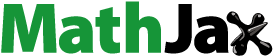
Abstract
Polydopamine (PDA) stands as a versatile material explored in cancer nanomedicine for its unique properties, offering opportunities for multifunctional drug delivery platforms. This study explores the potential of utilizing a one-pot synthesis to concurrently integrate Fe, Gd and Mn ions into porous PDA-based theranostic drug delivery platforms called Ferritis, Gadolinis and Manganis, respectively. Our investigation spans the morphology, magnetic properties, photothermal characteristics and cytotoxicity profiles of those potent nanoformulations. The obtained structures showcase a spherical morphology, robust magnetic response and promising photothermal behaviour. All of the presented nanoparticles (NPs) display pronounced paramagnetism, revealing contrasting potential for MRI imaging. Relaxivity values, a key determinant of contrast efficacy, demonstrated competitive or superior performance compared to established, used contrasting agents. These nanoformulations also exhibited robust photothermal properties under near infra-red irradiation, showcasing their possible application for photothermal therapy of cancer. Our findings provide insights into the potential of metal-doped PDA NPs for cancer theranostics.
Introduction
In the pursuit of advancing cancer diagnostics and therapy, nanoparticles (NPs) have emerged as innovative and versatile tools, promising transformative breakthroughs in personalized medicine [Citation1]. The synergistic integration of diagnostic imaging and therapeutic capabilities within a single nanoplatform, often referred to as theranostics, holds immense promise for addressing the intricacies of cancer treatment [Citation2,Citation3]. These nanoscale systems seamlessly integrate diagnostic and therapeutic functionalities, offering a multifaceted approach to cancer management. Nanotheranostics has opened a realm of possibilities for precise and personalized treatment strategies in the dynamic landscape of cancer therapeutics. One standout modality is photothermal therapy (PTT), a cutting-edge approach harnessing the unique properties of NPs for its therapeutic prowess [Citation4]. PTT relies on converting light into heat, a phenomenon exploited by photoresponsive NPs [Citation5] and other plasmonic materials [Citation6]. Upon exposure to near-infra-red (NIR) light, these NPs efficiently absorb the light energy, rapidly elevating their temperature [Citation7]. This localized hyperthermia becomes a precision weapon, selectively ablating cancer cells while sparing healthy tissues [Citation8]. PTT not only offers targeted and minimally invasive treatment but also holds advantages in terms of reduced side effects and improved patient tolerance.
While PTT emerges as a powerful standalone modality, the concept of combined therapies heralds a new era in cancer treatment. The rationale behind combined therapies lies in their synergistic potential to enhance treatment efficacy, overcome resistance and address cancer heterogeneity. One notable combination involves integrating PTT with other therapeutic modalities, such as chemotherapy [Citation9,Citation10], immunotherapy [Citation11,Citation12] or photodynamic therapy [Citation13,Citation14], creating a multifaceted approach to combat cancer.
The diagnostic modalities of nanotheranostics can serve in a variety of bioimaging techniques, including fluorescent imaging [Citation15,Citation16], computed tomography (CT) [Citation17–19], positron emission tomography (PET) [Citation20,Citation21], photoacoustic imaging [Citation22,Citation23] or even multimodal imaging [Citation24–26]. Magnetic resonance imaging (MRI) stands out as a cornerstone in this multifaceted diagnostic landscape, offering non-invasive, high-resolution imaging capabilities [Citation27]. Beyond anatomical delineation, MRI facilitates real-time monitoring of therapeutic responses, providing a comprehensive approach to cancer management [Citation28]. MRI’s significance lies in its ability to differentiate soft tissues with exceptional contrast, offering detailed insights into organs, muscles and tumours [Citation29]. Integrating multiple imaging sequences in MRI, including T1-weighted, T2-weighted and contrast-enhanced imaging, enables a comprehensive assessment of tissue characteristics and pathology [Citation30]. The strategic incorporation of specific metals, such as iron (Fe), gadolinium (Gd) and manganese (Mn), to modify NPs represents a well-established approach in advancing MRI contrast agents due to their beneficial magnetic properties [Citation31–33]. The incorporation of those specific metals into nanostructures imparts a paramagnetic nature to these entities [Citation34]. Paramagnetism arises from the presence of unpaired electrons in the metal ions, creating localized magnetic moments [Citation35]. In the context of MRI, this paramagnetic property becomes particularly valuable. The contrast agents are employed to enhance the visibility of tissues and improve the differentiation between structures. The presence of such agents induces favourable alterations in the magnetic field around them. This alteration results in an enhancement of the relaxation rates of nearby water protons, influencing both the longitudinal (T1) and transverse (T2) relaxation processes [Citation36]. This heightened contrast is crucial for improving the sensitivity and specificity of the imaging, enabling the visualization of anatomical details and pathological conditions with greater clarity. Therefore, the introduction of paramagnetic metals to nanostructures enhances their utility as effective MRI contrast agents.
In the dynamic landscape of cancer therapeutics, polydopamine (PDA)-based nanoformulations emerge as powerful agents [Citation37]. PDA, known for its biocompatibility and facile modification, has emerged as a promising scaffold for theranostic innovations [Citation38]. The inherent versatility of PDA allows for the chelation of various metals [Citation39,Citation40], enhancing diagnostic imaging capabilities [Citation31,Citation41], while its photothermal properties make it conducive to therapeutic interventions [Citation42,Citation43]. The convergence of imaging modalities, such as MRI, with therapeutic interventions, such as PTT, offers a comprehensive strategy for precision medicine.
In this study, we developed and characterized potential PDA-based theranostic platforms, unravelling the inherent versatility of these nanostructures in accommodating different metal chelates – Fe, Mn and Gd. Although such modifications of PDA nanostructures were already shown [Citation39,Citation41,Citation44–46], we present for the first time a one-pot synthesis of porous PDA NPs enriched with either Fe, Mn or Gd ions. One-pot synthesis facilitates the simultaneous formation of PDA NPs and incorporation of metal ions within a single reaction vessel. This seamless integration ensures homogeneous distribution of metal ions throughout the NP structure, minimizing the risk of uneven metal localization encountered in post-synthesis modifications. The one-pot approach streamlines the synthesis process, eliminating the need for additional steps involved in post-synthesis modifications. This simplification not only reduces the complexity of the procedure but also mitigates the potential challenges associated with purification and separation of intermediates. Coordinated synthesis allows for precise control over the morphology and composition of PDA NPs with embedded metal ions. This level of control is instrumental in tailoring the physical and chemical properties of the NPs, optimizing their performance for specific applications such as imaging and therapy. Moreover, conducting the synthesis in a one-pot fashion optimizes time and resource utilization. By eliminating additional synthesis steps and reducing the number of reaction cycles, one-pot synthesis enhances overall efficiency and economizes the use of reagents and solvents. Additionally, the simultaneous formation of PDA NPs and metal ion integration minimizes the likelihood of NP aggregation during subsequent modification steps. This reduction in aggregation preserves the desired morphology and ensures the stability of the resulting nanostructures. Integrating those metals into the PDA framework enhances the magnetic properties crucial for MRI contrast and showcases the adaptability of PDA-based nanoformulations. Beyond their diagnostic capabilities, our nanoformulations exhibit potent photothermal properties, setting the stage for a comprehensive and integrated approach to cancer therapy. Theranostic NPs, such as PDA containing Fe, Gd or Mn metals (named Ferritis, Gadolinis and Manganis NPs in this study), stand poised to revolutionize cancer therapeutics by providing real-time diagnostics, targeted therapies and the potential for personalized treatment regimens.
