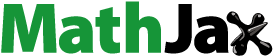
ABSTRACT
This paper reports on analyses of the compressive Young’s modulus (Ep), compressive strength (σp) and thermal conductivity (κ) of sintered porous yttria-stabilized zirconia compacts (YSZ). The Ep and σp values are affected by the number of grains and the grain boundary area in sintered YSZ ceramics. The measured porosity dependence of the Ep and σp values was compared with that of the theoretical Ep and σp values derived for the open pore structure. The tendency of Ep and σp values to increase at low porosity was clarified by the proposed theoretical porosity dependence of Ep and σp. The strain at fractures also increased with increases in the grain boundary area and depended on the crack propagation mode affected by the degree of grain growth. The κ values for porous YSZ, which decreased at high porosity, were analyzed theoretically with two model structures: a pore-dispersed YSZ continuous phase system (F model) and a YSZ-dispersed pore continuous phase system (G model). The measured κ values at 0–30% porosity were in good agreement with the κ values calculated for the model F structure. In the high porosity range above 50%, the measured κ values approached the κ value curve for the model G structure with increases in porosity.
1. Introduction
Porous ceramics are widely used as refractory bricks, filters, catalyst supports and electrodes in solid oxide fuel cells. The mechanical and thermal properties of porous ceramics exert a significant influence on the above applications. Ryshkewitch [Citation1] reported the empirical linear relationship between the logarithmic compressive strength and the porosity of porous ceramics. Many researchers have devoted efforts to examining the experimental parameters fitted in the empirical equation. Our group analyzed the mechanical properties of porous ceramics theoretically and experimentally with respect to the grain boundary area between partially sintered grains [Citation2–Citation4]. The strength (σp) of porous alumina is dominated by the number of grains (N) packed in bulk volume (V) and the grain boundary area (πy2, y: radius of circular grain boundary). Equation (1) has been derived for σp of the partially sintered spherical particles illustrated in ,
where p (=h/r), n and D represent the ratio of the distance shrunk (h) between two particles to the particle radius (r), the coordination number of the particles and the relative density (ratio of bulk density to theoretical density), respectively. The σ0 value corresponds to the strength needed to fracture the several grain boundaries surrounding one grain three-dimensionally at D = 1. As seen in Equation (1), increases in the p and D values enhance the compressive strength of porous ceramics. The D value in Equation (1) is also a function of the p value. The p value is derived from the specific surface area of porous ceramics [Citation2,Citation3]. In the initial stage of sintering, the relative densities of alumina compacts are almost constant, but the specific surface areas decrease with increases in the sintering temperature [Citation2]. Under these conditions, increases in the p value contribute to the strength of porous ceramics. In the middle stage of sintering, the relative density increases from 60% to 80% with the sintering temperature. Both the p and the D values enhance the σp value, as shown in Equation (1). Similarly, the Young’s modulus (E) for a porous structure with continuous (open) pores was derived theoretically based on Equation (1) in the previous paper [Citation5]. The derived relationship between the Young’s modulus and porosity is also analyzed in the present paper.
The thermal conductivity (κ) of porous ceramics is derived theoretically using three parameters: κ1 for a gas phase contained in the pores, κ2 for continuous solid phase 2 and the volume fraction V1 of the pores [Citation6]. The measured thermal conductivity of porous mullite with 0–23% porosity was in good agreement with the values calculated for the pore-dispersed structure [Citation7]. In the high-porosity range from 33% to 55%, the measured thermal conductivity deviated from the values for the pore-dispersed structure and approached the values for the pore-continuous structure [Citation7]. The results indicate that the pore-dispersed structure at low porosity changes to a mixed structure of pore-dispersed mullite-continuous phase and mullite-dispersed pore-continuous phase at high porosity. Similarly, the Maxwell model [Citation8] treats homogeneous structure of the two phases, and clarifies the thermal conductivity of porous yttria-stabilized zirconia ceramics (YSZ) fabricated with polymer spheres as a pore former [Citation9]. The Effective Medium Theory presents the thermal conductivity of heterogeneous structures of two continuous phases and clarifies the thermal conductivity (0.06–0.42 W/mK) of YSZ ceramics with a high porosity of 52–76% [Citation10]. The Intermingled Fractal Units model [Citation11] also clearly presents the thermal conductivity of porous YSZ with unidirectionally aligned pore channels fabricated by a freeze-casting process [Citation12].
