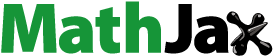
Abstract
The aim of this paper was to utilise an existing in vitro setup to quantify the oxygen offloading capabilities of two different subsets of injectable oxygenation therapeutics: (1) artificial oxygen carriers (AOCs), which bind or dissolve oxygen and act as transport vectors, and (2) kosmotropes, which increase water hydrogen bonding and thereby decrease the resistance to oxygen movement caused by the blood plasma. Dodecafluoropentane emulsion (DDFPe) was chosen to represent the AOC subset while trans sodium crocetinate (TSC) was selected to represent the kosmotrope subset. PEG-Telomer-B (PTB), the surfactant utilised to encapsulate DDFP in emulsion form, was also tested to determine whether it affected the oxygen transport ability of DDFPe. The in vitro set-up was used to simulate a semi closed-loop circulatory system, in which oxygen could be delivered from the lungs to hypoxic tissues. Results of this study showed that (1) 0.5 ml of a PFC outperformed 6.25 ml of a kosmotrope in a controlled, in vitro setting and (2) that PTB and sucrose do not contribute to the overall oxygen transportation efficacy of DDFPe. These results could be therapeutically beneficial to ongoing and future pre-clinical and clinical studies involving various oxygenation agents.
Introduction
With respect to a variety of acute medical conditions including traumatic brain injury [Citation1–3], haemorrhagic shock [Citation2,Citation4], stroke [Citation5,Citation6], and acute respiratory distress syndrome, intravenous therapeutics that are able to facilitate respiratory gas exchange have become a promising avenue for treatment as post-onset prophylactics. Though these injectable therapeutics serve to restore adequate oxygen delivery after acute hypoxic injury, the mechanism through which they facilitate tissue oxygenation can greatly vary. One subset of these oxygenation therapeutics is known as artificial oxygen carriers (AOCs), which physically carry and transport oxygen (O2) by either binding or dissolving diatomic O2 molecules. AOCs found their origin as haemoglobin based oxygen carrier solutions, due to the stability and efficacy of haemoglobin as an O2 carrier, and the field grew from there [Citation7,Citation8]. These O2 carriers are efficient drug delivery systems in that they are cyclic transport vectors and can repeatedly load and unload O2 for as long as they remain in circulation in vivo. Moreover, in lower O2 partial pressure environments, AOCs with high O2 binding affinities may deliver even more O2 than red blood cells (RBCs) [Citation7].
Within this field of AOCs, perfluorocarbons (PFCs) have a long-standing history of use and exploration as RBC substitutes. PFCs are highly fluorinated carbon chains with an ideal gas like chemical inertness, which allows for the dissolution of similarly inert gasses, such as O2, CO2, N2 and NO, into stable CF3 pockets formed by adjacent PFC molecules [Citation9]. The efficacy of PFCs results from their increased O2 delivery capability, which takes priority over O2 absorption ability in vivo [Citation10]. Consequently, although PFCs, which dissolve O2, possess a lower O2 affinity than RBCs, which physically bind O2, the combination of this lower O2 affinity in conjunction with higher diffusion rates, creates a reservoir of O2 that is more readily available for extraction by the tissues [Citation11]. Additionally, PFCs preferentially dissolve CO2 over O2, which could aid in the targeted oxygenation of hypoxic tissues primarily undergoing anaerobic respiration and consequently, producing large quantities of CO2 [Citation11,Citation12]. In order to be administered intravenously as an effective therapeutic, PFCs must be encapsulated as a microbubble or nanodroplet in a lipid monolayer and stabilised in an emulsion—a process that has continuously been refined over decades. When improving the efficacy of PFCs, two main variables must be considered: (1) the PFC itself and (2) the PFC encapsulation surfactant. For this reason, third generation emulsions have focussed on optimising the O2 carrying capacity of PFCs, by increasing the CF3 group density [Citation13] while decreasing the molecular weight and boiling point [Citation14], and utilising surfactants such as PEG-Telomer-B (PTB), which decrease the reactivity of the PFC with surrounding material, allowing for increased shelf life and stability during in vivo circulation.