Materials and methods
Materials
Gadolinium (III) chloride hexahydrate (99%), iron (III) chloride hexahydrate (98.0–102%), mesitylene (1,3,5-trimethylbenzene, TMB, 97%), Pluronic® F-127, Trizma® base (tris(hydroxymethyl)aminomethane), ≥99.9%, Hank’s balanced salt solution (HBSS) buffer, foetal bovine serum (FBS), antibiotic antimycotic solution (10,000 units penicillin, 10 mg/mL streptomycin, 25 μg/mL amphotericin B were purchased from Merck (Darmstadt, Germany). Dopamine hydrochloride (99%) was purchased from Alfa Aesar (Heysham, UK). Ethanol (99.6%) and acetone (r.g.) were purchased from Avantor Performance Materials Poland S.A. (formerly POCH S.A.; Gliwice, Poland). Trypsin–EDTA (0.25%) solution, cell media Dulbecco’s modified Eagle medium (DMEM 4.5 g/L glucose, l-glutamine and 3.7 g/L sodium bicarbonate, w/o sodium pyruvate), minimum essential medium Eagle (MEM 4.5 g/L glucose, l-glutamine and 3.7 g/L sodium bicarbonate, w/o sodium pyruvate), sodium pyruvate (100 mM), non-essential amino acids solution (100×) were purchased from Thermo Fisher Scientific (Waltham, MA). WST-1 Cell Proliferation Reagent was purchased from Takara Bio (San Jose, CA). Manganese(II) chloride tetrahydrate, 98%, was purchased from Carl Roth GmbH (Karlsruhe, Germany). Uranyl acetate, 98%, was purchased from Polysciences (Warrington, PA).
Cell lines: MRC-5 human lung fibroblast cells and A549 human lung carcinoma epithelial cells were purchased from the American Type Culture Collection (Manassas, VA).
Synthesis of PDA-based nanoparticles
The synthesis procedure was based on previously established protocols [Citation42,Citation47]. Briefly, 0.36 g of Pluronic F127 and 0.36 g of TMB were added to a solution comprising 60 mL of ethanol and 65 mL of Milli-Q water, facilitating template formation for PDA polymerization. After 30 min of mixing, 10 mL of Tris buffer (90 mg) was introduced, followed by 60 mg of dopamine hydrochloride. Subsequently, the mixture was left to react for an additional 18 h at room temperature (RT). Subsequently, the metal ions were introduced as metal sources (FeCl3·6H2O, GdCl3·6H2O or MnCl2·4H2O) into the reaction mixture. The amounts were adjusted to 150 mg of each metal. This resulted in the formation of iron (Ferritis), gadolinium (Gadolinis) and manganese (Manganis) NPs, respectively. The reaction mixture was further mixed for 6 h. The NPs were purified through four rounds of centrifugation and sonication in an ethanol:acetone (2:1 v/v) solution to remove impurities and excess reactants. The obtained nanostructures were stored in ethanol in cold conditions.
TEM imaging and EDS
The samples were prepared using the drop-casting method for transmission electron microscopy (TEM) imaging. A droplet of the NP solution was placed onto a TEM grid (Lacey Formvar/Carbon, 300 mesh, copper, approximately grid hole size: 63 µm). Subsequently, the solvent within the droplet was allowed to evaporate naturally, forming a thin film of NPs on the grid. The images were acquired using Jeol 1400 TEM (JEOL Ltd., Tokyo, Japan), operating at a maximum accelerating voltage of 120 kV. Energy-dispersive X-ray spectroscopy (EDS) analysis was performed using a high-resolution transmission electron microscope (HR-TEM), Jeol ARM 200F (JEOL Ltd., Tokyo, Japan), operating at a maximum accelerating voltage of 200 kV, equipped with an EDX detector.
DLS measurements
The size distribution, polydispersity and zeta potential of the NPs were assessed using the Zetasizer Nano ZS (Malvern Panalytical Ltd., Malvern, UK) with a 173° detector and electrophoretic mobility measurements. The samples were prepared by mixing 5 µL of NPs (1 mg/mL), diluted in 1.45 mL of deionized water. The results represent the arithmetic mean ± standard deviation from three independent measurements.
ICP-OES
Metal quantification was achieved using inductively coupled plasma optical emission spectrometry (ICP-OES). The NP solutions were subjected to drying and then re-dissolved in 65% nitric acid to a concentration of 1 mg/mL. Subsequently, the acidified solution was heated at 50 °C overnight to ensure the complete dissolution of the NPs. The measurements were performed using a Shimadzu ICPE-9820 (Shimadzu, Kyoto, Japan).
SQUID measurements
Magnetic properties of the NPs were evaluated using the magnetic property measurement system (MPMS) with a superconducting quantum interference device (SQUID) as the magnetic field sensor. The samples were prepared as follows: 100 µL of either pure PDA NPs (MPDA), Ferritis, Gadolinis or Manganis NPs in water, each at a concentration of 1 mg/mL, were placed in a polycarbonate measurement capsule. The solution was centrifugated, the supernatant was removed and the resulting pellet was allowed to dry. The magnetic susceptibility was investigated within a temperature range from 2 to 300 K while subjected to a magnetic field of 1 kOe. Magnetization measurements were performed by varying the magnetic field between −70 kOe and 70 kOe at 2 K and 300 K.