As explained above, the development of the pore structure during sintering is affected by the sintering temperature, the packing density of the powder compact and the particle size and shape of the starting powder. In this paper, a porous ceramic compact was fabricated with a granular powder of nanometer-sized primary YSZ particles [Citation13]. Granular powder is widely used in industrial applications to increase the compactibility of fine particles. The compressive strength, compressive Young’s modulus and thermal conductivity of partially sintered porous YSZ compacts were investigated with respect to the formation of grain boundaries. The measured properties were analyzed using the proposed theoretical equations. In this paper, theoretical expressions of the compressive strength, including the number of fractured grain boundaries per sintered grain, Young’s modulus and strain at fracture based on the theoretical compressive strength, were newly derived and compared with the measured results. The theoretical values were in good agreement with the measured values for porous microstructures of nanometer-sized YSZ grains.
2. Experimental procedure
2.1. Fabrication of porous YSZ compacts
An 8 mol% yttria-stabilized zirconia powder (YSZ, Y0.15Zr0.85O1.93) with a Brunauer–Emmett–Teller (BET) surface area of 11.58 m2/g (corresponding particle size 87.8 nm) and a true density of 5.826 g/cm3 (Tosoh Co., Ltd., Tokyo, Japan) was dispersed in double-distilled water to make 10 vol% solid suspensions at pH 3.0 using a 1 M-HCl solution. After the 10 vol% suspensions were stirred for 24 h, they were consolidated by pressure filtration up to 16 MPa through a glass filter with a 20 μm pore diameter and three sheets of a membrane filter with a 0.1 μm pore diameter, which were attached to the bottom of a piston moving at a crosshead speed of 0.2 mm/min [Citation13–Citation15]. When a YSZ suspension in a closed cylinder (20 mm diameter) was compressed by the piston, the filtrate moved into and through pore channels formed in the upper piston. The consolidated powder compacts were dried at 373 K in air for 24 h. The dried powder compacts were then sintered at 973–1773 K in air for 1–4 h. The apparent and bulk densities of the sintered YSZ were measured by the Archimedes method using double-distilled water. The BET specific surface areas of the sintered YSZ compacts were determined by the nitrogen gas adsorption method at P(equilibrium N2 pressure)/P0(saturated N2 pressure) = 0.30 using a mixed gas of 30% N2–70% He (i.e. the single-point BET method, Flow Sorb II 2300, Shimazu Co., Kyoto, Japan). Prior to the specific surface area measurement, the samples were dried at 373 K for 24 h. The microstructures of the sintered YSZ were observed by field-emission scanning electron microscope (S-4100H, Hitachi High-Technologies Co., Tokyo, Japan) and analyzed to measure their grain sizes using an image analysis software (LUZUX-F, Nireco Co., Tokyo, Japan). The average grain size was determined from ~75 grains.
2.2. Measurements of compressive mechanical properties
Each sintered porous YSZ compact was cut into a rectangular prism with a length of 5 mm, a width of 5 mm and a height of 5 mm. The porous YSZ prisms were then sandwiched between two copper plates (20 × 20 × 1 mm3), as shown in . The samples were then compressed at a crosshead speed of 0.5 mm/min while the strain along the compressive direction was measured using a strain gauge attached to the samples. Details of the configuration employed for the compressive test have been reported elsewhere [Citation2,Citation16]. The compressive test was performed with five samples for each sintering condition. In the compressive test, the samples were fractured to measure their compressive strengths.