Another subset of oxygenation therapeutics is known as kosmotropes. These are unique order-inducing chemical compounds that increase protein stability, reduce hydrophobic molecule stability, and form strong hydrogen bonds with water [Citation15]. The mechanism of action of kosmotropes, with respect to O2 delivery, is based on studies that identify blood plasma as accounting for nearly 70% of the resistance to O2 movement experienced as O2 diffuses from RBCs to tissue [Citation16,Citation17]. Due to their hydrophobic intermolecular forces, kosmotropes can form strong hydrogen bonds with surrounding water molecules, which make the water more structured on a microscopic scale, thereby decreasing its density. This physical change “opens up” the water phase to facilitate rapid O2 diffusion and decrease plasma resistivity [Citation18].
The aim of this experiment was to utilise an existing in vitro setup [Citation12], capable of evaluating the O2 offloading ability of a drug product in a hypoxic environment, to assess the relative efficacies of these two subsets of injectable oxygenation therapeutics in vitro. For the purposes of this experiment, dodecafluoropentane emulsion (DDFPe) was chosen to represent the AOC subset while trans sodium crocetinate (TSC) was selected to represent the kosmotrope subset. PTB, the surfactant used in DDFPe, was also tested to determine whether it affected the O2 transport ability of DDFPe. The utilised setup allows for the simulation of O2 transfer from the lungs to hypoxic tissues through a semi-closed circulatory system, with 0.9% saline serving as a blood plasma substitute. Testing drug products through such a setup allows for the evaluation of the relative efficacy of O2 delivery while accounting for the effects of cyclic oxygenation and de-oxygenation of “blood” as seen in biological circulatory systems, as well as the additional pressures exerted by a semi-closed circulatory system [Citation12].
Materials and methods
The in vitro set-up illustrated in , established by Jayaraman et al. [Citation12], was designed to simulate gas exchange and O2 delivery from the lungs to hypoxic tissues. The O2 offloading capability of various stabilised solutions including DDFPe, TSC, and PTB was measured through a series of assays () utilising the set-up depicted in .
Figure 1. Experimental set-up. In vitro oxygenation set-up established by Jayaraman et al. [Citation12], used to simulate gas exchange and oxygen delivery from the lungs (fluid reservoir) to hypoxic tissues (gas exchange vessel).
![Figure 1. Experimental set-up. In vitro oxygenation set-up established by Jayaraman et al. [Citation12], used to simulate gas exchange and oxygen delivery from the lungs (fluid reservoir) to hypoxic tissues (gas exchange vessel).](/cms/asset/4465bb24-fab0-4a91-a654-643145762d3f/ianb_a_1879103_f0001_c.jpg)
Table 1. Table of assays.
Preparation of product
Sodium chloride was purchased from Sigma Aldrich. Sodium sulphite was purchased from J.T. Baker. Purified water (18MΩ·cm) from an in-house purification system was used as the diluent. Dissolved O2 measurements were obtained using an Oakton DO110 metre. Silastic tubing (1.47 mm I.D. × 1.96 mm O.D.) was purchased from VWR. The finished medicinal product, 2% w/v dodecafluoropentane emulsion (DDFPe), manufactured by NuvOx Pharma (Tucson, AZ) was used [Citation19]. Specifically, a 30% sucrose solution was homogenised along with PTB and DDFP. The emulsion was processed using a semi-sealed, stainless steel containment system attached to an Avestin Emulsiflex-C50 homogeniser keeping the temperature below 8 °C. The homogenates were then subject to terminal sterile filtration immediately prior to filling into 10 ml vials. Particle sizing by Nycomp showed a mean particle size of approximately 250 nm. The PTB + Sucrose solution was prepared by adding 0.779 g of purified PTB into 150 ml of a buffered sugar solution composed of sodium phosphate (Sigma Aldrich) and sucrose (Emprove Low Endotoxin Sucrose). Lastly, the stabilised TSC solution was prepared in accordance with the referenced United States Patent 6,060,511 by combining TSC with a mixture of 8% cyclodextrin (Sigma Aldrich) and 2.3% mannitol (Sigma Aldrich) [Citation20].