Relaxivity evaluation
Relaxivity (r1) evaluation was performed using an NMR spectrometer operating at 16.5 MHz for protons. The measurements were performed on the NPs solutions in water at concentrations ranging from 0.1 to 1 mg/mL, corresponding to concentrations of 0.3–1.7 mM of Fe for Ferritis NPs, 0.67–1.34 mM of Gd for Gadolinis NPs, and 0.39–1.56 mM of Mn for Manganis NPs. Pure water was used as a reference. The measurements were conducted at RT and 37 °C. T1 relaxation times were determined using the inversion-recovery method (180° – τ − 90°), involving the inversion of spin magnetization followed by the observation of its return to the equilibrium state. Subsequently, the NMR T1 relaxation rate (R1) was calculated. Relaxivity values were obtained by fitting the relaxation rate data to the following equation using GraphPad Prism 7 software (La Jolla, CA):
where R1 represents the T1 relaxation rate of the sample, r1 denotes relaxivity, CMe signifies the molar concentration of the metal (Me = Fe, Gd or Mn) and Rwater signifies the T1 relaxation rate of the pure solvent (water).
MRI measurements
The contrasting potential of Ferritis, Gadolinis and Manganis NPs was evaluated using a high-field Agilent MRI scanner operating at 9.4 T (equivalent to 400 MHz for protons). For this evaluation, we utilized six different concentrations of NP solutions in water, ranging from 0.1 to 1 mg/mL, which corresponds to 0.3–1.7 mM of Fe for Ferritis NPs, 0.67–1.34 mM of Gd for Gadolinis NPs, and 0.39–1.56 mM of Mn for Manganis NPs. Pure water served as the control in these experiments. The measurements were performed in both T1-weighted method with inversion-recovery (single-echo multi-slice – SEMS) and T2-weighted method with multiple echos (multi-echo multi-slice – MEMS sequence) modes. The specific experimental parameters employed in these measurements can be found in . T1 and T2 maps were generated and analysed using ImageJ (Bethesda, MD) and Origin software (Irvine, CA).
Table 1. Experimental parameters applied to acquire T1- and T2-weighted images of paramagnetic nanoformulations using an MRI scanner.
Photothermal evaluation
One millilitre of the NP solution in water (concentrations of 10, 25 or 50 µg/mL) was irradiated using an 808 nm NIR laser (Changchun New Industries Optoelectronics Tech. Co., Ltd., Changchun, China). A sample was placed within a quartz cuvette, and the irradiation was performed at a distance of 5 cm from the cuvette using the laser power of 3 W/cm2 for 5 min.
Cell viability
The cell viability studies were performed to assess the biosafety of the NPs using the WST-1 assay. Cells were cultured either in MEM (A549) or DMEM (MRC-5) supplemented with 10% FBS, 1% antibiotics, non-essential amino acids and sodium pyruvate under standard conditions (37 °C, 5% CO2). A549 cells, derived from human lung adenocarcinoma, represent a commonly studied cancer cell line, while MRC-5 cells, derived from human foetal lung fibroblasts, serve as a representative normal cell line for comparison. MRC-5 and A549 cells were seeded onto a 96-well flat-bottom plate. After 24 h, the NP solutions of varying concentrations in water (50 µL per well) were added to the cells. Subsequently, after an additional 48 h, 10 µL of the WST-1 Cell Proliferation Reagent was added to the culture medium. Following a 4-h incubation period, the cell proliferation was evaluated using UV–vis measurements. The measurements were carried out at 450 nm (with a reference wavelength of 620 nm) using a multi-well plate reader (Anthos Zenyth 340rt, Biochrom, Cambridge, UK). Cell viability was expressed as the respiration activity normalized to untreated cells. The measurements were performed in triplicates.
Statistical analysis
Appropriate statistical tests were applied to assess the significance of differences observed among the experimental groups. For parametric data, including normally distributed continuous variables, either the two-sample t-test or analysis of variance (one-way ANOVA) followed by post hoc Tukey’s analysis were applied. A significance level of p < .05 was set to determine statistical significance. All statistical analyses were carried out using GraphPad Prism software (La Jolla, CA).
Results and discussion
Preparation of metal-modified porous PDA nanostructures
Nanoparticles have emerged as versatile platforms with tremendous potential in the field of theranostics, combining therapeutic and diagnostic capabilities into a single entity [Citation48]. These nanoformulations, with their tuneable properties and multifunctionality, offer multiple advantages in the development of advanced theranostic systems. In this study, we report the synthesis and characterization of porous PDA-based NPs modified with different metal ions (Fe, Gd and Mn), aiming to harness their theranostic potential. The synthesis procedure was based on previously established protocols [Citation42,Citation47]. illustrates the TEM images of the obtained NPs. Ferritis NPs exhibit a spherical shape, while Gadolinis and Manganis NPs display additional smaller structures that were challenging to purify, indicating potential differences in their formation processes. presents the DLS measurements of the nanostructures’ hydrodynamic diameter and zeta potential. Ferritis NPs were shown to have the smallest hydrodynamic diameter of 209.5 ± 5 nm, Manganis − 246.2 ± 11 nm while Gadolinis NPs appear to be the largest − 290.0 ± 11 nm – among the three systems. On the other hand, Manganis NPs exhibit the lowest zeta potential (−25.6 ± 1 mV), while Ferritis and Gadolinis NPs possess more uniform potential values (0.9 ± 1 mV and −1.9 ± 1 mV, respectively). The presence of metals within the NPs was confirmed using EDS analysis (), which demonstrated the uniform distribution of the metals throughout the NPs, suggesting their incorporation within the structure and not solely on the surface. All of the reported structures possess visible pores. Porosity is known to enhance the drug loading capability of the nanocarriers compared to nonporous structures [Citation49]. Such a design enables a high capacity for loading multiple therapeutic molecules, including doxorubicin [Citation42,Citation50], temozolomide [Citation51], paclitaxel [Citation52], docetaxel [Citation53,Citation54], siRNA [Citation55] or immune agents [Citation56] for synergistic anticancer treatment. Moreover, the intrinsic reactivity of PDA allows for facile conjugation of targeting ligands, enabling precise delivery to tumour sites while minimizing off-target effects. Various strategies have been explored to functionalize PDA and melanin NPs for tumour targeting, such as antibodies [Citation57–59], peptides [Citation51] and aptamers [Citation60] that specifically recognize receptors overexpressed on tumour cells. Additionally, coating PDA NPs with cell membranes derived from tumour cells or immune cells has emerged as a promising approach to enhance tumour targeting [Citation42,Citation61]. Future research in this field holds great potential for the development of targeted and personalized cancer theranostic systems utilizing PDA-based nanoformulations.
Figure 1. Characterization of Ferritis, Gadolinis and Manganis nanoparticles. (A) TEM images of each type of nanoparticles. (B) DLS characterization of Z-average, PDI (polydispersity index) and zeta potential. All measurements were performed in triplicates, and data are presented as mean ± SD. (C) EDS images displaying metal distribution (Fe, Gd or Mn) throughout the nanoparticles.