2.3. Measurements of heat capacity and thermal diffusivity
The heat capacity (CP1, J/m3K at 0.1013 MPa, 1 atm) and thermal diffusivity of the porous YSZ compacts (diameter 10 mm, thickness 2 mm) were measured at room temperature by the laser flash method at Okayama Ceramics Research Foundation (1406–18 Nishikatakami, Bizen-shi, Okayama, Japan). A pulsed laser was irradiated on a sample surface, and the time-dependence of temperature on the opposing surface of the sample was measured. Based on the time-dependence of temperature, the thermal diffusivity (α, m2/s) and specific heat capacity (CP2, J/gK) were determined. The thermal diffusivity is expressed by Equation (2):
where L and t1/2 are the thickness of the sample and half the time until the surface of the sample reaches the maximum temperature. The CP2 value is expressed by Equation (3) at room temperature and Equation (4) at higher temperatures, respectively:
where m, ma and mb are the masses of the sample, a laser absorber and an adhesive agent, respectively, Q the absorbed heat quantity, and ΔT0,R and ΔT0,T the parameters of the temperature relaxation curves at room temperature and higher temperatures, respectively. The thermal conductivity (κ, W/mK) is obtained by multiplying the thermal diffusivity (α, m2/s), specific heat capacity (CP2, J/gK) and bulk density (ρ, g/m3), as presented by Equation (5):
3. Results and discussion
3.1. Sintering of porous YSZ compacts
shows the (a) specific surface area, (b) linear shrinkage and (c) relative density of porous YSZ sintered at 973–1673 K. The specific surface areas of the porous YSZ compacts decreased at above 1273 K, and the linear shrinkage and relative density increased with the heating temperature. No difference in the shrinkage was observed in the directions of the diameter or height of the YSZ compacts, a result reflecting isotropic shrinkage with sintering. shows the relative density as a function of linear shrinkage (q) during sintering based on the starting diameter or height of the YSZ sample. The four solid lines represent the theoretical relationship between the relative density (D) and the linear shrinkage (q) as expressed by Equation (6):
Figure 3. (a) Specific surface area, (b) shrinkage and (c) relative density of porous YSZ compacts as functions of sintering temperature.
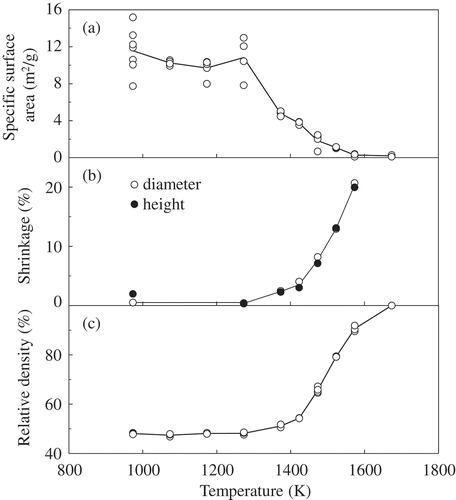
Figure 4. Relationship between shrinkage (q) and relative density for YSZ compacts sintered at 973–1573 K. The four solid lines represent the theoretical D–q relationship expressed by Equation (6) in the text.

The packing density (D0) of spherical particles in a green compact is 0.524, 0.637, 0.680 and 0.741 for a simple cubic structure (sc), a random close packed structure (rcp), a body-centered cubic structure (bcc) and a cubic close packed structure (ccp), respectively. The measured green densities (47.7–48.4%) near q = 0% were close to the D0 value for sc structure. The measured q values for both the diameter and the height of the disk sample changed along the sc curve of the D–q relationship. The packing densities of the alumina powder compacts of submicrometer-sized particles in our previous paper were 60.8–61.9% [Citation3] and higher than the YSZ green densities in . Granulation of the nanometer-sized YSZ particles reduced the packing density.
shows typical microstructures for YSZ sintered at (a) 973 K, (b) 1373 K, (c) 1473 K and (d) 1523 K. The microscopic images of the microstructures at 973 and 1373 K show uniform packing of the nanometer-sized YSZ particles. With increases in the sintering temperature (1473, 1523 K), the YSZ particles were connected with each other. As seen in , the grain growth rate increased at higher sintering temperatures. shows the grain size distributions of the porous YSZ compacts sintered at 973–1573 K. In the sintering temperature range from 973 to 1473 K, narrow grain size distributions with median sizes of 80–105 nm were observed. The measured median sizes were close to the diameter (88 nm) of the starting YSZ particles corresponding to the specific surface area. When the sintering temperature increased to above 1523 K, the grain size distributions became significantly wider, raising the median size to 171 and 206 nm after sintering at 1523 and 1573 K, respectively.
3.2. Compressive mechanical properties of porous YSZ compacts
shows typical compressive stress (σ)–strain (ε) relationships for porous and dense YSZ compacts (48.1–100% relative densities) sintered at 1273–1773 K. The σ–ε plot of each sample exhibited good linearity. A similar good linear relationship was also observed in sintered porous alumina compacts of submicrometer-sized grains [Citation3]. A nonlinear stress–strain curve (i.e. pseudo-ductile property) was observed in sintered porous alumina compacts, however, which were fabricated from submicrometer-sized bimodal particles [Citation4]. This type of deformation behavior was analyzed in our previous paper.