In vitro performance testing
In vitro model
The in vitro oxygenation experimental setup has previously been described in detail by Jayaraman et al. [Citation12]. The setup was designed to simulate the O2 uptake by the blood in the lungs, the transport of O2 by the blood to the tissues, and finally, the uptake of O2 by the tissues from the blood. The fluid reservoir (80 ml of 0.9% saline solution) represented O2 uptake while the gas exchange vessel (900 ml of 0.9% saline solution) was purposed for O2 offloading (see ). Silastic™ tubing placed in the gas exchange vessel simulated the circulatory system by permitting gas exchange and offloading O2 to areas of low O2 concentration. Sparging with nitrogen gas was used to lower the dissolved O2 concentration of saline in the gas exchange vessel to approximately 1.75 mg/l. A continuous supply of nitrogen (2.0 standard ft3/minute) was also pumped into the headspace of the gas exchange vessel throughout the experiment to displace air since the vessel was not completely sealed. Once the sparging process was complete, the solution in the fluid reservoir received a constant influx of O2 (2.0 l/min). Fluid was pumped out of the reservoir through the silastic tubing with a peristaltic pump at a rate of 30 ml/min. A galvanic dissolved oxygen (DO) sensing probe (DO 110 m, Oakton Instruments), which utilises a Clark electrode to assess the dissolved O2 concentration, was used to monitor the O2 concentration within the gas exchange vessel, which was recalibrated each day. Each assay was conducted over a 45-min duration at ∼21 °C.
Mechanism of action study
About 0.5 ml of DDFPe was tested against 0.5 ml of 0.9% Saline and 6.25 ml of TSC () to determine what effect the mechanism of action of the injected material has on its respective O2 transportation abilities. The doses of DDFPe and TSC tested were determined from clinical literature [Citation18,Citation21] for an assumed 80 kg individual with 5 l of blood, which translated to 80 ml of saline in the fluid reservoir (). These assays were conducted under the same experimental conditions as mentioned above. It is important to note, however, that the TSC solution experimentation was performed in a dark environment to minimise potential photodegradation.
Artificial oxygen carrier (DDFPe) vs kosmotrope (TSC) equivalent dosage calculations:
Assumptions:
Average human blood volume = 5 l
Weight of patient = 80 kg
DDFPe clinical dose: 0.10 ml/kg (2% w/v DDFP) [Citation21]
Plan to use 0.5 ml DDFPe in the in vitro set up:
Scaling factor (ratio of in vitro to clinical dilution for 1 l):
TSC clinical dose: 25 mg TSC/kg [Citation18]
Calculate amount of TSC for in vitro set up based on 3.906 scaling factor above:
Solve for × using TSC clinical dilution for 1 l:
Clinical and in vitro DDFPe and TSC dose comparison [Citation22]:
To account for most effective usage of material, a 0.5 ml dose of DDFPe was selected for experimentation. The scaling factor of the clinical dilution to the in vitro dilution of DDFPe was calculated to be 3.906. This scaling factor of 3.906 was then used to determine the equivalent in vitro dilution of TSC based on the current clinical dilution of TSC (20 ml TSC/1 l blood). This equivalent in vitro dilution of TSC was determined to be 6.25 ml TSC for experimentation. The clinical and in vitro dosages of DDFPe and TSC listed under the heading “Clinical and In Vitro DDFPe and TSC Dose Comparison” are meant to depict the differences in the magnitude of active ingredient administered with respect to each AOC.
