Abstract
Cell encapsulation into spherical microparticles is a promising bioengineering tool in many fields, including 3D cancer modelling and pre-clinical drug discovery. Cancer microencapsulation models can more accurately reflect the complex solid tumour microenvironment than 2D cell culture and therefore would improve drug discovery efforts. However, these microcapsules, typically in the range of 1 – 5000 µm in diameter, must be carefully designed and amenable to high-throughput production. This review therefore aims to outline important considerations in the design of cancer cell microencapsulation models for drug discovery applications and examine current techniques to produce these. Extrusion (dripping) droplet generation and emulsion-based techniques are highlighted and their suitability to high-throughput drug screening in terms of tumour physiology and ease of scale up is evaluated.
STATEMENT OF PURPOSE/HIGHLIGHT POINTS
3D microencapsulation models of cancer offer a customisable platform to mimic key aspects of solid tumour physiology in vitro. However, many 3D models do not recapitulate the hypoxic conditions and altered tissue stiffness established in many tumour types and stages. Furthermore, microparticles for cancer cell encapsulation are commonly produced using methods that are not necessarily suitable for scale up to high-throughput manufacturing. This review aims to evaluate current technologies for cancer cell-laden microparticle production with a focus on physiological relevance and scalability. Emerging techniques will then be touched on, for production of uniform microparticles suitable for high-throughput drug discovery applications.
Introduction
Cancer is a major public health burden and a leading cause of death globally [Citation1]. It is therefore imperative that anti-cancer drugs go through robust testing at the preclinical stage in order to produce effective treatments that decrease disease severity and prolong life expectancy. However, 97% of potential cancer therapies fail clinical trials [Citation2]. A major factor in this dismal success rate is that conventional pre-clinical cancer models do not accurately reflect the in vivo solid tumour microenvironment. They are oversimplified, such that drugs that appear to have pre-clinical efficacy are not effective when faced with the complexity of tumour biology in patients.
Current widely used pre-clinical models of cancer are monolayer adherent cell line culture and animal disease models. Culture of cancer cells in a monolayer on tissue culture plastic is a simple, high-throughput in vitro tumour model. However, cells grown in this way lack 3D architecture, interactions with the extracellular matrix (ECM), vasculature and stromal cells that influence tumour progression [Citation3,Citation4]. This affects key aspects of cellular behaviour [Citation5], as many studies have shown differences in morphology [Citation6], proliferation rate [Citation7], cell cycle distribution [Citation8], gene expression [Citation9] and drug sensitivity [Citation10] between cells cultured in 2D or 3D environments. Furthermore, cells grown in 2D are typically cultured on a substrate that is physiologically inaccurate in stiffness, meaning that cells are not grown in an appropriate mechanical environment which can dramatically alter cell gene expression and behaviour [Citation11]. Furthermore, in many cancer models only the cancer cell type of interest is grown, meaning that these models can only be used to test drugs that target the cancer cells themselves [Citation12], and do not take into consideration drugs that could target tumour-promoting stromal cells.
Animal models are currently considered the gold standard of pre-clinical drug testing, as they incorporate 3D architecture and stromal elements surrounding the tumour to mimic the native tumour microenvironment (TME). However, this strategy also has shortcomings since animal models are very often not predictive of clinical efficacy due to fundamental genetic, molecular and cellular differences between humans and commonly used animals such as mice [Citation13]. Furthermore, they are not amenable to high-throughput drug screening [Citation12] and present ethical concerns. For these reasons, it is necessary move towards a 3Rs approach and develop better, animal-free preclinical drug screening tools that more accurately reflect the in vivo TME.
An emerging approach to reflect the complex TME more accurately is to culture cancer cells in a 3D environment to recapitulate structural and functional properties of an in vivo tumour [Citation14]. 3D tumour cell cultures are often less sensitive to drugs than 2D cultures and therefore provide a more realistic drug response. There are a multitude of factors which contribute to this reduced drug sensitivity in 3D models. Drugs compounds in the medium have reduced delivery to cells within the centre of the 3D model, where hypoxic conditions also drive drug resistance. Cell-ECM interactions are also important in determining a tumour’s drug response [Citation15]. Therefore, 3D tumour models could avoid over-estimation of drug sensitivity in in vivo settings. In vitro 3D cell culture may be carried out in a scaffold-free environment such as the aggregation of cells to form multicellular tumour spheroids (MCTS) [Citation16] or by encapsulating cells within a scaffold material to mimic the ECM [Citation17]. Furthermore, these two approaches can be combined in microencapsulation cancer models which seek to aggregate cancer cells in spherical microparticles, microspheres or microgels made of scaffold material [Citation18], often a hydrogel. Cell microencapsulation is a useful bioengineering tool which packages cells into a semi-permeable membrane, often in order to provide a favourable environment for growth or to protect cells from harsh outside conditions. The technology was originally conceived to facilitate cell transplantation by protecting cells from the host’s immune response [Citation19], however is now useful in many fields such as tissue engineering and regenerative medicine [Citation20], expansion and cryopreservation of primary cells [Citation21,Citation22], delivery of biologic drugs [Citation23,Citation24] and food technology via encapsulation of probiotic cells [Citation25]. Advantages of microencapsulation models are providing a highly customisable microenvironment to incorporate biochemical, microenvironmental and mechanical factors that are specific to a particular cancer. These microencapsulation models specifically facilitate drug discovery by enabling cell-cell and cell-ECM interactions, physiological oxygen gradients and a 3D structure that limits drug availability to the innermost cells. The polymer microcapsule membrane may also serve to mimic the barrier of a blood vessel wall between the tumour cells and drug. For example, a previous study has constructed microencapsulation models using breast cancer cells encapsulated within alginate-poly-L-lysine- alginate (APA) microcapsules. Resulting multicellular spheroids within the capsules developed a necrotic core and had reduced sensitivity to chemotherapeutic drugs compared to monolayer cultures [Citation26].