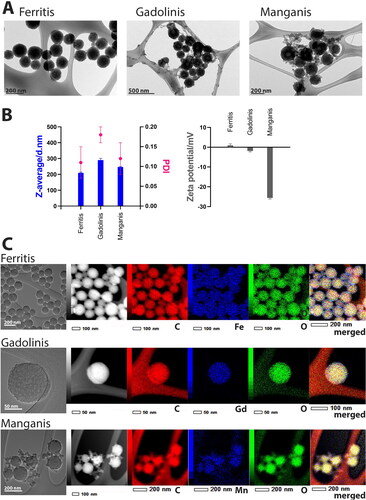
Contrasting potential of PDA-based nanoplatforms
MRI has become an indispensable tool in clinical diagnostics due to its non-invasiveness and high spatial resolution. The magnetic attributes of contrast agents play a pivotal role in enhancing imaging efficacy. The significance of magnetism in the context of MRI lies in its ability to influence the relaxation times of nearby protons in water molecules, thereby improving the contrast of the resulting images. Paramagnetic nanomaterials, characterized by enhanced magnetic susceptibility, contribute to improved contrast in MRI images [Citation62].
The deliberate incorporation of metal components into the synthesis of Ferritis, Gadolinis and Manganis NPs is designed to harness their paramagnetic nature and, consequently, elevating their performance as MRI contrast agents. Through SQUID analysis, we aimed to unravel the unique magnetic signatures of these nanoformulations, thereby providing valuable insights for their potential application in enhancing the precision and sensitivity of MRI imaging. The obtained results established the paramagnetic behaviour of all three nanoformulations. The susceptibility curves () follow the Curie law typical for paramagnets, and the magnetization curves () exhibited the characteristic profile observed for paramagnetic materials. Crucially, the comparative assessment with bare polydopamine (MPDA) reveals that the distinctive paramagnetic magnetic signatures result from the metal introduction. This suggests that the unique paramagnetic contributions of Ferritis, Gadolinis and Manganis NPs and underscores their potential for serving as contrasting agents in MRI, which is consistent with our previous studies’ results [Citation42].
Figure 2. SQUID measurements of MPDA NPs, Ferritis, Gadolinis and Manganis NPs. (A) Magnetic susceptibility. (B) Magnetization at 2 K. (C) Magnetization at 300 K.
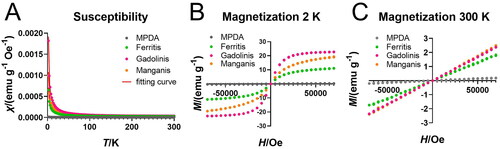
In our investigation of the potential MRI contrasting of the investigated NPs – Ferritis, Gadolinis and Manganis NPs – we have performed a detailed evaluation of their relaxivity, a crucial parameter for assessing their effectiveness as contrast agents in MRI. Relaxivity characterizes the efficiency of a contrast agent in influencing the relaxation times of nearby water protons. The relaxivity values indicate the efficiency of the nanoformulations in influencing proton relaxation times, which is crucial for generating optimal contrast in MRI images. Higher relaxivity implies greater contrast enhancement, making the NPs more effective as contrast agents.
The relaxation times for Ferritis, Gadolinis and Manganis NPs () were systematically measured using a 16.5 MHz NMR spectrometer at both 22 and 37 °C. The calculated relaxivity values, based on the R1 (longitudinal relaxation rate) measurements, were found to be 2.3 s−1 mM−1, around 4 s−1 mM−1, and around 19 s−1 mM−1 for Ferritis, Gadolinis and Manganis NPs, respectively. The MRI imaging experiments further confirmed a great contrasting potential of the developed structures in both T1- (, Tables S1–S3) and T2- (, Tables S1–S3) weighted mode.
Figure 3. Relaxivity (R1) evaluation of (A) Ferritis, (B) Gadolinis and (C) Manganis nanoparticles at 22 and 37 °C measured using a 16.5 MHz (0.4 T) spectrometer.
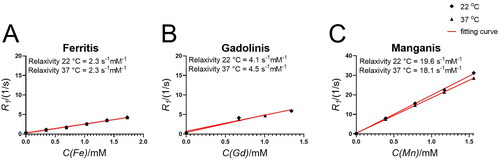
Figure 4. T1 contrasting properties of (A, B) Ferritis, (C, D) Gadolinis and (E, F) Manganis nanoparticles measured using a 9.4 T MRI spectrometer. The left images are T1-weighted images obtained using the MRI scanner at different τ values, whereas the right graphs are the corresponding T1 times extracted from the maps.
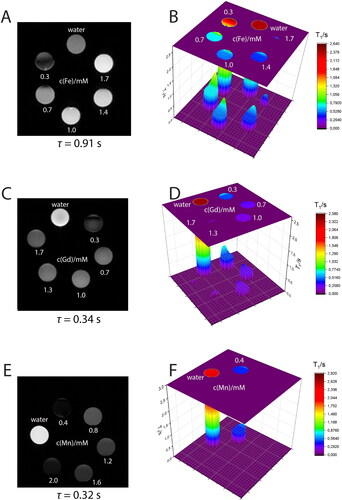
Figure 5. T2 contrasting properties of (A, B) Ferritis, (C, D) Gadolinis and (E, F) Manganis nanoparticles measured using a 9.4 T MRI spectrometer. The left images are T2-weighted images obtained using the MRI scanner, whereas the right graphs are the corresponding T2 times extracted from the maps.
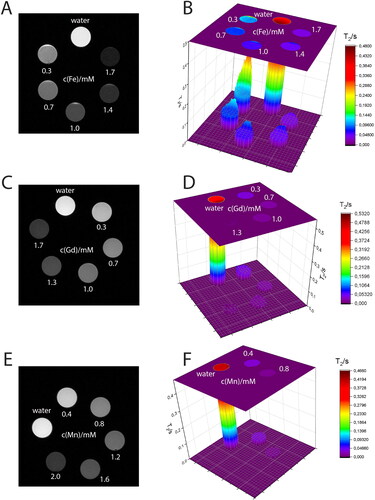
Our nanoformulations exhibit promising relaxivity values compared to commercially available and clinically used contrasting agents. Ferritis and Gadolinis NPs display relaxivity values that are competitive with or exceed those of established contrast agents such as Teslascan (1.9 s−1 mM−1 at 0.47 T [Citation63]), Magnevist (3.4 s−1 mM−1 at 0.47 T [Citation63]) or Prohance (3.1 s−1 mM−1 at 0.47 T [Citation63]). Manganis NPs not only significantly exceed the relaxivity value of the commercially available formulations but also compete with many proposed NP-based MRI contrast agents, such as gadolinium-labelled iron/iron oxide core/shell NPs (7.2 s−1 mM−1 at 0.5 T [Citation64]). The outstanding potential of the synthesized structures is further underscored when compared to other reports on PDA-based MRI contrast agents. Ding et al. introduced Mn-containing NPs (Mn3O4@PDA) with the r1 value of 14.47 s−1 mM−1 at 1.2 T [Citation65]. In a separate study, Wang et al. presented a series of Mn-based PDA NPs with r1 values between 4.7 and 8.3 s−1 mM−1 (measured at 7 T) [Citation44], whereas Fe-based PDA nanosystems demonstrated by Li et al. were reported to have r1 values between 1.2 and 4.6 s−1 mM−1 (measured at 7 T) [Citation45]. However, systems of exceptionally high relaxivity were also reported, including Gd-containing PDA NPs with r1 values from 35.1 to 74.6 s−1 mM−1 (measured at 1.4 T) [Citation66]. Nevertheless, our findings suggest the potential utility of the Ferritis, Gadolinis and Manganis nanoformulations in MRI imaging, demonstrating their capability to enhance image contrast and contribute to the advancement of diagnostic imaging technologies.