PTB + sucrose control study
About 0.5 ml of a PTB + Sucrose solution was tested against 0.5 ml of DDFPe and 0.5 ml of 0.9% Saline () to observe whether the O2 offloading behaviour of DDPFe was affected by the presence of PTB and Sucrose in the emulsion during testing. These assays were conducted under the same experimental conditions as mentioned above.
Statistical analysis
A statistical analysis to determine the significance of experimental results was conducted on Excel via a two-tailed, two-sample unequal variance (heteroscedastic) t-test. A heteroscedastic t-test was chosen since the compared samples had different variances and sample sizes, and were observed and analysed from independent experimental runs. Data are expressed as means ± standard error of mean (SEM). Significance was defined as p ≤ .05.
Results
Mechanism of action study
displays the O2 offloading data for assays 1–4 (). Assay 1, the saline control run, exhibited the lowest net O2 increase, while assay 2, the DDFPe experimental run, resulted in the greatest net O2 increase within the gas exchange vessel. The “net” increase or decrease refers to the total change in the O2 concentration as observed from the beginning to the end of the experimental run. In comparing the magnitudes of O2 transfer of DDFPe and TSC over the course of a 45-min run, an injection of 0.5 ml of DDFPe resulted in a net O2 concentration increase of 5.95 mg/l ± 0.283 mg/l, while an injection of 6.25 ml of TSC solution resulted in a net increase of 4.59 mg/l ± 0.187 mg/l. A standard t-test was used to verify the statistical significance of these results (p-value = .03798).
Figure 2. Net oxygen offloading in mechanism of action comparative study. The net increase in oxygen concentration in the gas exchange vessel for assays 1–4 (). The error bars displayed reflect the SEM. The statistical analysis conducted was a two-tailed, two-sample unequal variance (heteroscedastic) t-test. p-Values are 0.01520, 0.03798, 0.03208 for Saline vs. DDFPe, DDFPe vs TSC, and Saline vs TSC, respectively. For the PTB + Sucrose Control study, p-values are 0.01472 and 0.57763 for DDFPe vs PTB + Sucrose, and Saline vs PTB + Sucrose, respectively.
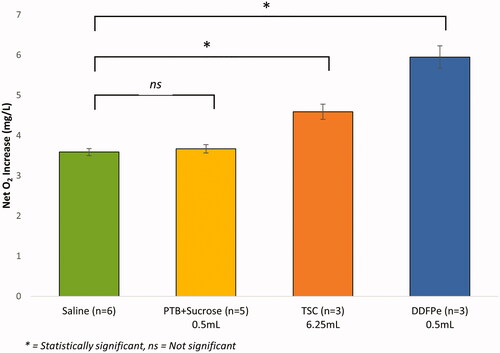
When assessing the mechanism of oxygenation observed in assays 1–3, displays the average oxygenation curves for the tested compounds over the course of 45-min. Based on , it appears that both DDFPe and TSC display a logarithmic oxygenation behaviour, with neither compound having reached its maxima within the 45-min period. However, the relative slopes of the curves imply that, given sufficient time, the maximal oxygenation potential of TSC is most likely lower than that of DDFPe.
PTB + sucrose control study
displays the O2 offloading data for assays 1, 2, 3, and 4 (). Assay 2, the DDFPe experimental run, resulted in the greatest net O2 increase within the gas exchange vessel, while assay 1, the saline control run, exhibited the lowest net O2 increase. The PTB + Sucrose solution (Assay 4) exhibited no significant change in oxygenation when compared to saline over the course of 45 min. The net increase in O2 concentration resulting from administration of DDFPe, PTB + Sucrose, and Saline were 5.95 ± 0.283 mg/l mg/l, 3.67 mg/l ± 0.103 mg/l, and 3.59 mg/l ± 0.088 mg/l, respectively.