Design considerations for microencapsulation models
One of the main points to consider when encapsulating cancer cells is the composition of the encapsulating matrix material. Biomaterials used for this purpose may be of natural origin, for example collagen and alginate, synthetic, such as polyethylene glycol (PEG) and polylactic acid (PLA) or a combination of the two in a hybrid material such as PEG-fibrinogen. This has been well reviewed elsewhere [Citation18] and will not be discussed in this work.
Several other design considerations are often overlooked when engineering a tumour-like model. It is important not only to provide a 3D scaffold for cell-cell interactions, but also an appropriate biochemical and biomechanical environment. A key feature of the TME is the establishment of biochemical and oxygen gradients across the tumour tissue which can have profound effects on cancer progression [Citation27]. Lack of oxygen in the centre of tumours (hypoxia) is known to promote many cellular processes for tumour progression and can contribute to drug resistance [Citation28]. Therefore, it is of great importance to consider microparticle size and its effect on hypoxia when designing microencapsulation models for drug screening. Microparticles with a diameter greater than the 100-200 µm diffusion distance of oxygen in tissues should therefore be used when modelling advanced disease. Controlling the microparticle size is also key for obtaining reproducible results in drug testing, as varying sizes could affect cellular response to drugs [Citation29].
Tumour cells also respond to the physical forces surrounding them and often have a much stiffer ECM than healthy tissue, leading to firm lumps in the tissue that can be detected by palpation. Altered stiffness is a driver of metastasis and drug resistance in many tumour types [Citation30]. Therefore, the stiffness of the malignant tissue should be reflected in the matrix material used to encapsulate the cells of interest.
In addition to the microenvironmental considerations above, thought must also be given to the scalability of the encapsulation technique. Small scale, physiologically complex in vitro models are useful to the fundamental researcher [Citation31], however their applicability to high-throughput drug screening is limited by a labour-intensive manufacturing method. Therefore, when designing a model for drug screening, the microencapsulation technique employed for model production must be scalable.
There are many techniques available for cell microencapsulation, such as interfacial phase inversion [Citation32], polyelectrolyte complexation [Citation33], and micromolding [Citation34] however these are generally used in other applications of cell encapsulation and do not fall into the scope of this review.
The remainder of this review will therefore focus on current microencapsulation techniques for the production of cancer drug screening models, taking into account the design considerations described above and how well they recapitulate the TME. The two main methods of microparticle production in cancer cell modelling will be discussed: extrusion and emulsion-based techniques, before reflecting on emerging techniques and their potential for cancer cell microencapsulation.
Extrusion methods
Extrusion or dripping through a nozzle is a popular method of producing hydrogel microparticles, due to its simple apparatus. In this technique, liquid precursor hydrogel is loaded into a pipette or syringe and slowly extruded through its nozzle such that the liquid precursor is released in a dropwise manner. This is often followed by external gelation of the droplets by collecting them into a bath containing crosslinker solution. Droplet size is mainly dependent on nozzle diameter and is also influenced by solution viscosity, flow rate and distance of the nozzle from the collection bath and is typically in the range of 1500−5000 µm [Citation35–37]. A low flow rate is needed to avoid polydispersity of the droplet size, so this method of particle production is typically low throughput [Citation38]. Droplet breakup can be promoted by input of energy in the form of electrostatic potential or vibration [Citation39,Citation40], or by altering the liquid flow by jet cutting [Citation41] or flowing onto a spinning disk [Citation42] (). This increases productivity and allows production of smaller particles below 1000 µm in diameter [Citation43].
Figure 1. (a) Methods of producing droplets by extrusion dripping. (b) Microfluidic flow focusing device for droplet generation. This figure was drawn by the authors.
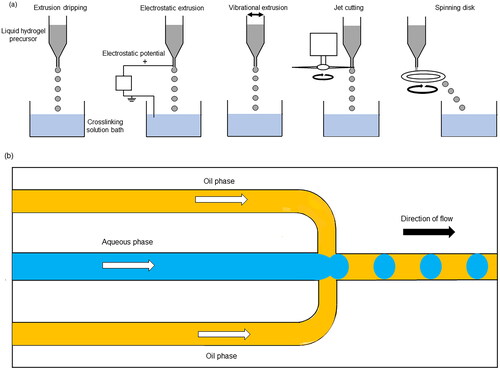
One approach to creating microparticles by extrusion is to use simple pipetting onto a surface to produce hydrogel droplets. The advantages of this method are a high degree of control in terms of particle size and complexity, and little to no specialist equipment required for production. For example, colorectal cancer microencapsulation models were created by pipetting a cell-laden alginate solution onto a hydrophobic surface block, followed by addition of crosslinker solution to form a very straightforward microencapsulation model [Citation44]. Yeon et al. [Citation45] used simple pipetting to create more complex multilayered core-shell beads comprised of collagen and alginate. The alginate solution was first pipetted through a milled multi-hole plastic substrate to form a hanging droplet, into which cell-laden collagen solution was then injected also by pipetting to form a double-layered droplet. The droplet can be customised by adding further hydrogel layers and allows culture of heterogeneous cell populations in different layers (). For example, breast cancer cells were encapsulated within the collagen core with mammary epithelial cells in the alginate layer to mimic the structure of the mammary duct and to investigate the differential cytotoxicity of drugs on cancerous and healthy cells. This precision drop-within-drop formation results in highly customisable and size-controllable particles.
