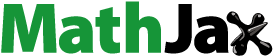
ABSTRACT
Preparing drug-eluting beads (DEBs) from radiopaque materials such as titania (TiO2) can meet clinical need for directly visualizing DEBs during drug-eluting bead transcatheter arterial chemoembolization (DEB-TACE). Porous anatase-type TiO2 microspheres with mean volume diameters of approximately 30 µm were obtained when silica nanoparticles (SiNPs) were introduced into the TiO2 matrix by a sol–gel process involving a water-in-oil emulsion, and the SiNPs were then dissolved by subsequent treatment with NaOH solution. Of special note, microspheres prepared using SiNPs of approximately 20 – 25 nm in diameter had a high specific surface area of ~120 m2·g−1 and a high doxorubicin (DOX)-adsorption capacity of ~150 mg·mL−1, and they gradually released ~10% of the adsorbed DOX within 5 days. The DOX-loaded microspheres were non-cytotoxic, moreover, and exerted anticancer effects on HeLa cells. We propose that the present TiO2 microspheres are potentially useful as novel radiopaque embolic materials for DEB-TACE.
1. Introduction
Hepatocellular carcinoma (HCC) is the most common primary malignancy of the liver. HCC is also the sixth most common neoplasm and the third most frequent cause of cancer-related deaths [Citation1]. The four major treatments used for combating HCC are (1) hepatic resection, (2) transcatheter arterial chemoembolization (TACE), (3) percutaneous therapies such as percutaneous ethanol-injection therapy, percutaneous microwave coagulation therapy, and radiofrequency ablation, and (4) chemotherapy. TACE is a specific type of chemoembolization that blocks the hepatic artery to treat liver cancer [Citation2]. In Japan, TACE is widely performed in 32% of patients with unresectable HCC after the initial diagnosis and in 58% of those with recurrent HCC [Citation3]. Gelatin sponge particles and polyvinyl alcohol microspheres have been used as embolic materials in conventional TACE [Citation4,Citation5]. Prior to embolization of the hepatic artery using embolic materials in conventional TACE, a mixed solution of an imaging agent (such as lipiodol) and an anticancer drug (such as doxorubicin or cisplatin) is injected into the arterial supply closest to the tumor.
As an alternative to conventional TACE, TACE using drug-eluting beads (DEB-TACE) has attracted attention [Citation2] for its ability to deliver higher concentrations of anticancer drugs to within the target tumor and to lower the systemic concentration compared with conventional TACE. Typical DEBs comprise sulfonate-modified poly (vinyl alcohol) hydrogel or a copolymer of sodium acrylate and vinyl alcohol. Presently, DC Bead®, HepaSphereTM, and Embozene TANDEMTM are approved as embolic materials for DEB-TACE [Citation6–Citation8]. The benefits for survival and tumor response of DEB-TACE over conventional TACE are not yet well defined, although a randomized controlled trial revealed an improved tumor response rate for DEB-TACE compared with conventional TACE [Citation9].
DEBs are radiolucent, since they are polymeric materials, as described above, which makes it impossible to determine their spatial-temporal distribution within target tissues. Hence, a clinical need exists for direct visualization of DEBs to assess the completeness of target tissue embolization (for efficacy) as well as unintentional embolization of non-target tissue (for safety) [Citation10]. Ceramic microspheres with low radiolucency such as titania (TiO2) are candidate radiopaque embolic materials. We reported previously that porous TiO2 microspheres can be prepared by a sol–gel technique [Citation11] and that microspheres can release rhodamine B (a model hydrophilic drug) [Citation12], but the size of the microspheres (~15 µm) was not appropriate for arterial embolization. In this study, we attempted to synthesize porous TiO2 microspheres several dozen micrometers in diameter by modifying the preparation conditions and evaluated the potential of porous TiO2 microspheres as radiopaque DEBs by investigating their doxorubicin (DOX)-loading and releasing ability as well as their cytotoxicity and anticancer efficacy with respect to HeLa tumor cells. Although HeLa cells are not HCC cells but, rather, cervical cancer cells, it is possible to evaluate the anticancer efficacy of TiO2 microsphere samples using HeLa cells in this preliminary study.