Photothermal properties
Photothermal therapy has emerged as a promising approach in cancer treatment, leveraging light-absorbing materials to convert optical energy into heat and induce localized hyperthermia in targeted tissues. PDA has gained attention as an effective photothermal agent owing to its strong light absorption properties in the NIR region. The photothermal properties of PDA and its derivatives make them valuable candidates for applications in cancer therapy, including as carriers for drug delivery and as photothermal agents, which can result in a synergistic anticancer treatment.
This study explored the photothermal properties of the potential theranostic platforms – Ferritis, Gadolinis and Manganis NPs (). Solutions of each NP at concentrations of 50, 100 and 200 µg/mL were irradiated with an 808 nm laser for 5 min. The ensuing temperature changes were recorded using a thermocouple and a thermal camera (full overview of the thermal camera measurements is displayed in Figure S1). Our results demonstrate a concentration-dependent increase in temperature for all three nanoformulations. Notably, the recorded temperature changes exceeded 20 °C, signifying the substantial photothermal efficacy of all the presented nanoformulations. Notably, the similarity in the temperature increase suggests a consistent and reliable photothermal response.
Figure 6. Photothermal evaluation of (A) Ferritis, (B) Gadolinis and (C) Manganis nanoparticles solutions in water measured using a thermocouple.

These findings underscore the potential of our nanosystems for PTT. The concentration-dependent temperature rise observed holds promise for precise and controlled hyperthermia induction in targeted tissues. Moreover, the versatility of PDA-based nanocarriers opens avenues for combining phototherapy with other therapeutic modalities. Examples include synergistic combinations with chemotherapy [Citation53] and immunotherapy [Citation56], showcasing the multifunctional potential of these nanoformulations in advancing therapeutic strategies for various diseases.
Biosafety evaluation
The development of theranostic platforms for cancer treatment and imaging necessitates a comprehensive understanding of the biosafety profile of the employed nanoformulations. In this context, we conducted cytotoxicity studies on MRC-5 (non-cancerous, human fibroblasts) and A549 (cancerous, lung carcinoma) cells, employing the WST-1 assay with a 48-h incubation period. The tested concentration range for each NP spanned from 1.25 to 40 µg/mL.
In the evaluation of MRC-5 cells (), Ferritis, Gadolinis and Manganis NPs exhibited a notable lack of significant toxicity up to a 10 µg/mL concentration. However, at the highest tested concentration (40 µg/mL), cell viability was reduced to approximately 30%. This indicates a concentration-dependent impact on cell viability but highlights a relatively favourable safety profile at lower concentrations. Interestingly, in the case of A549 cells (), Ferritis and Manganis NPs showed similar results. However, Gadolinis NPs exhibited a more pronounced decrease in cell viability compared to the other two nanoformulations, suggesting potential variations in cytotoxic effects among the different NPs. Statistical evaluation of the obtained results is summarized in Table S4.
Figure 7. Cytotoxicity study. WST-1 assay results of Ferritis, Gadolinis and Manganis NPs incubated with (A) normal cells (MRC-5) and (B) cancer cells (A549).
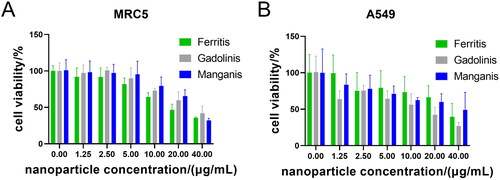
These cytotoxicity findings are essential for assessing the safety of Ferritis, Gadolinis and Manganis NPs as potential theranostic platforms. The concentration-dependent response observed in MRC-5 and A549 cells allows for careful consideration of the dosage range that minimizes cytotoxic effects while maximizing therapeutic efficacy. The nuanced differences in cytotoxicity among the nanoformulations emphasize the importance of tailoring the choice of NPs based on the specific therapeutic and imaging requirements. Nevertheless, the presented biosafety analyses contribute valuable insights, supporting the potential applicability of Ferritis, Gadolinis and Manganis NPs as safe and effective platforms for the integrated treatment and imaging of cancer. Further investigations and optimizations are warranted to refine the therapeutic window and enhance the translational potential of these nanoformulations in clinical settings.
Conclusions
In this comprehensive study, we have successfully developed and characterized multifunctional theranostic platforms employing PDA-based NPs modified with Fe, Gd and Mn, namely Ferritis, Gadolinis and Manganis NPs, respectively. Our investigation spans crucial aspects, including one-pot synthesis of porous PDA-metal NPs, MRI contrast enhancement, PTT and biosafety analysis, culminating in creating promising nanosystems for integrated cancer treatment and imaging. Ferritis NPs, distinguished by a spherical morphology, present an intriguing contrast to the smaller, challenging-to-purify structures observed in Gadolinis and Manganis NPs. The magnetic properties of the nanoformulations were meticulously evaluated, revealing their paramagnetic nature ideal for enhancing MRI contrast. All of the nanoformulations gained their paramagnetic behaviour as a result of enriching PDA with metal ions. While Ferritis NPs showcased the least profound paramagnetic properties, all of the structures demonstrate a distinct magnetic behaviour. Relaxivity values, a key determinant of contrast efficacy, demonstrated competitive or superior performance compared to established, used contrasting agents. This positions Ferritis, Gadolinis and Manganis NPs as viable candidates for improving the precision and sensitivity of MRI imaging; however, the Mn-modified nanostructures showed superior relaxivity values compared to their Fe- and Gd-modified counterparts. Furthermore, our nanoformulations exhibited robust photothermal properties under irradiation, showcasing their potential for PTT of cancer. The concentration-dependent temperature increase highlights the controlled and targeted hyperthermic capabilities of these nanostructures. Importantly, this photothermal efficacy opens avenues for synergistic combinations with other therapeutic modalities. Cytotoxicity studies on non-cancerous (MRC-5) and cancerous (A549) cells provided critical insights into the biosafety profile of the nanoformulations. The obtained results reveal the biocompatibility of Ferritis and Manganis NPs at lower concentrations. Gadolinis NPs, while exhibiting a similar trend with MRC-5 cells, display a more pronounced decrease in cell viability for A549 cells at higher concentrations. While a concentration-dependent reduction in cell viability was observed, especially at higher concentrations, the favourable response at lower doses underscores the potential for a therapeutic window that balances efficacy and safety.