Figure 2. Simple pipetting method to create multilayered collagen-alginate beads. (a) First layer of alginate formed by pipetting through a hole in the substrate. (b) Second layer of collagen and cells (red) added. (c) Third layer of collagen (green) to form a double-layered droplet. (d) Small amount of alginate added to the top of the droplet. (e) Resulting multilayered hydrogel beads. Figure taken from [Citation45] and reproduced with permission from Springer Nature.
![Figure 2. Simple pipetting method to create multilayered collagen-alginate beads. (a) First layer of alginate formed by pipetting through a hole in the substrate. (b) Second layer of collagen and cells (red) added. (c) Third layer of collagen (green) to form a double-layered droplet. (d) Small amount of alginate added to the top of the droplet. (e) Resulting multilayered hydrogel beads. Figure taken from [Citation45] and reproduced with permission from Springer Nature.](/cms/asset/f338f7c0-3953-4aff-bae3-713a1937c2e3/ianb_a_2359996_f0002_c.jpg)
In a similar vein, a co-culture model of prostate cancer and osteoblast cells has been fabricated using a digital repeater pipette to produce hyaluronan-gelatin based microparticles [Citation46]. Hydrogel droplets were extruded onto a superhydrophobic surface with great control over size by fine adjustments of the volume dispensed and were uniform and spherical in shape (). The microparticles were in the range of 0.8 − 2 mm diameter and therefore would allow physiologically relevant diffusion gradients to be established. The resulting bone metastasis niche model was shown to have higher resistance to a chemotherapeutic drug when compared to a conventional 3D spheroid model. However, due to the labour intensiveness during manufacturing, these simple pipetting techniques generally would not be suitable for high-throughput drug screening applications. Nevertheless, the methods outlined here may be useful when testing different matrix material and ECM mimetic component formulations in a medium-throughput setting, in particular those techniques that can be translated to a well plate format [Citation46]. Several studies have applied automated extrusion using a commercial microencapsulation system, or an in-house droplet generator using electrostatic potential to improve microparticle production throughput. Cells of different tumour types such as colorectal cancer [Citation47], breast cancer [Citation48] and hepatocellular carcinoma [Citation49] have been encapsulated in this way. In one study, a vibrational microencapsulation system produced hydrogel droplets at a relatively low flow rate (<1 L per hour) [Citation47]. Since the aim of the study was to recapitulate an early-stage tumour, a small droplet diameter was desirable to avoid induction of hypoxia associated with later-stage disease progression. In the vibrational system, flow rate decreases with droplet size, therefore this method is perhaps not suitable for high-throughput production of early-stage cancer models.
Figure 3. Production of microencapsulated prostate cancer bone metastasis niche model via pipetting onto a superhydrophobic surface. (a) Schematic production of microencapsulated co-culture bone metastasis niche model using methacrylated gelatin (GelMA) and methacrylated hyaluronan (HA-MA). (b) Widefield fluorescence micrographs of cell-specific tracking in microencapsulated models. Prostate cancer cells (PC-3) were stained with DiO (blue), and human osteoblasts (hOB) with DiD (red). Scale bars = 200 µm. Figure taken from [Citation46] and reproduced with permission from Elsevier.
![Figure 3. Production of microencapsulated prostate cancer bone metastasis niche model via pipetting onto a superhydrophobic surface. (a) Schematic production of microencapsulated co-culture bone metastasis niche model using methacrylated gelatin (GelMA) and methacrylated hyaluronan (HA-MA). (b) Widefield fluorescence micrographs of cell-specific tracking in microencapsulated models. Prostate cancer cells (PC-3) were stained with DiO (blue), and human osteoblasts (hOB) with DiD (red). Scale bars = 200 µm. Figure taken from [Citation46] and reproduced with permission from Elsevier.](/cms/asset/2b6f73e1-5c27-4a1d-b2a0-54b37997e666/ianb_a_2359996_f0003_c.jpg)
However, a more recent study [Citation48] was able to demonstrate production of uniform spheroids using a high-throughput vibrational microencapsulation device, although data concerning average particle size was not presented. The authors also showed the possibility to produce microparticles of a stiffness similar to primary breast tumour tissue [Citation11] by crosslinking. This is important to note due to the viscosity limitation of extrusion-based processes, which can cause blockages in the nozzle. Xu et al. [Citation49] used an electrostatic droplet generator to produce microparticles for the study of metastatic ability of encapsulated hepatocellular carcinoma cells. Spheroids were formed within five days of culture and had a high metastatic ability compared to 2D adhesion cultured cells. The authors concluded that the alginate bead models would be suitable for high-throughput screening of anti-metastatic drugs, however the bead size and size distribution was not reported other than stating that the size was uniform. Therefore, it is difficult to predict whether the beads produced using this technique would be suitable for scale up. Furthermore, alginate stiffness was not considered in the study but would have an important effect on drug response for this cancer type [Citation50].
Emulsion techniques
Emulsification is a common technique for microparticle production in many applications such as food [Citation51] and drug delivery [Citation52]. Cell encapsulation using emulsion techniques involves the dispersion of a cell-laden aqueous phase, usually a hydrogel, into an immiscible organic phase to create droplets of the former, and gelation is subsequently initiated by addition of a chemical crosslinker or alteration of temperature or pH, depending on the dispersed (aqueous) phase properties. Whilst coarse, conventional emulsification techniques such as homogenisation or rotor-stator emulsification are readily scalable, they may cause high shear and thermal stress, potentially damaging cells, and produce polydisperse droplets [Citation53]. Indeed, Pradhan et al. [Citation54] manufactured a PEG-fibrinogen based breast cancer microencapsulation model by vortexing the cell-laden aqueous phase with an oil phase to create an emulsion, followed by photocrosslinking for droplet gelation. The technique is simple and readily accessible requiring no specialist equipment, supported high cell viability and produced droplets that were less heterogeneous in size than spheroids produced by the hanging drop method. However, the microparticles were still very polydisperse with their diameters ranging from 50–500 µm. Therefore, a more controlled approach is necessary when producing emulsions for cell encapsulation and drug discovery applications.