2. Materials and methods
2.1. Preparation of samples
All reagent-grade chemicals were purchased from Wako Pure Chemical Industries, Ltd. (Tokyo, Japan). Porous TiO2 microspheres were prepared using a sol–gel method with a water-in-oil (W/O) emulsion, based on our previous studies [Citation13,Citation14]. The composition and stirring conditions of the oil phase were the same as those in our previous study [Citation14]. The components and mixing conditions of the aqueous phase were also the same as those in our previous study [Citation14], but the methanol content was reduced from 2.7 g to 1.7 g for this study.
Similarly to our previous study [Citation12], silica (SiO2) nanoparticle (SiNP) suspensions with varying SiO2 contents were prepared by adding different amounts of colloidal SiO2 with SiNPs of different sizes (20 − 25 nm and 40 − 50 nm) (SNOWTEX® XL and SNOWTEX® 50, Nissan Chemical Industries, Ltd., Tokyo, Japan) to ultrapure H2O. The concentrations of the SNOWTEX® XL and SNOWTEX® 50 SiNPs were 40 and 48 wt. %, respectively. The total H2O content of the dispersion was fixed at 4.2 g by adjusting the amount of H2O added. The SiNP dispersion was mixed with the aqueous phase and then dropped into the oil phase under continuous mixing in a homogenizer to achieve a W/O emulsion. shows the sample names, SiNP sizes, and SiO2/H2O weight ratios in the aqueous phase. The capital letters (STXL or ST50) in the sample names indicate the abbreviated SiNP product names (STXL: SNOWTEX® XL, ST50: SNOWTEX® 50) and the numbers in the sample names (STXL-xx or ST50-xx) indicate the SiO2 content (wt. %). As a reference, Non-porous TiO2 microspheres (np-Ti) were prepared as references without adding an SiNP dispersion. Mixing proceeded at 1500 rpm at temperatures of 30°C for 20 min, 40°C for 20 min, and, finally, 55°C for 140 min. After mixing, the W/O emulsions were centrifuged at 3000 rpm for 5 min (CN-1050, AS ONE Corp.), and the supernatant was removed by decantation. The precipitates were washed thrice with 30 mL of ethanol, dried at 36.5°C for 24 h, heat-treated at 150°C for 3 h with the temperature increasing at a rate of 0.25°C·min−1, and, finally, heat-treated at 600°C for 5 h with the temperature increasing at a rate of 2.5°C·min−1. The heating steps were performed in an electric furnace (FO100, Yamato Scientific Co., Ltd., Tokyo, Japan).
Table 1. Sample names, the SiNP sizes, and the SiO2/H2O weight ratios in the starting solutions.
To create porous structures, the heat-treated samples were soaked in centrifuge tubes containing 6 mL of 3 mol·L−1 sodium hydroxide (NaOH) at room temperature for 24 h (NaOH treatment). After soaking, the solutions were centrifuged at 6000 rpm (CN-1050, AS ONE Corp.), and the supernatant was removed by decantation.
Thirty milliliters of pure water were added to the centrifuge tubes, and the samples were washed gently by shaking. This washing process was repeated three times. Finally, the samples were dried at 90◦C for 24 h.
2.2. Characterization of samples
Sample morphologies were observed by field-emission scanning electron microscopy (FE-SEM; JEM-7100F, JEOL Ltd., Tokyo, Japan). The crystalline phases of the samples were investigated using a powder X-ray diffractometer (XRD: MiniFlex600HDA, Rigaku Corp., Tokyo, Japan) with a Cu Kα source operating at 40 kV and 15 mA at a scanning rate of 4◦·min−1. The structures of the samples were investigated by Fourier transform infrared spectroscopy (FT-IR; FT/IR-6200, JASCO Corp., Tokyo, Japan) by recording the transmission spectra using the potassium bromide (KBr) pellet method. The KBr contents of the tested samples were maintained at approximately 0.125 wt. %. The specific surface area (SSA), total pore volume, and pore size distribution of the samples were measured using a gas absorption analyzer (Autosorb®-iQ, Quantachrome Corp., Florida, USA). The samples were heated at 200◦C for 2 h before measurements were taken to remove adsorbed water. The particle size distributions of the samples were measured with a particle size analyzer (NANO-flex, MicrotracBEL Corp., Osaka, Japan), and the zeta potentials of the samples in pure water were measured using a streaming potential analyzer (Stabino, MicrotracBEL Corp., Osaka, Japan).