In conclusion, Ferritis, Gadolinis and Manganis NPs represent a promising theranostic platform for cancer treatment and imaging. Their multifunctional attributes, including MRI contrast enhancement and photothermal properties, present opportunities for integrated therapeutic strategies. The observed biosafety profile adds to the translational potential of these nanoformulations, paving the way for further exploration and optimization in the quest for effective and safe theranostic platforms in clinical applications. Moreover, this study marks a significant stride towards developing a potent theranostic platform for cancer treatment and imaging and underscores the remarkable versatility inherent in PDA-based nanoformulations. The successful synthesis and characterization of Ferritis, Gadolinis and Manganis NPs highlight the adaptability of PDA as a scaffold for chelating different metals, adding to the possible avenues for tailored modifications catering to diverse therapeutic needs. The ability to seamlessly integrate various metal components into PDA structures imparts distinct magnetic properties essential for MRI contrast enhancement and opens avenues for expanding the therapeutic repertoire. The observed paramagnetic nature of these nanoformulations, coupled with their robust photothermal properties, showcases the potential for a singular platform capable of combining multiple therapeutic modalities.
Author contributions
Magdalena J. Bigaj-Józefowska: investigation, data curation, methodology, visualization, validation, formal analysis and writing – original draft preparation; Emerson Coy: investigation, data curation and writing – review and editing; Karol Załęski: investigation, data curation and writing – review and editing; Tomasz Zalewski: investigation, data curation and writing – review and editing; Marcin Frankowski: investigation, data curation and writing – review and editing; Radosław Mrówczyński: investigation, data curation and writing – review and editing; Bartosz F. Grześkowiak: conceptualization, investigation, data curation, methodology, validation, writing – original draft preparation, supervision, project administration and funding acquisition.
Supplemental Material
Download MS Word (379.6 KB)Disclosure statement
No potential conflict of interest was reported by the author(s).
Data availability statement
All data generated or analysed during this study are included in this published article (and its Supplementary Information files).
Additional information
Funding
References
- Sur S, Rathore A, Dave V, et al. Recent developments in functionalized polymer nanoparticles for efficient drug delivery system. Nano Struct Nano Objects. 2019;20:100397. doi: 10.1016/j.nanoso.2019.100397.
- Indoria S, Singh V, Hsieh M-F. Recent advances in theranostic polymeric nanoparticles for cancer treatment: a review. Int J Pharm. 2020;582:119314. doi: 10.1016/j.ijpharm.2020.119314.
- Li L, Fu J, Wang X, et al. Biomimetic “nanoplatelets” as a targeted drug delivery platform for breast cancer theranostics. ACS Appl Mater Interfaces. 2021;13(3):3605–3621. doi: 10.1021/acsami.0c19259.
- Shi Y, Liu M, Deng F, et al. Recent progress and development on polymeric nanomaterials for photothermal therapy: a brief overview. J Mater Chem B. 2017;5(2):194–206. doi: 10.1039/C6TB02249A.
- Tian L, Li X, Ji H, et al. Melanin-like nanoparticles: advances in surface modification and tumour photothermal therapy. J Nanobiotechnol. 2022;20(1):485. doi: 10.1186/s12951-022-01698-x.
- Aguilar‐Ferrer D, Vasileiadis T, Iatsunskyi I, et al. Understanding the photothermal and photocatalytic mechanism of polydopamine coated gold nanorods. Adv Funct Mater. 2023;33:1–14. doi: 10.1002/adfm.202304208.
- Beik J, Abed Z, Ghoreishi FS, et al. Nanotechnology in hyperthermia cancer therapy: from fundamental principles to advanced applications. J Control Release. 2016;235:205–221. doi: 10.1016/j.jconrel.2016.05.062.
- Pérez-Hernández M. Mechanisms of cell death induced by optical hyperthermia. In: R. M. Fratila and J. M. De La Fuente (editors). Nanomaterials for magnetic and optical hyperthermia applications. Elsevier; 2019. p. 201–228.
- Su J, Sun H, Meng Q, et al. Bioinspired nanoparticles with NIR‐controlled drug release for synergetic chemophotothermal therapy of metastatic breast cancer. Adv Funct Mater. 2016;26(41):7495–7506. doi: 10.1002/adfm.201603381.
- Phung DC, Nguyen HT, Phuong Tran TT, et al. Combined hyperthermia and chemotherapy as a synergistic anticancer treatment. J Pharm Investig. 2019;49(5):519–526. doi: 10.1007/s40005-019-00431-5.
- Shen J, Lin M, Ding M, et al. Tumor immunosuppressive microenvironment modulating hydrogels for second near-infrared photothermal-immunotherapy of cancer. Mater Today Bio. 2022;16:100416. doi: 10.1016/j.mtbio.2022.100416.
- Zhang G, Chen X, Chen X, et al. Enhanced immunotherapy based on efficient tumor inhibition the synergistic click reaction-mediated chemotherapy and photothermal therapy for efficient tumor inhibition. Biol Med Chem. 2023:1–15. doi: 10.26434/chemrxiv-2023-nl43g.
- Overchuk M, Weersink RA, Wilson BC, et al. Photodynamic and photothermal therapies: synergy opportunities for nanomedicine. ACS Nano. 2023;17(9):7979–8003. doi: 10.1021/acsnano.3c00891.
- Bienia A, Wiecheć-Cudak O, Murzyn AA, et al. Photodynamic therapy and hyperthermia in combination treatment—neglected forces in the fight against cancer. Pharmaceutics. 2021;13(8):1147. doi: 10.3390/pharmaceutics13081147.
- Gao S, Wang G, Qin Z, et al. Oxygen-generating hybrid nanoparticles to enhance fluorescent/photoacoustic/ultrasound imaging guided tumor photodynamic therapy. Biomaterials. 2017;112:324–335. doi: 10.1016/j.biomaterials.2016.10.030.