Microfluidic emulsification (or droplet microfluidics) is an alternative to coarse emulsification and can produce highly monodisperse droplets by controlling fluid flow on a micrometer scale [Citation55]. This is commonly carried out on a chip device using a hydrodynamic flow focusing technique. This can be achieved by flowing a stream of aqueous phase through a channel in the device, which is broken up by flow of immiscible oil phase producing droplets in a one-by-one fashion (). Droplet size is highly controlled by microchannel geometry, and the main advantage of this technique is the capability to produce highly monodisperse droplets (Coefficient of Variation < 3%) [Citation56]. Typically, this technique handles very small volumes of liquid and is suitable for encapsulation of high value products in low quantities rather than large volume, lower value products [Citation57].
In biology, microfluidic techniques are particularly valuable for their ability to encapsulate single cells, DNA fragments and substrates of enzymatic reactions into discrete compartments [Citation55]. In recent years, this method has also been applied to the production of microencapsulated cancer models. Fridman et al. [Citation58] claimed high-throughput generation of alginate-alginate sulphate microparticles for breast cancer cell encapsulation using a microfluidic flow focusing device (). Particles produced were uniform in size and were used for co-culture of breast cancer and stromal cells in a physiologically relevant mechanical environment. Although the encapsulated cancer cells displayed hallmarks of tumour progression and immunomodulation, average microparticle diameter was only 144 µm therefore key nutrient and oxygen gradients would not have been established, although adjustments of device design have been successful in creating hypoxic conditions on chip [Citation59]. Furthermore, due to the design of the device, production throughput is limited to 1000 microparticles at a time and therefore considerable scale up would need to be implemented for use in drug discovery.
Figure 4. Microfluidic droplet generation for a breast cancer cell and fibroblast co-culture microencapsulation model. (a) Droplet generation process using alginate (Alg) or alginate-alginate sulphate (Alg/Alg-S). (b) Resulting droplets with encapsulated MCF7 cells labelled with CFSE (green) and CCD1129SK human mammary fibroblasts labelled with CMAC (blue). Upper panel: 5X magnification, after droplet formation; lower panel: 20X maginification, after hydrogel crosslinking. Scale bar in (a) and (b) upper panels = 200 µm. Scale bar in (b) lower panels = 50 µm. Figure taken from [Citation58] reproduced with permission from Elsevier.
![Figure 4. Microfluidic droplet generation for a breast cancer cell and fibroblast co-culture microencapsulation model. (a) Droplet generation process using alginate (Alg) or alginate-alginate sulphate (Alg/Alg-S). (b) Resulting droplets with encapsulated MCF7 cells labelled with CFSE (green) and CCD1129SK human mammary fibroblasts labelled with CMAC (blue). Upper panel: 5X magnification, after droplet formation; lower panel: 20X maginification, after hydrogel crosslinking. Scale bar in (a) and (b) upper panels = 200 µm. Scale bar in (b) lower panels = 50 µm. Figure taken from [Citation58] reproduced with permission from Elsevier.](/cms/asset/dab9e7aa-8965-4148-881b-023170808be9/ianb_a_2359996_f0004_c.jpg)
Other studies have also been successful in creating rapid and uniform cell-laden droplets and can offer precise control over droplet size, cell number per droplet [Citation60] and core-shell component ratio [Citation61] by adjusting fluid flow rate. However, droplet size range is again a limitation of these systems with maximum droplet diameter limited by the channel width. Nonetheless, Wu et al. [Citation60] did manufacture droplets with an average diameter of up to 350 µm which would allow establishment of physiologically relevant oxygen and nutrient gradients [Citation62] and demonstrated the potential of microfluidic techniques in personalised medicine for rapid screening of potential drugs against uniform patient-derived microencapsulated tumouroids.
A hybrid approach combining extrusion and microfluidic principles was employed by Sakai et al. [Citation63] to fabricate core-shell gelatine-alginate microparticles for cervical cancer cell encapsulation (). The authors extruded cell-laden gelatin droplets into a co-flowing oil stream to create a uniform emulsion. The gelatin microparticles were then solidified, collected and coated with alginate solution and extruded into a crosslinking solution via an electrostatic droplet generator to produce two-layered droplets This method appears to adopt the flexibility of extrusion methods for easy and cost-effective adjustment of droplet size, by varying nozzle width, whilst maintaining precise control over size uniformity using fluid flow. The resulting spheroids grown in microparticles were much more uniform in size and shape than those grown on agarose gel. As well as showing greater resistance to anti-cancer therapeutics than 2D cultured cells, hypoxia-inducible factor 1-alpha (HIF-1a) was also shown to be upregulated in the microencapsulation models, a major regulator of the hypoxic response [Citation64]. Furthermore, the study demonstrated that the cell-enclosing microparticles could be cryopreserved and thawed without affecting the cell growth and chemosensitivity. Therefore, this technology may be suitable for the production and storage of large numbers of cancer models for drug screening and generating an opportunity to create off-the-shelf, commercially available models that could be used by multiple labs and companies, improving consistency and comparability of results.
