a inhibited Bcl2 expression [Citation33]. Beclin1/BECN 1 (Atg6), as a scaffold protein forming III PI3K (phosphoinositide 3-kinase) complex, binds to Atg9 through the Vps34–p150 complex and forms the PI3K core complex that activates autophagy [Citation34–36]. The imbalance between Bcl2 and Beclin1 can exacerbate autophagy. In this study, HG levels induced overexpression of miR-34 in H9C2 cells and inhibited Bcl2 expression, which may promote Beclin1 protein expression, induce autophagy, and aggravate H9C2 cell injury, with AS-IV and miR-34a-inhibitor antagonizing this process.
miR-34a may regulate autophagy through the Bcl2/Beclin 1-PI3K III pathway. The classical signalling pathway known to regulate autophagy is the mammalian target of rapamycin (mTOR) signalling pathway. The P13K-Akt pathway also activates mTOR, which is a negative regulator of autophagy, thus inhibiting the occurrence of autophagy [Citation37]. In this study, we found that Bcl2 and pAKT/AKT expression was decreased in HG-induced H9C2 cell damage but increased in AS-IV and miR-34a-inhibitor-treated groups. Sirt1 has many substrates that participate in various cell cycle phases by deacetylation. Numerous studies have shown that Sirt1 is involved in mitochondrial protection, antioxidant defence, DNA repair, and genomic stability responses that can prevent cardiomyocyte apoptosis, fibrosis, hypertrophy, and inflammation [Citation38]. Sirt1 is considered a potential target for the treatment cardiovascular diseases, particularly diabetic cardiomyopathy [Citation39]. miR-34a indirectly interferes with the extension of autolysosome by inhibiting Sirt1 [Citation33]. miR-34a has been reported to regulate Sirt1 expression in endothelial progenitor cells (EPCs), whereas atorvastatin up-regulated Sirt1 expression by inhibiting miR-34a. The level of miR-34a is negatively correlated with Sirt1 protein [Citation40]. Our results suggest that miR-34a decreased Sirt1 transcription in the HG group, whereas AS-IV and miR-34a-inhibitor antagonized this effect. Sirt1 protein expression was not significantly different in each group, suggesting that Sirt1 may play its role at the transcription level.
Insulin acts as a signalling factor that activates the P13K-Akt/PKB-mTOR pathway to inhibit autophagy [Citation15,Citation41]. Therefore, in insulin deficiency-related type 1 diabetic cardiomyopathy and insulin resistance-related type 2 diabetic cardiomyopathy, cardiomyocyte autophagy may be activated, along with glucose and lipid metabolism disorders, hyperinsulinemia and other signalling pathway changes. AS-IV has a multi-target mechanism of action, including antioxidative stress. Excessive oxidative stress produces numerous oxygen free radical substances that change mitochondrial function and abnormally increase NADPH oxidase activity, leading to an accelerated myocardial injury in diabetic patients [Citation42]. AS-IV increases the production of nitric oxide (NO) by activating the PI3K/Akt pathway in H9C2 cells and inactivating glycogen synthase kinase 3β (GSK-3β) through the NO/cGMP/PKG signalling pathway to prevent the mitochondrial permeability transition pore (mPTP) from opening and reperfusion injury and reduce myocardial injury [Citation43]. AS-IV has many cardiovascular protective effects, including anti-inflammation, anti-virus, anti-oxidation, inhibition of intracellular calcium overload and foam cell formation, and protection of endothelial cells [Citation4]. In addition, it has protective effects on diabetic nephropathy by inhibiting activation of the MEK1/2-ERK1/2-RSK2 signalling pathway [Citation44]. AS-IV regulates blood glucose metabolism to avoid hyperinsulinemia damage [Citation45]. We consider that AS-IV reduces autophagy injury through the antioxidative stress pathway. AS-IV was found to inhibit DOXO-induced cardiotoxicity by activating the PI3K/Akt pathway [Citation46]. These results are consistent with our study. More research is needed in the future to study these multi-target mechanisms of AS-IV in myocardial protection.
Abbreviations | ||
H9c2(2-1) | = | Rat cardiomyocytes H9c2(2-1) |
PI3K | = | phosphoinositide 3-kinase |
Atg | = | autophagy-related |
PAS | = | pre-autophagosomal structures |
Sirt1 | = | silent mating type information regulation 2 homologue 1 |
LC3 | = | microtubule-associated protein 1 light chain 3 |
AS-IV | = | Astragaloside-IV |
MEK1/2 | = | mitogen-activated protein kinase 1/2 |
ERK1/2 | = | extracellular signal-regulated kinases 1 and 2 |
RSK2 | = | ribosomal S6 kinase 2 |
LDH | = | lactate dehydrogenase |
MDA | = | malondialdehyde |
SOD | = | superoxide dismutase |
Disclosure statement
No potential conflict of interest was reported by the authors.