2.3. Evaluation of the samples’ DOX loading and release
Two-milligram samples of STXL-10, STXL-20, ST50-10, and ST50-20 were soaked in microtubes containing 1.5 mL of 0.5 mg·mL−1 aqueous DOX solution. The microtubes were rotated at 3 rpm for 0.5 to 4 h at room temperature with a tube rotator (TR-350, AS ONE Corp.). After the desired period, the samples were removed from the DOX solution by centrifugation at 3000 rpm for 3 min (CN-1050, AS ONE Corp.). Then the supernatant was diluted ten-fold, and the DOX concentration in each diluted supernatant was measured using an ultraviolet–visible range spectrometer (V-730BIO, JASCO Corp.). The amount of DOX loaded into the samples was calculated from the DOX concentration measured in the supernatant.
The samples were washed with 1.5 mL of phosphate buffer solution (PBS) at the end of the 4 h experimental period, and the resulting DOX-loaded samples (prepared with STXL-10, STXL-20, ST50-10, and ST50-20) were soaked in 1.5 mL of PBS in microtubes that were then rotated at 36.5◦C with a tube rotator at 3 rpm for 15 min to 120 h. After the desired period, each sample was extracted by centrifugation, and the amount of DOX in the supernatant was measured by spectrophotometry. These DOX-loading and -release evaluations were performed for three replicas per sample.
2.4. Evaluation of the in vitro biocompatibility of the samples
The in vitro biocompatibility of the samples was evaluated using Rat fibroblast Rat-1 cells in a procedure similar to that in our previous studies [Citation14,Citation15]. Rat-1 cells were cultured in Dulbecco’s modified Eagle’s medium (DMEM; Wako Pure Chemical Industries Ltd.) with 10% horse serum (Thermo Fisher Scientific, K.K., Tokyo, Japan), 100 units·mL−1 penicillin (Meiji Seika Kaisha, Ltd., Tokyo, Japan), and 100 µg·mL−1 streptomycin (Meiji Seika Kaisha, Ltd.).
Cellular experiments were conducted for the following four groups: a control group (no administration), a pure TiO2 microsphere group (np-Ti), and two porous TiO2 microsphere groups (STXL-20 and ST50-20). A cell suspension consisting of 1 × 104 Rat-1 fibroblasts was seeded in each well of a 24-well plate with 0.8 mL medium. Using 24-well cell culture inserts with porous membrane bottoms with 1.0-µm pores (Corning Inc.), test samples (np-Ti, STXL-20, and ST50-20) were added to the wells by deposition on the membranes of the inserts. The sample groups (np-Ti, STXL-20, and ST50-20) were tested at concentrations of 1, 10, and 100 mg/well. Cell proliferation was examined after 1, 3, and 7 days of culture. Total DNA from the cells was extracted using an AllPrep DNA/RNA/Protein Mini Kit (Qiagen GmbH, Hilden, Germany) in accordance with the manufacturer’s protocol [Citation16]. Following extraction, the DNA concentrations were measured by absorbance at 260 nm using a spectrophotometer (e-spect, BM Equipment Co., Ltd., Tokyo, Japan). The values derived from five replicates per sample were presented as the mean DNA content. The results were expressed as the mean ± the standard deviation. Statistical analysis was performed using Tukey’s test, and differences with p-values < 0.05 were considered statistically significant.
2.5. Evaluation of the anticancer efficacy of samples with respect to HeLa cells
STXL-20 samples loaded with different amounts of DOX were evaluated for anticancer efficacy because STXL-20 showed the highest DOX-loading ability among the present samples. First, the samples were autoclaved at 121◦C for 20 min. Then, 1.0 mg of each autoclaved sample was soaked in a microtube containing 0.8 mL of aqueous DOX solution at a concentrations of 0, 0.01, 0.1, 10, or 100 µg·mL−1. The microtubes were rotated at 3 rpm for 4 h at room temperature. The DOX-loaded samples were then collected from the DOX solutions by centrifugation at 104 rpm for 1 min (CF15RXII, Hitachi Koki Co., Ltd., Tokyo, Japan). They were soaked in 1.0 mL of pure water in microtubes, gently washed by shaking the microtubes and then collected by centrifugation at 104 rpm for 1 min.
HeLa cells were cultured in DMEM (Wako Pure Chemical Industries Ltd.) containing 10% fetal bovine serum (Thermo Fisher Scientific, K.K.), 100 units·mL−1 penicillin (Meiji Seika Kaisha, Ltd.), and 100 µg·mL−1 streptomycin (Meiji Seika Kaisha, Ltd.). The cells were routinely subcultured every third day in a 60.1 cm2 culture dish at 37◦C in humidified air with 5% CO2.