Figure 5. Core-shell gelatin-alginate microparticles for cervical cancer cell encapsulation produced using a hybrid extrusion and microfluidic approach. (a–d) HeLa cell growth and spheroid formation after encapsulation in two-layered microcapsules. (e) Spheroids harvested from microcapsules after 18 days of cultivation. (f) Spheroids formed on agarose gel after 9 days of cultivation. Scale bar in (a–d) = 150 μm; scale bar in (f) = 300 μm. Figure taken from [Citation63] reproduced with permission from John Wiley and Sons.
![Figure 5. Core-shell gelatin-alginate microparticles for cervical cancer cell encapsulation produced using a hybrid extrusion and microfluidic approach. (a–d) HeLa cell growth and spheroid formation after encapsulation in two-layered microcapsules. (e) Spheroids harvested from microcapsules after 18 days of cultivation. (f) Spheroids formed on agarose gel after 9 days of cultivation. Scale bar in (a–d) = 150 μm; scale bar in (f) = 300 μm. Figure taken from [Citation63] reproduced with permission from John Wiley and Sons.](/cms/asset/68d66798-0d1a-4406-bc48-6183aca24730/ianb_a_2359996_f0005_c.jpg)
In terms of on-demand mass production of particles by microfluidics, scale-up remains a significant challenge. Microfluidic devices are often costly and laborious to manufacture and due to limitations of droplet formation frequency, scale-out rather than scale-up is most widely applied to increase throughput [Citation65]. Running of multiple devices in parallel can cause challenges in ensuring consistent fluid flow, although this has been tackled by Nisisako et al. [Citation66] who were able to produce oil droplet emulsions on a large scale. However, high complexity of the system design and manufacturing costs are limiting. In-air microfluidics approaches similar to the strategy used by Sakai et al. [Citation63] may allow higher throughput, chip-free microparticle production in a biocompatible manner [Citation67].
Emerging techniques
Further to the main techniques of fabricating microparticles for cancer cell encapsulation reported so far in this review, there is also literature describing innovative ways of producing highly uniform droplets. Centrifugal droplet generation has been reported as a method to mass produce droplets encapsulating cancer cells in an oil-free and cost-effective manner [Citation68]. The technique uses centrifugal force to detach droplets from a nozzle and tune their size and rate of production. This work is promising for application in cancer drug screening due to its high uniformity of droplets, higher throughput capability than standard microfluidic devices and easy integration into cell biology laboratories. Another fully aqueous, highly uniform droplet production technique uses a microfluidic approach but employs acoustic modulation to form droplets in an aqueous two-phase system [Citation69]. The oil free formulation simplifies cancer cell-laden microparticle preparation and eliminates the need for washing and particle recovery steps. Acoustofluidics-produced cell-laden microparticles have previously been adapted to a well plate format [Citation70], therefore this technique may be amenable to high-throughput drug screening.
Another approach would be to consider a drop-by-drop emulsification technique that has higher throughput capabilities than microfluidics, such as membrane emulsification. Droplets are created by pushing an aqueous phase through a microporous membrane into an oil phase, creating a uniform emulsion. This technique is scalable [Citation71] and has previously been used for yeast cell encapsulation [Citation72] and therefore could be adaptable to mammalian cell encapsulation.
Concluding remarks
There is a great need for well-designed 3D cell culture systems to bridge the gap between 2D cell culture and animal models in in vitro cancer modelling and pre-clinical drug discovery. Cell microencapsulation models are an opportunity for increased physiological relevance in drug screening and therefore improvement in clinical trial success rates. High-throughput technologies for cell-laden microparticle production that allow control over particle size, uniformity and mechanical strength are therefore required. Extrusion based microparticle production is a relatively simple and versatile method to produce cancer microencapsulation models that recapitulate many aspects of tumour biology. However, data concerning microparticle size and uniformity are often lacking. Alternatively, microfluidic techniques have the advantage of producing highly uniform droplets but are less versatile in terms of increasing particle size. These current methods for cell microencapsulation models may struggle with translation to high-throughput manufacturing, however further innovation in these fields is bringing improvements to cell-laden microparticle production.
Authors’ contributions
L.B. and K.C. conceived and designed the review. L.B. drafted the article and K.C. revised it. Both authors agreed on the final version to be published.
Disclaimer
The views expressed in the submitted article are her own and not an official position of the institution or funder.
Disclosure statement
The authors report there are no competing interests to declare.
Data availability statement
Data sharing is not applicable to this article as no new data were created or analysed in this study.
Additional information
Funding
References
- Sung H, Ferlay J, Siegel RL, et al. Global cancer statistics 2020: GLOBOCAN estimates of incidence and mortality worldwide for 36 cancers in 185 countries. CA Cancer J Clin. 2021;71(3):209–249. doi: 10.3322/caac.21660.
- Wong CH, Siah KW, Lo AW. Estimation of clinical trial success rates and related parameters. Biostatistics. 2019;20(2):273–286. doi: 10.1093/biostatistics/kxx069.
- Bussard KM, Mutkus L, Stumpf K, et al. Tumor-associated stromal cells as key contributors to the tumor microenvironment. Breast Cancer Res. 2016;18(1):84. doi: 10.1186/s13058-016-0740-2.
- Henke E, Nandigama R, Ergün S. Extracellular matrix in the tumor microenvironment and its impact on cancer therapy. Front Mol Biosci. 2020;6:160. doi: 10.3389/fmolb.2019.00160.
- Edmondson R, Broglie JJ, Adcock AF, et al. Three-dimensional cell culture systems and their applications in drug discovery and cell-based biosensors. ASSAY Drug Dev Technol. 2014;12(4):207–218. doi: 10.1089/adt.2014.573.
- Huang H, Ding Y, Sun XS, et al. Peptide hydrogelation and cell encapsulation for 3D culture of MCF-7 breast cancer cells. PLoS One. 2013;8(3):e59482. doi: 10.1371/journal.pone.0059482.