Discussion
The results shown in demonstrate that, within the context of this in vitro simulation of hypoxia, AOCs appear to facilitate greater magnitudes of O2 transfer at lower doses than kosmotropes. However, both compounds display statistically significant improvements in oxygenation when compared to the 0.9% saline control. Furthermore, the results in suggest that PTB and sucrose do not contribute to the overall O2 transportation efficacy of DDFPe.
The diffusion of O2 in vivo follows Fick’s Law, which explains that the rate of O2 diffusion is dictated by three variables: (1) the plasma thickness, which refers to the distance that O2 must diffuse over in order to reach the target cells, (2) the O2 concentration gradient, and (3) the diffusion coefficient or diffusivity of O2 [Citation23]. However, plasma thickness is physiologically determined by the anatomy of the vasculature. Therefore, injectable oxygenation therapeutics seek to either increase the O2 concentration gradient or increase the diffusion coefficient in vivo to encourage greater magnitudes of O2 diffusion. PFCs fit into the former mechanism, while kosmotropes fit into the latter [Citation24]. further illustrates this concept of how PFCs and kosmotropes theoretically affect normal O2 transport in vivo.
Figure 4. Artificial oxygen carrier vs. kosmotrope mechanism of action. Illustration of theorised oxygen transport mechanism under normal physiological conditions (A), in the presence of PFC based AOCs (B), and in the presence of kosmotropic compounds (C). RBCs flow through the vasculature and release O2 in accordance with local pO2 gradients. The lighter red colour within the vasculature depicts blood plasma, while the red and blue membrane depicts a cell membrane surrounding the cell interior into which O2 must diffuse.
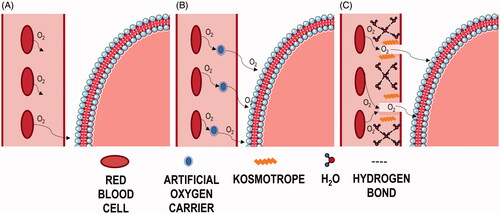
Under normal physiological conditions, O2 transport is dictated by the Bohr Effect, which describes how haemoglobin cooperatively binds O2 in higher pH environments and releases O2 in lower pH environments caused by increased CO2 tension [Citation25]. The O2 released by RBCs then passively diffuses through the blood plasma, based on oxygen tension (pO2) gradients, in order to reach the cell membrane [Citation26]—this is the major rate limiting step. At physiological pO2, oxygen possesses an extremely low solubility in plasma. Furthermore, the O2 extraction ratio (OER), which describes the extent to which RBCs release bound O2, is only ∼25% at rest. This indicates that under normal conditions only 25% of bound O2 is released into the plasma, and even under conditions of hypoxia and exceptional metabolic stress, this ratio rarely, if ever, exceeds 75% [Citation25]. This is in part due to an unstirred, stagnant boundary layer of plasma that forms as a result of the turbulent flow through arterioles and capillaries [Citation16,Citation17]. These layers surrounding RBCs create local O2 concentration gradients of lesser magnitude than that of the overall RBC to tissue O2 concentration gradient, and therefore (1) limit the extent of O2 released by RBCs and (2) increase the distance over which O2 molecules must diffuse in order to reach the cell surface. depicts O2 transport and diffusion under normal physiology, which is limited by low solubility and high resistivity experienced in blood plasma. Consequently, although an OER of 25% is sufficient for normal physiological function, in the event of an acute hypoxic event, even an elevated OER of 75% is often not enough to prevent tissue damage without the aid of molecules to facilitate transport through the plasma.