A cell suspension consisting of 2 × 104 HeLa cells was seeded in each well of a 24-well plate with 0.8 mL of medium. Into 24-well cell culture inserts with porous membrane bottoms with 1.0-µm pores (Corning Inc.), 1.0 mg of each DOX-loaded STXL-20 sample (prepared using DOX solutions with DOX concentrations of 0, 0.01, 0.1, 10, and 100 µg·mL−1, as described above) was added separately by deposition on the membranes of the inserts (DOX-MS group). As a reference, DOX was added directly to the culture medium to reach DOX concentrations of 0, 0.01, 0.1, 1, 10, and 100 µg·mL−1 (DOX-SOL group). Cell proliferation was examined after 1, 2, and 3 days of culture. Subsequently, HeLa cell viabilities were evaluated using an MTT Cell Proliferation Assay Kit (Trevigen, Inc., Gaithersburg, MD, USA) in accordance with the manufacturer’s protocol. Following extraction, the absorbance at 560 nm was measured using a microplate reader (GloMax®-Multi Detection System, Promega, K.K., Tokyo, Japan). In this study, the absorbance obtained for the DOX-SOL group with a DOX concentration of 0 µg·mL−1 (no administration) was used as a control (Acontrol), and the viability of the HeLa cells was calculated using the following equation, based on the absorbance obtained for each sample (Asample):
The values were derived from five replicates per sample and presented as the mean viabilities of HeLa cells. The results are expressed as the mean ± the standard deviation. Statistical analysis was performed using Tukey’s test, and differences from p-values < 0.05 were considered statistically significant.
3. Results
3.1. Structure and properties of samples
shows FE-SEM photographs of the samples. Irregularly shaped particles and particles with surface cracks were partially formed, but microspheres measuring several tens of micrometers in diameter were obtained. Samples STXL-10 and STXL-20 had pores of ~20 nm, and samples ST50-10 and ST50-20 had pores of ~40 nm. The number of pores on the surface of the samples increased with increases in the concentration of SiNP in the starting solution.
shows the particle size distributions of the samples. The sizes of all the samples ranged roughly from 10 to 90 µm, and the mean diameters of np-Ti, STXL-10, STXL-20, ST50-10, and ST50-20 were 27.9, 33.3, 32.6, 25.1, 28.7 µm, respectively.
shows the (a) XRD patterns and (b) FT-IR spectra of the samples. As in our previous study [Citation12], strong XRD diffraction peaks ascribed to anatase (Powder Diffraction File, Card No. 21–1272) were observed for all the samples. In addition to the anatase peaks, small XRD diffraction peaks ascribed to rutile (PDF: 21–1276) were found in the spectrum of sample np-Ti and IR absorption bands were observed at about 1220, 1100, 800, and 460 cm−1 in samples ST50-10 and ST50-20. These absorption bands were ascribable to Si–O–Si [Citation17]. The IR absorption bands at about 1100 cm−1 were more obvious in samples ST50-10 and ST50-20 than in samples STXL-10 and STXL-20. A broad IR absorption band in the rang of 400–800 cm−1, which has been ascribed to Ti–O [Citation18,Citation19], and a small IR absorption band at about 940 cm−1, which has been ascribed to Si–O–Ti [Citation18,Citation20], were observed in samples STXL-10 and STXL-20.
shows the SSAs and total pore volumes of the samples. Sample np-Ti had a significantly lower SSA and total pore volume than the other samples, and samples STXL-20 and ST50-20 had higher SSAs and total pore volumes than samples STXL-10 and ST50-10, respectively. Samples STXL-10 and STXL-20 also showed higher SSAs than samples ST50-10 and ST50-20, respectively, but there was little difference betwen the total pore volumes of sample STXL-10 and sample ST50-10 or of sample STXL-20 and sample ST50-20.
Table 2. SSA, total pore volume, and zeta potential for samples.