Figure 7. Compressive stress–strain curves for YSZ compacts sintered at 1273–1773 K in air. The sudden decrease in compressive strain indicated by the arrow reflects a compressive fracture.
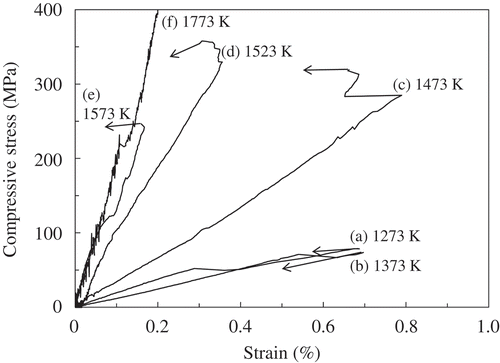
shows the dependence of (a) compressive strength (σp), (b) Young’s modulus (Ep) and (c) strain (εp) at fracture of YSZ sintered at 973–1773 K on the f (p) value (=3.046(2p − p2)D2/3/(4 − 3np2 + np3)2/3) of the right term of Equation (1). The f (p) value is explained in Section 1. The p value is related to the linear shrinkage q value in Equation (7) based on the starting diameter or height of the YSZ disk [Citation2,Citation3]:
Figure 8. (a) Compressive strength, (b) Young’s modulus and (c) strain at fracture for porous YSZ ceramics as functions of the f (p) value in the right term of Equation (1) in the text. The solid circle (●) and open circle (○) keys represent the YSZ samples of small grain growth and large grain growth during sintering, respectively. (See the grain size distributions in .) The A–E lines show the theoretical relationship presented in .
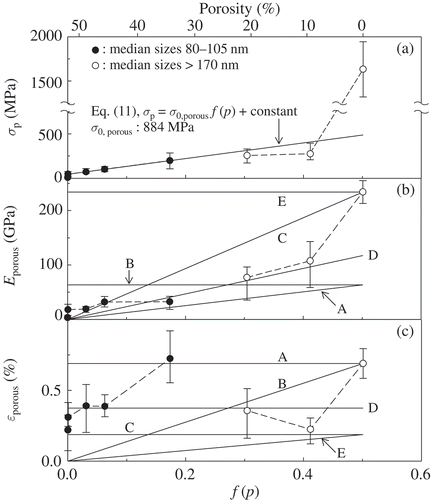
The A–E lines in show the theoretical relationship presented in and are explained in Section 3.2.2. The compressive strength increased with increases in f (p). The strength extrapolated from the data for the porous YSZ compacts to f (p) = 0.5012 for full density was lower, however, than the measured strength (average value 1.63 ± 0.31 GPa). This result is discussed in a later section. The measured compressive strength (1.63 GPa) for dense YSZ compacts (100% relative density) sintered at 1773 K for 4 h was slightly lower than the reported compressive strength (2.1 GPa) for 12 mol% Y2O3-stabilized ZrO2 single crystal [Citation17], and was 4.4 times greater than the bending strength of 370 MPa [Citation18] or 7.3 times greater than the tensile strength of 225 MPa for 8 mol% YSZ polycrystalline [Citation18]. Similarly, the Young’s modulus of the porous YSZ increased gradually with increases in the f (p) value. The strain at fracture showed a tendency to increase with increases in the f (p) value for two different microstructures (small grain growth for those of 80–105 nm median size and large grain growth for those of >170 nm median size). The extrapolated strain at fracture became larger for small grain growth as compared with large grain growth at a similar f (p) value. The measured compressive strengths, Young’s moduli and strains at fracture are compared with the calculated values in the later sections based on the theoretical relationships derived in previous works [Citation2–Citation5].
Table 1. Possible Young’s modulus (Ep) and strain (εp) at fracture for porous YSZ ceramics.