depicts O2 transport and diffusion in the presence of PFC based artificial O2 carriers. It was previously theorised that PFCs act as RBC substitutes due to their high affinity for O2 without the trade-off of reduced bioavailability. However, in terms of mathematical modelling, the effects observed after administration of DDFPe far outweigh what is expected given the small dosage [Citation10]. Consequently, it is more probable that PFCs act in conjunction with circulating RBCs to improve the efficiency of O2 transport, as opposed to acting independently as “superior RBCs”. As seen in , PFCs presumably act as an intermediate transport vessel for released O2 in the vasculature. It is theorised that they improve the efficiency of O2 transfer by increasing local O2 gradients caused by unstirred layers, which in turn encourages RBCs to readily release more O2. Circulating PFCs then dissolve released O2 and facilitate O2 transfer through the resistive plasma, and once at the tissue surface, the weak intermolecular forces experienced between PFCs and dissolved gases, and the larger surface-to-volume ratio of PFCs, are more favourable for gas exchange [Citation12]. The results observed using this in vitro setup support that a small dose of PFC can demonstrate a significant increase in oxygenation.
Kosmotropes, contrastingly, facilitate O2 transport by increasing the diffusion constant, also known as the diffusivity, of O2 in plasma. They do so by decreasing the entropy of water molecules in the plasma, which thereby reduces the plasma density and decreases the resistance faced by O2 molecules [Citation18]. As previously mentioned, blood plasma is comprised of nearly 92% water. The intrinsic structure of water, and by extension plasma, is due to hydrogen bonds formed between adjacent H2O molecules. Theoretically, a single water molecule should be able to form up to four hydrogen bonds simultaneously. However, in reality, this number averages between 2 and 3.6 [Citation24]. Kosmotropes are order inducing molecules. TSC, for example, is a large hydrophobic molecule. Consequently, as illustrated in , administration of a kosmotrope, such as TSC, decreases plasma entropy by interacting with similarly hydrophobic plasma components and therefore encourages the formation of additional hydrogen bonds between polar water molecules. This brings the average number of hydrogen bonds per water molecule closer to 4 [Citation24], and this physical change in density “opens up” the water phase of the plasma allowing O2 to diffuse towards the vascular wall more easily [Citation18]. This change in density is illustrated in with the lighter plasma regions through which the O2 is diffusing. This in vitro setup utilised 0.9% saline solution as a plasma substitute in simulating hypoxia. Consequently, the increase in oxygenation observed through these experiments supports that kosmotropes increase the diffusivity of O2 primarily by affecting interactions between water molecules.
Additionally, these results indicate that the use of PTB as a surfactant to encapsulate PFCs does not beneficially nor detrimentally affect the oxygenation abilities of the PFC. The oxygenation capability of PTB was originally tested due to the similarities between the hydrophilic poly(oxyethylene) (POE) regions of Poloxamer 188 and PTB (see region x, ). Poloxamer 188 is a surfactant that has shown success in mitigating the severity of acute chest syndrome episodes in sickle cell anaemia patients [Citation27,Citation28]. However, the mechanism through which Poloxamer 188 improves micro-vascular blood flow is thought to involve binding between the hydrophobic core (see region y, ) of Poloxamer 188 and adjacent hydrophobic RBC and neutrophil cell surface regions, which appears to block extraneous hydrophobic adhesive interactions in the bloodstream and thereby reduce the blood viscosity. This allows the hydrophilic POE chains (see region x and z, ) free to interact with the surrounding media [Citation28]. PTB shares the same hydrophilic POE chain (see region x, ) as Poloxamer 188, however, it lacks the hydrophobic core. Instead, PTB’s chemical composition includes a fluorinated tail (see region y, ). Consequently, based on these in vitro experiments where the hydrophobic silastic tubing is representative of the vasculature, it appears that the chemical similarities between the poly(oxyethylene) hydrophilic units in Poloxamer 188 and PTB, do not amount to the same capabilities of facilitating O2 transfer in vitro. Rather these in vitro results support the supposition that the success of Poloxamer 188 in vivo is mechanistically more accredited to the hydrophobic core of Poloxamer 188 than its hydrophilic chains.
Figure 5. Chemical structure of Poloxamer 188 and PEG-Telomer-B. Comparison of the chemical composition of surfactants (A) Poloxamer 188 and (B) PEG-Telomer-B.