- Luca AC, Mersch S, Deenen R, et al. Impact of the 3D microenvironment on phenotype, gene expression, and EGFR inhibition of colorectal cancer cell lines. PLoS One. 2013;8(3):e59689. doi: 10.1371/journal.pone.0059689.
- Lagies S, Schlimpert M, Neumann S, et al. Cells grown in three-dimensional spheroids mirror in vivo metabolic response of epithelial cells. Commun Biol. 2020;3(1):1–10. doi: 10.1038/s42003-020-0973-6.
- Szot CS, Buchanan CF, Freeman JW, et al. 3D in vitro bioengineered tumors based on collagen I hydrogels. Biomaterials. 2011;32(31):7905–7912. doi: 10.1016/j.biomaterials.2011.07.001.
- Loessner D, Stok KS, Lutolf MP, et al. Bioengineered 3D platform to explore cell-ECM interactions and drug resistance of epithelial ovarian cancer cells. Biomaterials. 2010;31(32):8494–8506. doi: 10.1016/j.biomaterials.2010.07.064.
- Butcher DT, Alliston T, Weaver VM. A tense situation: forcing tumour progression. Nat Rev Cancer. 2009;9(2):108–122. doi: 10.1038/nrc2544.
- Imparato G, Urciuolo F, Netti PA. In vitro three-dimensional models in cancer research: a review. Int Mater Rev. 2015;60(6):297–311. doi: 10.1179/1743280415Y.0000000003.
- Mak IWY, Evaniew N, Ghert M. Lost in translation: animal models and clinical trials in cancer treatment. Am J Translat Res. 2014;6(2):114–118.
- Smalley KSM, Lioni M, Herlyn M. Life isn’t flat: taking cancer biology to the next dimension. In Vitro Cell Dev Biol Anim. 2006;42(8-9):242–247. doi: 10.1290/0604027.1.
- Kapałczyńska M, Kolenda T, Przybyła W, et al. 2D and 3D cell cultures – a comparison of different types of cancer cell cultures. Arch Med Sci. 2018;14(4):910–919. doi: 10.5114/AOMS.2016.63743.
- Lin R-Z, Chang H-Y. Recent advances in three-dimensional multicellular spheroid culture for biomedical research. Biotechnol J. 2008;3(9-10):1172–1184. doi: 10.1002/biot.200700228.
- Thiele J, Ma Y, Bruekers SMC, et al. 25th anniversary article: designer hydrogels for cell cultures: a materials selection guide. Adv Mater. 2014;26(1):125–148. doi: 10.1002/adma.201302958.
- Ferreira LP, Gaspar VM, Mano JF. Design of spherically structured 3D in vitro tumor models -Advances and prospects. Acta Biomater. 2018;75:11–34. doi: 10.1016/J.ACTBIO.2018.05.034.
- Lim F, Sun AM. Microencapsulated islets as bioartificial endocrine pancreas. Science. 1979;210(4472):908–910. doi: 10.1126/SCIENCE.6776628.
- Du J, Yarema KJ. Cell microencapsulation for tissue engineering and regenerative medicine. In: J.M. Karp, W. Zhao, editors. Micro-and Nanoeng Cell Surface, vol 1. William Andrew Publishing, 2014. pp. 215–239. doi: 10.1016/B978-1-4557-3146-6.00010-6.
- Serra M, Correia C, Malpique R, et al. Microencapsulation technology: a powerful tool for integrating expansion and cryopreservation of human embryonic stem cells. PLoS One. 2011;6(8):e23212. doi: 10.1371/JOURNAL.PONE.0023212.
- Malpique R, et al. Alginate encapsulation as a novel strategy for the cryopreservation of neurospheres. Tissue Eng Part C Methods. 2010;16:965–977. doi: 10.1089/TEN.TEC.2009.0660.
- Murua A, Portero A, Orive G, et al. Cell microencapsulation technology: towards clinical application. J Controlled Release. 2008;132(2):76–83. doi: 10.1016/j.jconrel.2008.08.010.
- Dubrot J, et al. Delivery of immunostimulatory monoclonal antibodies by encapsulated hybridoma cells. Cancer Immunol Immunoth. 2010;59(11):1621–1631. doi: 10.1007/S00262-010-0888-Z.
- Heidebach T, Först P, Kulozik U. Microencapsulation of probiotic cells for food applications. Crit Rev Food Sci Nutr. 2012;52(4):291–311. doi: 10.1080/10408398.2010.499801.
- Zhang X, Wang W, Yu W, et al. Development of an in vitro multicellular tumor spheroid model using microencapsulation and its application in anticancer drug screening and testing. Biotechnol Progress. 2005;21(4):1289–1296. doi: 10.1021/bp050003l.
- Oudin MJ, Weaver VM. Physical and chemical gradients in the tumor microenvironment regulate tumor cell invasion, migration, and metastasis. Cold Spring Harb Symp Quant Biol. 2016;81(1):189–205. doi: 10.1101/sqb.2016.81.030817.
- Shannon AM, Bouchier-Hayes DJ, Condron CM, et al. Tumour hypoxia, chemotherapeutic resistance and hypoxia-related therapies. Cancer Treat Rev. 2003;29(4):297–307. doi: 10.1016/S0305-7372(03)00003-3.
- Asthana A, Kisaalita WS. Microtissue size and hypoxia in HTS with 3D cultures. Drug Discov Today. 2012;17(15-16):810–817. doi: 10.1016/j.drudis.2012.03.004.
- Ishihara S, Haga H. Matrix stiffness contributes to cancer progression by regulating transcription factors. Cancers (Basel). 2022;14(4):1049. doi: 10.3390/cancers14041049.