3.2.1. Compressive strength
The compressive strength of porous ceramics (σp) is expressed by Equation (8) (modified Equation (1)) in relation to the compressive strength (σ2) of fully dense ceramics, the f (p) value defined by Equation (1) and the number (nf) of grain boundaries which originally surrounded the individual grains and were fractured by compressive stress:
In Equation (8), the nf value at fractures is distinguished from the coordination number (n) in the f (p) value related to the sintering process. That is, the σ0,porous value in Equation (8) is expressed by Equation (9):
For fully dense ceramics, Equation (10) is derived from Equation (8).
where F corresponds to the f (p) value at D(relative density) = 1. That is, nf,dense is equal to 2/F. In this experiment, nf,dense is calculated to be 2/F = 3.99 for F = 0.5012 and the ratio of nf,dence to n (coordination number, 6) results in 4/6 (=0.67). The difference in the compressive strengths of porous and dense ceramics is therefore dominated by the difference in the nf and f (p) values in Equation (8). shows the fractured surface of a fully dense YSZ compact with a compressive strength of 1.32 GPa. Two types of crack propagation, from the grain boundaries and grain interiors, were observed. It is apparent from the observation in that the nf,dense value is lower than the n value (coordination number of grains).
Figure 9. Microstructure of the fractured surface after a compressive test of a fully dense YSZ compact with compressive strength of 1.32 GPa.
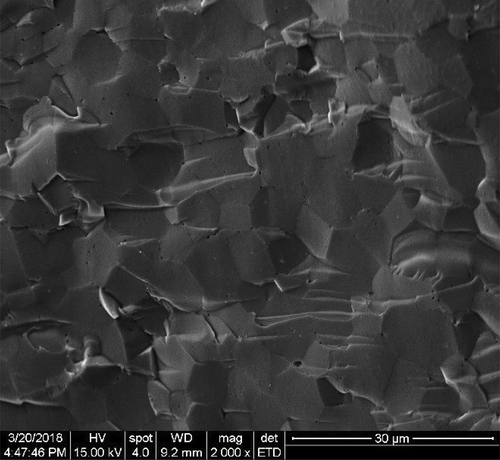
As seen in ), the σp–f (p) relationship showed good linearity for small grain growth in the f (p) range from 0 to 0.174, which was expressed by Equation (11):
The σp value at f (p) ~0 corresponds to the compressive strength of a weak green compact. The measured slope provided the values of σ0,porous = 884 MPa and nf,porous = 1.08 from Equation (9) for σ2 = 1633 MPa. This result indicates that (1) the nf,porous value is about one-fourth of the nf,dense value (=3.99) and (2) compressive fractures of porous YSZ compacts are caused by the fracture of one grain boundary per grain. The drastic increase of σp at f (p) > 0.4 is due to the change from nf,porous to nf,dense with decreasing porosity and additional fractures from the grain interiors with large grain growth.
In dense ceramics or glass, mechanical strength is dominated by the largest defect located on the surface or in the interior. The nature of surface defects in non-oxide ceramics is different from those of oxide ceramics. When SiC or Si3N4 is heated in an oxygen-rich atmosphere, the SiC or Si3N4 is converted to SiO2, which is accompanied by a volume expansion and decrease in the size of the surface defects, leading to an increase in mechanical strength (i.e. healing effect) [Citation19–Citation21]. Similarly, surface defects of glass are healed through the viscous flow of atoms during heating, which inhibits any decrease in strength. Thus, the disappearance of surface defects with annealing in an oxygen-rich atmosphere contributes to strengthening these materials. On the other hand, surface defects in highly porous ceramics are difficult to locate with certainty and to distinguish from the interior defects. Although the influence of surface defects can be examined by healing the defects, no such experiment was carried out in this study. Also, since the load applied to porous ceramics is transmitted to many grain boundaries, it is hard to derive a clear quantitative relationship between the surface defect size and stress distribution. For the above reasons, the influence of surface defects on compressive strength was not examined in this study. In Ref. [Citation22], it is reported that the surface defects of porous ceramics provide only a negligible influence on their mechanical strengths.