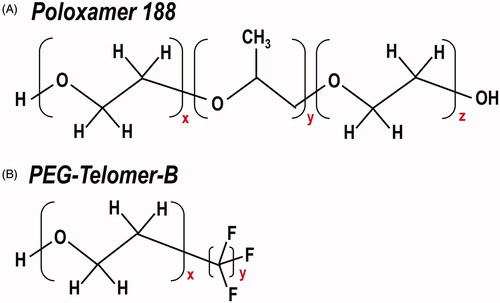
The results of this study show that 0.5 ml (10 mg DDFP) of a PFC outperformed 6.25 ml (125 mg TSC) of a kosmotrope in a controlled, in vitro setting. It should be stated that the numerical O2 offloading concentrations measured in this in vitro model cannot be scaled quantitatively to an in vivo situation due to the physiological limitations of an acellular, in vitro model. However, the trends observed in the in vitro model may still be biologically relevant.
With respect to both PFCs and kosmotropes, it would be pertinent to utilise whole blood in this in vitro model as both classes of molecules rely heavily on blood components mechanistically. In terms of PFCs, in these experiments, it is very likely that DDFPe did not display its full oxygenation potential due to the lack of RBCs present. The use of whole blood, therefore, could account for synergistic oxygenation effects of RBCs in tandem with PFCs. Whereas for kosmotropes, the use of whole blood would create a more representative model of the extent to which these molecules can alter the density of plasma and consequent diffusivity of O2 in vivo. Additionally, the use of whole blood would provide a clearer sense, in general, of how resistive blood plasma is to O2 movement in a simulation of extreme hypoxia. Furthermore, it would be of interest to include a test group in which DDFPe and TSC were administered together. Future in vitro studies will aim to incorporate such cellular components in order to create a more representative in vivo mechanistic model.
Disclosure statement
Meghna Jayaraman is an employee at NuvOx Pharma. Kaitlin Graham is an employee of NuvOx Pharma and owns stock in the company. Dr. Evan Unger is President and CEO of NuvOx Pharma, serves on the Board of Directors, and owns stock in the company. Dr. Unger is also a patent holder of the NuvOx Pharma technology. The authors report no other conflicts of interest in this work.
Data availability statement
All data supporting the results or analyses presented in the paper reside at NuvOx Pharma. For further information please contact the corresponding author.
References
- Spiess BD. Perfluorocarbon emulsions as a promising technology: a review of tissue and vascular gas dynamics. J Appl Physiol. 2009;106(4):1444–1452.
- Graham K, Moon-Massat PF, Unger EC. 2017 Military supplement: dodecafluoropentane emulsion (DDFPe) as a resuscitation fluid for treatment of hemorrhagic shock and traumatic brain injury: a review. Shock. 2019;52:50–54.
- Mullah SH, Saha BK, Abutarboush R, et al. Perfluorocarbon NVX-108 increased cerebral oxygen tension after traumatic brain injury in rats. Brain Res. 2016;1634:132–139.
- Cheung AT, To PL, Chan DM, et al. Comparison of treatment modalities for hemorrhagic shock. Artif Cells Blood Substit Immobil Biotechnol. 2007;35(2):173–190.
- Brown AT, Arthur MC, Nix JS, et al. Dodecafluoropentane emulsion (DDFPe) decreases stroke size and improves neurological scores in a permanent occlusion rat stroke model. Open Neurol J. 2014;8:27–33.
- Culp WC, Woods SD, Skinner RD, et al. Dodecafluoropentane emulsion decreases infarct volume in a rabbit ischemic stroke model. J Vasc Interv Radiol. 2012;23(1):116–121.
- Kawaguchi AT. Artificial oxygen carriers: a clinical point of view. Artif Organs. 2009;33(2):97–99.
- Remy B, Deby-Dupont G, Lamy M. Red blood cell substitutes: fluorocarbon emulsions and haemoglobin solutions. Br Med Bull. 1999;55(1):277–298.