Additional information
Funding
References
- Li L, Hou X, Xu R, et al. Research review on the pharmacological effects of astragaloside IV. Fundam Clin Pharmacol. 2017;31(1):17–36.
- Du J, Liu J, Zhen J, et al. Astragaloside IV protects cardiomyocytes from hypoxia-induced injury by down-regulation of lncRNA GAS5. Biomed Pharmacother. 2019;116:109028.
- Wang SG, Xu Y, Xie H, et al. AS-IV prevents lipopolysaccharide-induced injury in H9C2 cardiomyocytes. Chin J Nat Med. 2015;13(2):127–132.
- Zheng Q, Zhu JZ, Bao XY, et al. A preclinical systematic review and meta-analysis of AS-IV for myocardial ischemia/reperfusion injury. Front Physiol. 2018;9:795.
- Russell J, Du Toit EF, Peart JN, et al. Headrick. Myocyte membrane and microdomain modifications in diabetes: determinants of ischemic tolerance and cardioprotection. Cardiovasc Diabetol. 2017;16(1):155.
- Hölscher ME, Bode C, Bugger H. Diabetic cardiomyopathy: does the type of diabetes matter? IJMS. 2016;17(12):2136.
- Yang KC, Yamada KA, Patel AY, et al. Deep RNA sequencing reveals dynamic regulation of myocardial noncoding RNAs in failing human heart and remodeling with mechanical circulatory support. Circulation. 2014;129(9):1009–1021.
- Sun T, Li MY, Li PF, et al. MicroRNAs in cardiac autophagy: small molecules and big role. Cells. 2018;7(8):104
- Bei Y, Tao L, Cretoiu D, et al. MicroRNAs mediate beneficial effects of exercise in heart. Adv Exp Med Biol. 2017;1000:261–280.
- Tao H, Song ZY, Ding XS, et al. LncRNAs and miRs as epigenetic signatures in diabetic cardiac fibrosis: new advances and perspectives. Endocrine. 2018;62(2):281–291.
- de la Ballina LR, Munson MJ, Simonsen A. Lipids and lipid-binding proteins in selective autophagy. J Mol Biol. 2019;pii:S0022-2836(19):30352–30353.
- Mellor KM, Bell JR, Young MJ, et al. Myocardial autophagy activation and suppressed survival signaling is associated with insulin resistance in fructose-fed mice. J Mol Cell Cardiol. 2011;50(6):1035–1043.
- Guan Y, Zhou L, Zhang Y, et al. Effects of PP2A/Nrf2 on experimental diabetes mellitus-related cardiomyopathy by regulation of autophagy and apoptosis through ROS dependent pathway. Cell Signal. 2019;62:109339.
- Sciarretta S, Hariharan N, Monden Y, et al. Is autophagy in response to ischemia and reperfusion protective or detrimental for the heart? Pediatr Cardiol. 2011;32(3):275–281.
- Barlow AD, Thomas DC. Autophagy in diabetes: β-cell dysfunction, insulin resistance, and complications. DNA Cell Biol. 2015;34(4):252–260.
- Rusanova I, Fernández-Martínez J, Fernández-Ortiz M, et al. Involvement of plasma miRNAs, muscle miRNAs and mitochondrial miRNAs in the pathophysiology of frailty. Exp Gerontol. 2019;124:110637.
- Brindley E, Hill TDM, Henshall DC. MicroRNAs as biomarkers and treatment targets in status epilepticus. Epilepsy Behav. 2019;3:pii: S1525-5050(19)30353-1.
- Subramaniam S, Jeet V, Clements JA, et al. Emergence of microRNAs as key players in cancer cell metabolism. Clin Chem. 2019;17:pii: clinchem.2018.299651.
- Lagos-Quintana M, Rauhut R, Yalcin A, et al. Identification of tissue-specific microRNAs from mouse. Curr Biol. 2002;12(9):735–739.
- Lv J, Zhang Z, Pan L, et al. MicroRNA-34/449 family and viral infections. Virus Res. 2019;260:1–6.
- Angelini F, Pagano F, Bordin A, et al. Getting old through the blood: circulating molecules in aging and senescence of cardiovascular regenerative cells. Front Cardiovasc Med. 2017;4:62.
- Yang Y, Cheng HW, Qiu Y, et al. MicroRNA-34a plays a key role in cardiac repair and regeneration following myocardial infarction. Circ Res. 2015;117(5):450–459.
- Kong L, Zhu J, Han W, et al. Significance of serum microRNAs in pre-diabetes and newly diagnosed type 2 diabetes: a clinical study. Acta Diabetol. 2011;48(1):61–69.
- Boon RA, Iekushi K, Lechner S, et al. MicroRNA-34a regulates cardiac ageing and function. Nature. 2013;495(7439):107–110.