3.2.2. Compressive Young’s modulus and strain at fracture
Equations (8) and (10) are combined to give Equation (12), which clarifies the relationship between the Young’s modulus (Ep) and strain (εp) at fracture for porous YSZ:
where E2 and ε2 represent the Young’s modulus and strain at fracture for dense YSZ at 0% porosity. Possible Ep and εp values are summarized in according to various conditions. (b,c) shows the possible Ep and εp calculated for E2 = 234 GPa, F = 0.5012, ε2 = 0.689%, nf,dense = 3.99 and nf,porous = 1.08. The Young’s modulus (E2) for dense YSZ (100% relative density) sintered at 1773 K for 4 h was measured at 234 GPa, which was 14–17% larger than the reported values (205.8 GPa measured for 8 mol% Y2O3-stabilized zirconia with 2% porosity by the impulse excitation technique [Citation23] and 200 GPa measured for fully dense 8 mol% Y2O3-stabilized zirconia by the tensile test [Citation18]). The measured Ep values at 10–50% porosity, plotted along the D line in , approached the E2 value for dense YSZ with decreases in porosity. Little influence of grain growth on the Ep value (D line) was observed in ). A similar change of Ep with f (p) was measured for partially sintered porous alumina compacts in our previous paper [Citation5]. The εp for the D condition in was calculated to be 0.370%, which was similar to the measured εp values and lower than the ε2 value (0.684%), as seen in ). The measured εp values were distributed in the range of 0.2–0.6%. The upper and lower limits of εp are clarified by the A and C conditions. The above observation suggests that (1) the Ep values for the present porous YSZ were approximated by the product E2 f (p) (D condition), (2) the εp value for the D condition did not agree with the measured tendency of εp with f (p) and (3) the measured εp values were distributed between the upper and lower limits for the A and C conditions.
To understand the tendency of εp with f (p) in ) more clearly, the εp values were plotted against the Ep values in . The plotted data for two different microstructures of (a) small grain growth and (b) large grain growth indicate the tendency of the εp value to increase with increases in the Ep value. That is, an increase in the grain boundary area (increase in the f (p) value in ) contributes to increases in both the Ep and the εp values. The effect of grain growth on the εp value is closely related to the crack propagation mode during compressive tests [Citation22]. In the microstructures of small grain growth, fractures occur mainly along weak grain boundaries. Cracks in the microstructures of large grain growth, on the other hand, are propagated in the grain boundaries and grain interiors (). The data in ) and suggest that it is possible to achieve a high εp value in the microstructures of small grain growth. In addition, the corresponding compressive strength in ) also increases with increases in the grain boundary area.
Figure 10. Relationship between the Young’s modulus and strain at fracture for porous YSZ compacts with (a) median sizes of 80–105 nm (relative density, D = 0.48–0.65) and (b) large median sizes above 170 nm (relative density, D = 0.79–1.00) after sintering at 973–1473 and 1523–1773 K, respectively.
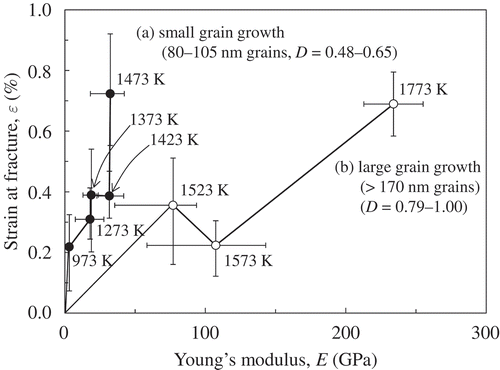
3.3. Thermal diffusivity, heat capacity and thermal conductivity of porous YSZ compacts
shows the thermal diffusivity (α), specific heat capacity (CP1) and thermal conductivity (κ = CP1α) of porous YSZ compacts at room temperature as a function of porosity (closed porosity plus open porosity). The α value was independent of porosity up to 20% porosity and then decreased with increases in porosity. The CP1 value decreased linearly with increasing porosity. The κ value (product of α and CP1) decreased nonlinearly with porosity. The measured κ values were compared with the calculated κap value for a solid material with particulate inclusion by Equation (13) [Citation6]:
Figure 11. (a) Thermal diffusivity (α), (b) specific heat capacity (CP1) at a constant pressure (1 atm, 101.33 kPa) and (c) thermal conductivity (κ = CP1α) of sintered porous YSZ compacts as functions of porosity.