- Riess JG. Understanding the fundamentals of perfluorocarbons and perfluorocarbon emulsions relevant to in vivo oxygen delivery. Artif Cells Blood Substit Immobil Biotechnol. 2005;33(1):47–63.
- Spiess BD. Basic mechanisms of gas transport and past research using perfluorocarbons. Diving Hyperb Med. 2010;40(1):23–28.
- Riess JG, Le Blanc M. Solubility and transport phenomena in perfluorochemicals relevant to blood substitution and other biomedical applications. Pure Appl Chem. 1982;54(12):2383–2406.
- Jayaraman MS, Graham K, Unger EC. In vitro model to compare the oxygen offloading behaviour of dodecafluoropentane emulsion (DDFPe). Artif Cells Nanomed Biotechnol. 2019;47(1):783–789.
- Johnson JL, Dolezal MC, Kerschen A, et al. In vitro comparison of dodecafluoropentane (DDFP), perfluorodecalin (PFD), and perfluoroctylbromide (PFOB) in the facilitation of oxygen exchange. Artif Cells Blood Substit Immobil Biotechnol. 2009;37(4):156–162.
- Johnson JLH. 2017. Oxygen carriers: are they enough for cellular support? In: Lapchak PA, Zhang JH, editors. Neuroprotective therapy for stroke and ischemic disease. Cham: Springer International Publishing. p. 621–640.
- Casanova-Morales N, Alavi Z, Wilson CAM, et al. Identifying chaotropic and kosmotropic agents by nanorheology. J Phys Chem B. 2018;122(14):3754–3759.
- Holland RA, Shibata H, Scheid P, et al. Kinetics of O2 uptake and release by red cells in stopped-flow apparatus: effects of unstirred layer. Respir Physiol. 1985;59(1):71–91.
- Huxley VH, Kutchai H. The effect of the red cell membrane and a diffusion boundary layer on the rate of oxygen uptake by human erythrocytes. J Physiol. 1981;316:75–83.
- Gainer JL, Sheehan JP, Larner JM, et al. Trans sodium crocetinate with temozolomide and radiation therapy for glioblastoma multiforme. J Neurosurg. 2017;126(2):460–466.
- Johnson JL, Unger E. 2014. Buffered oxygen therapeutic. United States Patent 8,822,549, September 2, 2014.
- Gainer JL. 1996. Trans-sodium crocetinate, methods of making and methods of use thereof. United States Patent 6,060,511.
- Culp WC, Onteddu SS, Brown A, et al. Dodecafluoropentane emulsion in acute ischemic stroke: a phase Ib/II randomized and controlled dose-escalation trial. J Vasc Interv Radiol. 2019;30(8):1244–1250 e1241.
- Kreimeier U. Pathophysiology of fluid imbalance. Crit Care. 2000;4(Suppl 2):S3–S7.
- Pittman RN. 2011. Regulation of tissue oxygenation. In: Neil Grange D, Granger JP, editors. Integrated systems physiology: from molecule to function to disease. San Rafael (CA): Morgan & Claypool Life Sciences.
- Gainer JL. Trans-sodium crocetinate for treating hypoxia/ischemia. Expert Opin Investig Drugs. 2008;17(6):917–924.
- Dunn J-OC, Mythen MG, Grocott MP. Physiology of oxygen transport. BJA Education. 2016;16(10):341–348.
- Popel AS. Theory of oxygen transport to tissue. Crit Rev Biomed Eng. 1989;17(3):257–321.
- Sandor B, Marin M, Lapoumeroulie C, et al. Effects of Poloxamer 188 on red blood cell membrane properties in sickle cell anaemia. Br J Haematol. 2016;173(1):145–149.
- Ballas SK, Files B, Luchtman-Jones L, et al. Safety of purified poloxamer 188 in sickle cell disease: phase I study of a non-ionic surfactant in the management of acute chest syndrome. Hemoglobin. 2004;28(2):85–102.