- Piegari E, Russo R, Cappetta D, et al. MicroRNA-34a regulates doxorubicin-induced cardiotoxicity in rat. Oncotarget. 2016;7(38):62312–62326.
- Ogata M, Hino S, Saito A, et al. Autophagy is activated for cell survival after endoplasmic reticulum stress. Mol Biol Cell. 2006;26(24):9220–9231.
- Berardi DE, Campodónico PB, Díaz Bessone MI, et al. Autophagy: friend or foe in breast cancer development, progression, and treatment. Int J Breast Cancer. 2011;2011:1.
- Zou M, Lu N, Hu C, et al. Beclin 1-mediated autophagy in hepatocellular carcinoma cells: implication in anticancer efficiency of oroxylin A via inhibition of mTOR signaling. Cell Signal. 2012;24(8):1722–1732.
- Harper JW, Schulman BA. Structural complexity in ubiquitin recognition. Cell. 2006;124(6):1133–1136.
- Danieli A, Martens S. p62-mediated phase separation at the intersection of the ubiquitin-proteasome system and autophagy. J Cell Sci. 2018;131(19):pii:jcs214304.
- Klionsky DJ, Abdelmohsen K, Abe A, et al. Guidelines for the use and interpretation of assays for monitoring autophagy (3rd edition). Autophagy. 2016;12(1):1–222.
- Li L, Yuan L, Luo J, et al. MiR-34a inhibits proliferation and migration of breast cancer through down-regulation of Bcl-2 and SIRT1. Clin Exp Med. 2013;13(2):109–117.
- Yang J, Chen D, He Y, et al. MiR-34 modulates Caenorhabditis elegans lifespan via repressing the autophagy gene atg9. Age (Dordr). 2013;35(1):11–22.
- Koukourakis MI, Giatromanolaki A, Sivridis E, et al. Beclin 1 over- and underexpression in colorectal cancer: distinct patterns relate to prognosis and tumour hypoxia. Br J Cancer. 2010;103(8):1209–1214.
- Maejima Y, Kyoi S, Zhai P, et al. Mst1 inhibits autophagy by promoting the interaction between Beclin1 and Bcl-2. Nat Med. 2013;19(11):1478–1488.
- Russell RC, Tian Y, Yuan H, et al. ULK1 induces autophagy by phosphorylating Beclin-1 and activating VPS34 lipid kinase. Nat Cell Biol. 2013;15(7):741–750.
- Kubli DA, Gustafsso AB. Unbreak my heart: targeting mitochondrial autophagy in diabetic cardiomyopathy. Antioxid Redox Signal. 2015;22(17):1527–1544.
- Karbasforooshan H, Karimi G. The role of SIRT1 in diabetic cardiomyopathy. Biomed Pharmacother. 2017;90:386–392.
- Kitada M, Ogura Y, Monno I, et al. Sirtuins and type 2 diabetes: role in inflammation, oxidative stress, and mitochondrial function. Front Endocrinol. 2019;10:187.
- Tabuchi T, Satoh M, Itoh T, et al. MicroRNA-34a regulates the longevity-associated protein SIRT1 in coronary artery disease: effect of statins on SIRT1 and microRNA-34a expression. Clin Sci (Lond). 2012;123(3):161–171.
- Nnah IC, Wang B, Saqcena C, et al. TFEB-driven endocytosis coordinates MTORC1 signaling and autophagy. Autophagy. 2019;15(1):151–164.
- Dludla PV, Joubert E, Muller CJF, et al. Hyperglycemia-induced oxidative stress and heart disease-cardioprotective effects of rooibos flavonoids and phenylpyruvic acid-2-O-β-D-glucoside. Nutr Metab (Lond). 2017;14:45.
- He Y, Xi J, Zheng H, et al. Astragaloside IV inhibits oxidative stress-induced mitochondrial permeability transition pore opening by inactivating GSK-3β via nitric oxide in H9c2 cardiac cells. Oxid Med Cell Longev. 2012;2012:1.
- Song G, Han P, Sun H, et al. AS-IV ameliorates early diabetic nephropathy by inhibition of MEK1/2-ERK1/2-RSK2 signaling in streptozotocin-induced diabetic mice. J Int Med Res. 2018;46(7):2883–2897.
- He KQ, Li WZ, Chai XQ, et al. AS-IV prevents kidney injury caused by iatrogenic hyperinsulinemia in a streptozotocin-induced diabetic rat model. Int J Mol Med. 2018;41(2):1078–1088.
- Jia Y, Zuo D, Li Z, et al. AS-IV inhibits doxorubicin-induced cardiomyocyte apoptosis mediated by mitochondrial apoptotic pathway via activating the PI3K/Akt pathway. Chem Pharm Bull. 2014;62(1):45–53.