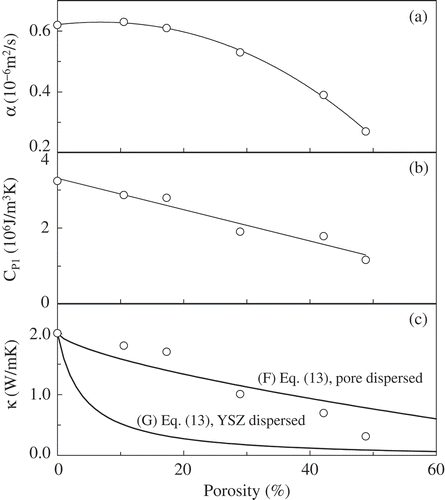
where κ1 and κ2 are the thermal conductivity for the dispersed and continuous phases, respectively, and V1 is the volume fraction of the dispersed phase. The κap value was calculated for two model structures: model F – a pore-dispersed YSZ continuous phase system and model G – a YSZ-dispersed pore continuous phase system. The following κ values were used for the calculation: κ(air, 298 K) = 0.0265 W/mK [Citation24], κ(YSZ, 298 K) = 2.01 W/mK (measured for the dense YSZ at 0% porosity). ) compares the κ values measured for porous YSZ compacts with the κ values calculated by Equation (13). The κ values calculated for the pore-dispersed YSZ continuous phase system (model F structure) decrease moderately with increasing porosity. In the model G structure of the YSZ-dispersed pore continuous phase system, incorporation of a small number of pores drastically decreases the κ values of porous YSZ. The measured κ values decreased along the κ curve for the model F structure by 30% porosity. In our previous study of the thermal conductivity of an alumina–mullite system [Citation25] and porous polycrystalline mullite [Citation7], the measured κ values at 0–25% porosity were well matched with the calculated κ curve for the model F structure (pore-dispersed phase 1–solid continuous phase 2). The good agreement between the measured κ value and the calculated κ value in the previous and present analyses indicates that (1) the proposed Equation (13) for the pore-dispersed phase is effective for microstructures containing at least 30 vol% porosity and (2) the kind of pore structure (open pores for a real microstructure or closed pores for the model F structure) has no influence on the κ values at 0–30% porosity. The measured κ values at 42–49% porosity deviated from the κ curve calculated for the model F structure, however, and approached the κ curve calculated for the model G structure. The real microstructure includes the characteristics of both model structures F and G.
4. Conclusions
Porous yttria-stabilized zirconia compacts (YSZ) were fabricated from a granular powder of nanometer-sized particles (median particle size 88 nm) to investigate the relationship between porosity and mechanical and thermal properties. Theoretical expressions of the compressive strength, including the number of fractured grain boundaries per sintered grain, Young’s modulus and strain at fracture based on the theoretical compressive strength, were newly derived in this study.
The YSZ aqueous suspensions were consolidated by pressure filtration. The green YSZ powder disks with a packing density of about 50% were sintered isotropically at 973–1773 K.
The compressive strength (σp) of a porous structure with open pores was theoretically expressed as functions of the shrinkage (p) between sintered YSZ grains, relative density (D), coordination number (n) of particles related to the sintering process and number (nf) of fractured grain boundaries that originally surrounded the individual grains and that were fractured by compressive stress.
The measured σp value matched well with the proposed theory of compressed strength. The relatively low σp values for sintered porous YSZ compacts were characterized by a small nf value (1.08) and a small grain boundary area as compared with the large nf value (3.99) and large grain boundary area of dense YSZ compacts.
The measured Young’s modulus (Ep) of porous YSZ compacts was also clearly expressed as functions of the Young’s modulus (E2) of dense YSZ, p, n and D. An increase in the grain boundary area with sintering contributes to increases in both the Ep value and the strain (εp) at fracture.
The εp value depended on the crack propagation mode affected by the degree of grain growth.
The thermal conductivities (products of thermal diffusivity and heat capacity) of the sintered porous YSZ compacts decreased gradually with increasing porosity. The κ values for the porous YSZ were calculated theoretically for the following two model structures: a pore-dispersed YSZ continuous phase system (F model structure) and a YSZ-dispersed pore continuous phase system (G model structure). The measured κ values at 0–30 vol% porosity were appropriately delineated by the calculated κ curve for the model F structure. The measured κ values at 42–49% porosity deviated from the κ curve calculated for the model F structure and approached the κ value curve calculated for the model G structure.
Disclosure statement
No potential conflict of interest was reported by the authors.
Additional information
Funding
References
- Ryshkewitch E. Compression strength of porous sintered alumina and zirconia. J Am Ceram Soc. 1953;36:65–68.