- Zhang Z, Xu W, Xiao P, et al. Molecular engineering of high-performance aggregation-Induced emission photosensitizers to boost cancer theranostics mediated by acid-triggered nucleus-targeted nanovectors. ACS Nano. 2021;15(6):10689–10699. doi: 10.1021/acsnano.1c03700.
- Kim D, Jeong YY, Jon S. A drug-loaded aptamer–gold nanoparticle bioconjugate for combined CT imaging and therapy of prostate cancer. ACS Nano. 2010;4(7):3689–3696. doi: 10.1021/nn901877h.
- Yang C, Guo C, Guo W, et al. Multifunctional bismuth nanoparticles as theranostic agent for PA/CT imaging and NIR laser-driven photothermal therapy. ACS Appl Nano Mater. 2018;1(2):820–830. doi: 10.1021/acsanm.7b00255.
- Yang J, Xie R, Feng L, et al. Hyperthermia and controllable free radical coenhanced synergistic therapy in hypoxia enabled by near-infrared-II light irradiation. ACS Nano. 2019;13(11):13144–13160. doi: 10.1021/acsnano.9b05985.
- Kamkaew A, Cheng L, Goel S, et al. Cerenkov radiation induced photodynamic therapy using chlorin e6-loaded hollow mesoporous silica nanoparticles. ACS Appl Mater Interfaces. 2016;8(40):26630–26637. doi: 10.1021/acsami.6b10255.
- Chen F, Hong H, Zhang Y, et al. In vivo tumor targeting and image-guided drug delivery with antibody-conjugated, radiolabeled mesoporous silica nanoparticles. ACS Nano. 2013;7(10):9027–9039. doi: 10.1021/nn403617j.
- Lu W, Melancon MP, Xiong C, et al. Effects of photoacoustic imaging and photothermal ablation therapy mediated by targeted hollow gold nanospheres in an orthotopic mouse xenograft model of glioma. Cancer Res. 2011;71(19):6116–6121. doi: 10.1158/0008-5472.CAN-10-4557.
- Krishna V, Singh A, Sharma P, et al. Polyhydroxy fullerenes for non‐invasive cancer imaging and therapy. Small. 2010;6(20):2236–2241. doi: 10.1002/smll.201000847.
- Fernandes DA, Fernandes DD, Malik A, et al. Multifunctional nanoparticles as theranostic agents for therapy and imaging of breast cancer. J Photochem Photobiol B. 2021;218:112110. doi: 10.1016/j.jphotobiol.2020.112110.
- Sharma S, Zvyagin A, Roy I. Theranostic applications of nanoparticle-mediated photoactivated therapies. J Nanotheranost. 2021;2(3):131–156. doi: 10.3390/jnt2030009.
- Lee HJ, Liu Y, Zhao J, et al. In vitro and in vivo mapping of drug release after laser ablation thermal therapy with doxorubicin-loaded hollow gold nanoshells using fluorescence and photoacoustic imaging. J Control Release. 2013;172(1):152–158. doi: 10.1016/j.jconrel.2013.07.020.
- Srinivasan S, Dasgupta A, Chatterjee A, et al. The promise of magnetic resonance imaging in radiation oncology practice in the management of brain, prostate, and GI malignancies. JCO Glob Oncol. 2022;8:e2100366. doi: 10.1200/GO.21.00366.
- Zhao X, Shen R, Bao L, et al. Chitosan derived glycolipid nanoparticles for magnetic resonance imaging guided photodynamic therapy of cancer. Carbohydr Polym. 2020;245:116509. doi: 10.1016/j.carbpol.2020.116509.
- Lau D, Corrie PG, Gallagher FA. MRI techniques for immunotherapy monitoring. J Immunother Cancer. 2022;10(9):e004708. doi: 10.1136/jitc-2022-004708.
- McGowan JC. Basic principles of magnetic resonance imaging. Neuroimaging Clin N Am. 2008;18(4):623–636, x. doi: 10.1016/j.nic.2008.06.004.
- Ju K-Y, Lee JW, Im GH, et al. Bio-inspired, melanin-like nanoparticles as a highly efficient contrast agent for T1-weighted magnetic resonance imaging. Biomacromolecules. 2013;14(10):3491–3497. doi: 10.1021/bm4008138.
- Botta M, Geraldes CFGC, Tei L. High spin Fe(III)-doped nanostructures as T1 MR imaging probes. WIREs Nanomed Nanobiotechnol. 2023;15(2):1–19. doi: 10.1002/wnan.1858.
- Marasini R, Rayamajhi S, Moreno-Sanchez A, et al. Iron(III) chelated paramagnetic polymeric nanoparticle formulation as a next-generation T1-weighted MRI contrast agent. RSC Adv. 2021;11(51):32216–32226. doi: 10.1039/D1RA05544E.
- Wang Z, Zou Y, Li Y, et al. Metal‐containing polydopamine nanomaterials: catalysis, energy, and theranostics. Small. 2020;16(18):e1907042. doi: 10.1002/smll.201907042.
- Kaufman AA, Hansen RO, Kleinberg RLK. Chapter 6: paramagnetism, diamagnetism, and ferromagnetism. In: A.A. Kaufman, R.O. Hansen, R.L.K. Kleinberg (editors), Methods in geochemistry and geophysics; Elsevier, 2008. p. 207–254.
- Belorizky E, Fries PH. Characterising contrast agents for magnetic resonance imaging. In: P. Bertrand (editor), Electron paramagnetic resonance spectroscopy. Cham: Springer International Publishing; 2020. p. 313–349.
- Li M, Xuan Y, Zhang W, et al. Polydopamine-containing nano-systems for cancer multi-mode diagnoses and therapies: a review. Int J Biol Macromol. 2023;247(2023):125826. doi: 10.1016/j.ijbiomac.2023.125826.
- Liebscher J. Chemistry of polydopamine – scope, variation, and limitation. Eur J Org Chem. 2019;2019(31–32):4976–4994. doi: 10.1002/ejoc.201900445.
- Zandieh M, Liu J. Metal-doped polydopamine nanoparticles for highly robust and efficient DNA adsorption and sensing. Langmuir. 2021;37(30):8953–8960. doi: 10.1021/acs.langmuir.1c00783.
- Zandieh M, Liu J. Transition metal-mediated DNA adsorption on polydopamine nanoparticles. Langmuir. 2020;36(12):3260–3267. doi: 10.1021/acs.langmuir.0c00046.
- Lemaster JE, Wang Z, Hariri A, et al. Gadolinium doping enhances the photoacoustic signal of synthetic melanin nanoparticles: a dual modality contrast agent for stem cell imaging. Chem Mater. 2019;31(1):251–259. doi: 10.1021/acs.chemmater.8b04333.