- Monteiro CF, Custódio CA, Mano JF. Bioengineering a humanized 3D tri-culture osteosarcoma model to assess tumor invasiveness and therapy response. Acta Biomater. 2021;134:204–214. doi: 10.1016/J.ACTBIO.2021.07.034.
- Crooks CA, Douglas JA, Broughton RL, et al. Microencapsulation of mammalian cells in a HEMA-MMA copolymer: effects on capsule morphology and permeability. J Biomed Mater Res. 1990;24(9):1241–1262. doi: 10.1002/JBM.820240908.
- Bhatia SR, Khattak SF, Roberts SC. Polyelectrolytes for cell encapsulation. Curr Opin Colloid Interface Sci. 2005;10(1-2):45–51. doi: 10.1016/j.cocis.2005.05.004.
- McGuigan AP, Bruzewicz DA, Glavan A, et al. Cell encapsulation in Sub-mm sized gel modules using replica molding. PLoS One. 2008;3(5):e2258. doi: 10.1371/JOURNAL.PONE.0002258.
- Chan ES, Lee BB, Ravindra P, et al. Prediction models for shape and size of ca-alginate macrobeads produced through extrusion–dripping method. J Colloid Interface Sci. 2009;338(1):63–72. doi: 10.1016/J.JCIS.2009.05.027.
- Davarcı F, Turan D, Ozcelik B, et al. The influence of solution viscosities and surface tension on calcium-alginate microbead formation using dripping technique. Food Hydrocoll. 2017;62:119–127. doi: 10.1016/j.foodhyd.2016.06.029.
- Costa ALR, Willerth SM, de la Torre LG, et al. Trends in hydrogel-based encapsulation technologies for advanced cell therapies applied to limb ischemia. Mater Today Bio. 2022;13:100221. doi: 10.1016/J.MTBIO.2022.100221.
- D, PonceletIn. Microencapsulation: fundamentals, methods and applications. In: V. M. Blitz, Jonathan P., Gun’ko, editors. Surface chemistry in biomedical and environmental science. Dordrecht, The Netherlands: Springer, 2006. pp. 23–34. doi: 10.1007/1-4020-4741-X.
- Manojlovic V, Djonlagic J, Obradovic B, et al. Immobilization of cells by electrostatic droplet generation: a model system for potential application in medicine. Int J Nanomedicine. 2006;1(2):163–171. p. doi: 10.2147/NANO.2006.1.2.163.
- Heinzen C, Berger A, Marison I. Use of vibration technology for jet Break-Up for encapsulation of cells and liquids in monodisperse microcapsules. In: C. Heinzen, A. Berger, and I. Marison, editors. Fundamentals of cell immobilisation biotechnology, vol 8a. Dordrecht, The Netherlands: Springer, 2004. pp. 257–275. doi: 10.1007/978-94-017-1638-3_14.
- Preibisch I, Niemeyer P, Yusufoglu Y, et al. Polysaccharide-Based aerogel bead production via jet cutting method. Materials 2018. 2018;11(8):1287. doi: 10.3390/ma11081287.
- Teunou E, Poncelet D. Rotary disc atomisation for microencapsulation applications-prediction of the particle trajectories. J Food Eng. 2005;71:345–353. doi: 10.1016/j.jfoodeng.2004.10.048.
- Leong J-Y, Lam W-H, Ho K-W, et al. Advances in fabricating spherical alginate hydrogels with controlled particle designs by ionotropic gelation as encapsulation systems. Particuology. 2016;24:44–60. doi: 10.1016/j.partic.2015.09.004.
- Smit T, Calitz C, Willers C, et al. Characterization of an alginate encapsulated LS180 spheroid model for anti-colorectal cancer compound screening. ACS Med Chem Lett. 2020;11(5):1014–1021. doi: 10.1021/ACSMEDCHEMLETT.0C00076/SUPPL_FILE/ML0C00076_SI_001.PDF.
- Yeon JH, Chung SH, Baek C, et al. A simple pipetting-based method for encapsulating live cells into multi-layered hydrogel droplets. BioChip J. 2018;12(3):184–192. doi: 10.1007/s13206-018-2307-z.
- Antunes J, Gaspar VM, Ferreira L, et al. In-air production of 3D co-culture tumor spheroid hydrogels for expedited drug screening. Acta Biomater. 2019;94:392–409. doi: 10.1016/j.actbio.2019.06.012.
- Rios De La Rosa JM, Wubetu J, Tirelli N, et al. Colorectal tumor 3D in vitro models: advantages of biofabrication for the recapitulation of early stages of tumour development. Biomed Phys Eng Express. 2018;4(4):045010. doi: 10.1088/2057-1976/aac1c9.
- Ertekin Ö, Monavari M, Krüger R, et al. 3D hydrogel-based microcapsules as an in vitro model to study tumorigenicity, cell migration and drug resistance. Acta Biomater. 2022;142:208–220. doi: 10.1016/J.ACTBIO.2022.02.010.
- Xu X-X, Liu C, Liu Y, et al. Encapsulated human hepatocellular carcinoma cells by alginate gel beads as an in vitro metastasis model. Exp Cell Res. 2013;319(14):2135–2144. doi: 10.1016/j.yexcr.2013.05.013.
- Schrader J, Gordon-Walker TT, Aucott RL, et al. Matrix stiffness modulates proliferation, chemotherapeutic response, and dormancy in hepatocellular carcinoma cells. Hepatology. 2011;53(4):1192–1205. doi: 10.1002/HEP.24108.