- Hirata Y, Shimonosono T, Sameshima T, et al. Compressive mechanical properties of porous alumina compacts. Ceram Int. 2014;40:2315–2322.
- Hirata Y, Shimonosono T, Sameshima S, et al. Sintering of alumina powder compacts and their compressive mechanical properties. Ceram Int. 2015;41:11449–11455.
- Hirata Y, Fujita H, Shimonosono T. Compressive mechanical properties of partially sintered porous alumina of bimodal particle size system. Ceram Int. 2017;43:1895–1903.
- Hirata Y, Takehara K, Shimonosono T. Analyses of Young’s modulus and thermal expansion coefficient of sintered porous alumina compact. Ceram Int. 2017;43:12321–12327.
- Hirata Y. Representation of thermal conductivity of solid material with particulate inclusion. Ceram Int. 2009;35:2921–2926.
- Hirata Y, Kinoshita Y, Shimonosono T, et al. Theoretical and experimental analyses of thermal properties of porous polycrystalline mullite. Ceram Int. 2017;43:9973–9978.
- Maxwell JC. A treatise on electricity and magnetism. Oxford, UK: Clarendon Press; 1904.
- Schlichting KW, Padture NP, Klemens PG. Thermal conductivity of dense and porous yttria-stabilized zirconia. J Mater Sci. 2001;36:3003–3010.
- Hu L, Wang C, Huang Y. Porous yttria-stabilized zirconia ceramics with ultra-low thermal conductivity. J Mater Sci. 2010;45:3242–3246.
- Pia G, Sanna U. An intermingled fractal units model to evaluate pore size distribution influence on thermal conductivity values in porous materials. Appl Therm Eng. 2014;65:330–336.
- Pia G. High porous yttria-stabilized zirconia with aligned pore channels: morphology directionality influence on heat transfer. Ceram Int. 2016;42:11674–11681.
- Ueno T, Hirata Y, Shimonosono T. Analysis of compressive deformation behavior of wet powder compacts of nanometer-sized yttria-stabilized zirconia particles. Ceram Int. 2016;42:1926–1932.
- Hirata Y, Matsushima K, Matsunaga N, et al. Viscoelastic properties of flocculated alumina suspensions during pressure filtration. J Ceram Soc Jpn. 2010;118:977–982.
- Hirata Y, Fukunaga Y, Matsunaga N, et al. Influence of pressure on filtration of aqueous alumina suspensions. Ceram Int. 2013;39:3547–3554.
- Shimonosono T, Ikeyama S, Hirata Y, et al. Compressive deformation of liquid phase-sintered porous silicon carbide ceramics. J Asian Ceram Soc. 2014;2:422–428.
- Lankford J. Deformation and fracture of yttria-stabilized zirconia single crystals. J Mater Sci. 1986;21:1981–1989.
- Kondoh J, Shiota H, Kawachi K, et al. Yttria concentration dependence of tensile strength in yttria-stabilized zirconia. J Alloy Compd. 2004;365:253–258.
- Matsunaga N, Hidaka N, Sameshima S, et al. Mechanical properties of SiC processed with nanometer-sized powder and polytitanocarbosilane. Key Eng Mater. 2007;352:49–52.
- Matsunaga N, Nakahama K, Hirata Y, et al. Enhancement of strength of SiC by heat-treatment in air. J Ceram Proc Res. 2009;10:319–324.
- Hirata Y, Matsunaga N, Hidaka N, et al. Improvement of Strength, Weibull modulus and damage tolerance of SiC. Mater Sci Forum. 2007;561–565:489–494.
- Deng Z-Y, Fukasawa T, Ando M, et al. Microstructure and mechanical properties of porous alumina ceramics fabricated by the decomposition of alumina hydroxide. J Am Ceram Soc. 2001;84:2638–2644.
- Giraud S, Canel J. Young’s modulus of some SOFCs materials as a function of temperature. J Eur Ceram Soc. 2008;28:77–83.
- Hata K, editor. Chemical handbook, basic part II. 3rd ed. Tokyo, Japan: Maruzen; 1984.
- Itoh S, Hirata Y, Shimonosono T, et al. Theoretical and experimental analyses of thermal conductivity of the alumina–mullite system. J Eur Ceram Soc. 2015;35:605–612.