- Bigaj-Józefowska MJ, Coy E, Załęski K, et al. Biomimetic theranostic nanoparticles for effective anticancer therapy and MRI imaging. J Photochem Photobiol B. 2023;249:112813. doi: 10.1016/j.jphotobiol.2023.112813.
- Qi C, Fu L-H, Xu H, et al. Melanin/polydopamine-based nanomaterials for biomedical applications. Sci China Chem. 2019;62(2):162–188. doi: 10.1007/s11426-018-9392-6.
- Wang Z, Xie Y, Li Y, et al. Tunable, metal-loaded polydopamine nanoparticles analyzed by magnetometry. Chem Mater. 2017;29(19):8195–8201. doi: 10.1021/acs.chemmater.7b02262.
- Li Y, Xie Y, Wang Z, et al. Structure and function of iron-loaded synthetic melanin. ACS Nano. 2016;10(11):10186–10194. doi: 10.1021/acsnano.6b05502.
- Wu Y, Huang Y, Tu C, et al. A mesoporous polydopamine nanoparticle enables highly efficient manganese encapsulation for enhanced MRI-guided photothermal therapy. Nanoscale. 2021;13(13):6439–6446. doi: 10.1039/D1NR00957E.
- Chen F, Xing Y, Wang Z, et al. Nanoscale polydopamine (PDA) meets π–π interactions: an interface-directed coassembly approach for mesoporous nanoparticles. Langmuir. 2016;32(46):12119–12128. doi: 10.1021/acs.langmuir.6b03294.
- Tran S, DeGiovanni P-J, Piel B, et al. Cancer nanomedicine: a review of recent success in drug delivery. Clin Transl Med. 2017;6(1):44. doi: 10.1186/s40169-017-0175-0.
- Xing Y, Zhang J, Chen F, et al. Mesoporous polydopamine nanoparticles with co-delivery function for overcoming multidrug resistance via synergistic chemo-photothermal therapy. Nanoscale. 2017;9(25):8781–8790. doi: 10.1039/C7NR01857F.
- Suneetha M, Kim H, Han SS. Doxorubicin-loaded fungal-carboxymethyl chitosan functionalized polydopamine nanoparticles for photothermal cancer therapy. Pharmaceutics. 2023;15(4):1281. doi: 10.3390/pharmaceutics15041281.
- Wu H, Zhang T, Liu Q, et al. Polydopamine-based loaded temozolomide nanoparticles conjugated by peptide-1 for glioblastoma chemotherapy and photothermal therapy. Front Pharmacol. 2023;14:1081612. doi: 10.3389/fphar.2023.1081612.
- Zhang L, Yang P, Guo R, et al. Multifunctional mesoporous polydopamine with hydrophobic paclitaxel for photoacoustic imaging-guided chemo-photothermal synergistic therapy. Int J Nanomedicine. 2019;14:8647–8663.
- Chen H, Chen H, Wang Y, et al. A novel self-coated polydopamine nanoparticle for synergistic photothermal-chemotherapy. Colloids Surf B Biointerfaces. 2021;200:111596. doi: 10.1016/j.colsurfb.2021.111596.
- Dai L, Wei D, Zhang J, et al. Aptamer‐conjugated mesoporous polydopamine for docetaxel targeted delivery and synergistic photothermal therapy of prostate cancer. Cell Prolif. 2021;54(11):e13130. doi: 10.1111/cpr.13130.
- Wang Z, Wang L, Prabhakar N, et al. CaP coated mesoporous polydopamine nanoparticles with responsive membrane permeation ability for combined photothermal and siRNA therapy. Acta Biomater. 2019;86:416–428. doi: 10.1016/j.actbio.2019.01.002.
- Wang L, He Y, He T, et al. Lymph node-targeted immune-activation mediated by imiquimod-loaded mesoporous polydopamine based-nanocarriers. Biomaterials. 2020;255:120208. doi: 10.1016/j.biomaterials.2020.120208.
- Mu X, Zhang F, Kong C, et al. EGFR-targeted delivery of DOX-loaded Fe3O4@polydopamine multifunctional nanocomposites for MRI and antitumor chemo-photothermal therapy. Int J Nanomedicine. 2017;12:2899–2911. doi: 10.2147/IJN.S131418.
- Liu J, Yu X, Braucht A, et al. N-cadherin targeted melanin nanoparticles reverse the endothelial–mesenchymal transition in vascular endothelial cells to potentially slow the progression of atherosclerosis and cancer. ACS Nano. 2024;18(11):8229–8247. doi: 10.1021/acsnano.3c12281.
- Liu J, Kang L, Smith S, et al. Transmembrane MUC18 targeted polydopamine nanoparticles and a mild photothermal effect synergistically disrupt actin cytoskeleton and migration of cancer cells. Nano Lett. 2021;21(22):9609–9618. doi: 10.1021/acs.nanolett.1c03377.
- Liu G, Zhou N, Cheng L, et al. “Four-in-one” versatile nanoplatforms for targeted dual chemo and photothermal synergistic cancer therapy. Pharmaceutics. 2019;11(10):507. doi: 10.3390/pharmaceutics11100507.
- Cao H, Jiang B, Yang Y, et al. Cell membrane covered polydopamine nanoparticles with two-photon absorption for precise photothermal therapy of cancer. J Colloid Interface Sci. 2021;604:596–603. doi: 10.1016/j.jcis.2021.07.004.
- Pellico J, Ellis CM, Davis JJ. Nanoparticle-based paramagnetic contrast agents for magnetic resonance imaging. Contrast Media Mol Imaging. 2019;2019:1845637. doi: 10.1155/2019/1845637.
- Rohrer M, Bauer H, Mintorovitch J, et al. Comparison of magnetic properties of MRI contrast media solutions at different magnetic field strengths. Invest Radiol. 2005;40(11):715–724. doi: 10.1097/01.rli.0000184756.66360.d3.
- Wang K, An L, Tian Q, et al. Gadolinium-labelled iron/iron oxide core/shell nanoparticles as T1–T2 contrast agent for magnetic resonance imaging. RSC Adv. 2018;8(47):26764–26770. doi: 10.1039/C8RA04530E.
- Ding X, Liu J, Li J, et al. Polydopamine coated manganese oxide nanoparticles with ultrahigh relaxivity as nanotheranostic agents for magnetic resonance imaging guided synergetic chemo-/photothermal therapy. Chem Sci. 2016;7(11):6695–6700. doi: 10.1039/c6sc01320a.
- Wang Z, Carniato F, Xie Y, et al. High relaxivity gadolinium-polydopamine nanoparticles. Small. 2017;13(43):1–7. doi: 10.1002/smll.201701830.