- Choudhury N, Meghwal M, Das K. Microencapsulation: an overview on concepts, methods, properties and applications in foods. Food Front. 2021;2(4):426–442. doi: 10.1002/FFT2.94.
- Singh MN, Hemant KSY, Ram M, et al. Microencapsulation: a promising technique for controlled drug delivery. Res Pharm Sci. 2010;5(2):65.
- Spyropoulosa F, Hancocks RD, Norton IT. Food-grade emulsions prepared by membrane emulsification techniques. Procedia Food Sci. 2011;1:920–926. doi: 10.1016/j.profoo.2011.09.139.
- Pradhan S, Clary JM, Seliktar D, et al. A three-dimensional spheroidal cancer model based on PEG-fibrinogen hydrogel microspheres. Biomaterials. 2017;115:141–154. doi: 10.1016/j.biomaterials.2016.10.052.
- van Vliet LD, Hollfelder F. Microfluidic droplets and their applications: diagnosis, drug screening and the discovery of therapeutic enzymes. IFMBE Proc. 2020;69:361–368. doi: 10.1007/978-981-13-5859-3_63/FIGURES/5.
- Vladisavljević GT, Kobayashi I, Nakajima M. Production of uniform droplets using membrane, microchannel and microfluidic emulsification devices. Microfluid Nanofluid. 2012;13(1):151–178. doi: 10.1007/s10404-012-0948-0.
- Shewan HM, Stokes JR. Review of techniques to manufacture micro-hydrogel particles for the food industry and their applications. J Food Eng. 2013;119(4):781–792. doi: 10.1016/j.jfoodeng.2013.06.046.
- Fridman IB, et al. High-throughput microfluidic 3D biomimetic model enabling quantitative description of the human breast tumor microenvironment. Acta Biomater. 2021;132:473–488. doi: 10.1016/J.A2021.06.025CTBIO.
- Berger Fridman I, Ugolini GS, Vandelinder V, et al. High throughput microfluidic system with multiple oxygen levels for the study of hypoxia in tumor spheroids. Biofabrication. 2021;13(3):035037. doi: 10.1088/1758-5090/ABDB88.
- Wu Z, Gong Z, Ao Z, et al. Rapid microfluidic formation of uniform Patient-Derived breast tumor spheroids. ACS Appl Bio Mater. 2020;3(9):6273–6283. doi: 10.1021/acsabm.0c00768.
- Li Y, Hai M, Zhao Y, et al. Controlled generation of cell-laden hydrogel microspheres with core-shell scaffold mimicking microenvironment of tumor. Chinese Phys B. 2018;27(12):128703. doi: 10.1088/1674-1056/27/12/128703.
- Kingsley DM, Roberge CL, Rudkouskaya A, et al. Laser-based 3D bioprinting for spatial and size control of tumor spheroids and embryoid bodies. Acta Biomater. 2019;95:357–370. doi: 10.1016/j.actbio.2019.02.014.
- Sakai S, Inamoto K, Liu Y, et al. Multicellular tumor spheroid formation in duplex microcapsules for analysis of chemosensitivity. Cancer Sci. 2012;103(3):549–554. doi: 10.1111/j.1349-7006.2011.02187.x.
- Jun JC, Rathore A, Younas H, et al. Hypoxia-Inducible factors and cancer. Curr Sleep Med Rep. 2017;3(1):1–10. doi: 10.1007/S40675-017-0062-7.
- Duran M, Serrano A, Nikulin A, et al. Microcapsule production by droplet microfluidics: a review from the material science approach. Mater Des. 2022;223:111230. doi: 10.1016/j.matdes.2022.111230.
- Nisisako T, Ando T, Hatsuzawa T. High-volume production of single and compound emulsions in a microfluidic parallelization arrangement coupled with coaxial annular world-to-chip interfaces. Lab Chip. 2012;12(18):3426–3435. doi: 10.1039/C2LC40245A/.
- Visser CW, Kamperman T, Karbaat LP, et al. In-air microfluidics enables rapid fabrication of emulsions, suspensions, and 3D modular (bio)materials. Sci Adv. 2018;4(1):eaao1175. doi: 10.1126/SCIADV.AAO1175/SUPPL_FILE/AAO1175_SM.PDF.
- De Lora JA, Velasquez JL, Carroll NJ, et al. Centrifugal generation of droplet-based 3D cell cultures. SLAS Technol. 2020;25(5):436–445. doi: 10.1177/2472630320915837/ATTACHMENT/578A34E8-F542-4826-9A89-971D95EBA568/MMC1-SUPPL.PDF.
- De Lora JA, Fencl FA, Macias Gonzalez ADY, et al. Oil-Free acoustofluidic droplet generation for multicellular tumor spheroid culture. ACS Appl Bio Mater. 2019;2(9):4097–4105. doi: 10.1021/ACSABM.9B00617/ASSET/IMAGES/LARGE/MT9B00617_0005.JPEG.
- Thakuri PS, Ham SL, Luker GD, et al. Multiparametric analysis of oncology drug screening with aqueous two-phase tumor spheroids. Mol Pharmaceutics. 2016;13(11):3724–3735. doi: 10.1021/ACS.MOLPHARMACEUT.6B00527/ASSET/IMAGES/LARGE/MP-2016-00527S_0008.JPEG.
- Piacentini E, Dragosavac M, Giorno L. Pharmaceutical particles design by membrane emulsification: preparation methods and applications in drug delivery. Curr Pharm Des. 2018;23(2):302–318. doi: 10.2174/1381612823666161117160940.
- Morelli S, Holdich RG, Dragosavac MM. Microparticles for cell encapsulation and colonic delivery produced by membrane emulsification. J Memb Sci. 2017;524:377–388. doi: 10.1016/j.memsci.2016.11.058.