25E6
3.2. DOX-adsorption capacity and DOX-release profiles of the samples
shows the DOX-adsorption capacity of the samples. Sample STXL-20 showed the highest DOX-adsorption capacity of 0.14 mg·mg−1 after soaking in DOX solution for 4 h. shows the DOX-release profiles of the samples. The present samples released 2‒8% of the adsorbed DOX within 5 days. Drug-delivery devices are mainly categorized as either reservoir-type drug-delivery devices or matrix-type drug-delivery devices [Citation21]. The drug-release profile of matrix-type drug delivery devices is determined by the diffusion rate of retained drug molecules. It is known that the drug-release profile of matrix-type drug-delivery devices follows the Higuchi release equation ), where the cumulative amount of released drugs (Q) is proportional to the square root of the soaking time (t) [Citation22]. Here, Hk is the Higuchi constant. As shown in , plotting the cumulative amount of DOX released as a function of the square root of the soaking time revealed a linear correlation with a high coefficient of determination (R2) between the cumulative amount of DOX released and the square root of the soaking times.
3.3. Cytotoxicity of samples with respect to Rat-1 cells
shows the DNA concentrations after extraction from Rat-1 cells cultured with 1, 10, or 100 mg of each sample. Samples np-Ti and STXL-20 showed no significant differences in DNA concentrations from those of the control group irrespective of the quantity. When 100 mg of sample ST50-20 was used, the DNA concentration after 7 days of culture decreased significantly compared to the control samples and samples with the same amount of np-Ti or STXL-20. The DNA concentration after 7 days of culture with ST50-20 (10 mg) also decreased significantly compared to the control samples and samples with the same quantity of np-Ti. The DNA concentration after 7 days of culture with 1 mg of ST50-20 was significantly lower, moreover, than that for the same amount of np-Ti.
3.4. Anticancer effects of samples with respect to HeLa cells
shows the viability of HeLa cells cultured for 1–3 days with DOX-loaded STXL-20 samples or DOX solution controls (DOX concentrations in both cases: 0–100 µg·mL−1). After 3 days of culture, the DOX-SOL groups with DOX concentrations of 1, 10, or 100 µg·mL−1 showed 70% inhibition of cell growth, whereas only the DOX-MS group with a DOX concentration of 100 µg·mL−1 showed this level of cell growth inhibition. Cell viability after 1 day of culture was almost the same for the DOX-SOL group with a DOX concentration of 10 µg·mL−1 as for the DOX-MS group with a DOX concentration of 100 µg·mL−1.
4. Discussion
4.1. Structure and properties of samples
Porous microspheres measuring several tens of micrometers in diameter were successfully obtained by the present method, and the number of pores on the surface of the samples could be controlled by changing the concentration of SiNP in the starting solution (). The pores of these samples are believed to be formed by dissolution of the SiNP in NaOH solution [Citation12]. Some samples have cracks on their surfaces. The mechanism of surface crack formation is not completely understood, but surface cracks might be formed to reduce the surface tension of the microspheres and retain their lowest energy [Citation23]. Based on the particle size distributions of the samples (), the sizes of all the samples ranged from roughly 10 to 90 µm, and the mean diameters of all the samples ranged from 27.9 and 33.3 µm, suggesting that the present samples have diameters applicable to TACE. All the samples were composed mainly of anatase, but sample np-Ti contained rutile in addition to anatase ((a)). In samples STXL-10, STXL-20, ST50-10, and ST50-20, which were heated at 600◦C in the presence of SiNPs, the SiNPs may have stabilized the TiO2 particle surfaces to suppress the direct formation of rutile particles and/or may have prevented direct contact between anatase particles and inhibited the phase transformation of anatase to rutile [Citation24,Citation25]. Several IR absorption bands ascribed to Si–O–Si were observed in all the samples except sample np-Ti ((b)), which suggests that a trace amount of SiNPs remains in these samples even after NaOH treatment. The IR absorption bands at around 1100 cm−1 in samples ST50-10 and ST50-20 are more obvious than in samples STXL-10 and STXL-20, indicating that larger amounts of SiNPs remained in samples ST50-10 and ST50-20 than in samples STXL-10 and STXL-20. The broad IR absorption band ascribed to Ti–O and the small IR absorption band ascribed to Si–O–Ti in samples ST50-10 and ST50-20 suggest that partial chemical bonding took place between TiO2 and SiNPs.
The significantly lower SSA and total pore volume of sample np-Ti as compared to those of the other samples () can be attributed to the fact that sample np-Ti lacked pores on its surface, as shown in . It is understood that using higher SiNP concentrations to prepare the samples resulted in higher SSAs and total pore volumes (STXL-20 > STXL-10, and ST50-20 > ST50-10). Smaller SiNPs also showed higher SSAs (STXL-10 > ST50-10, and STXL-20 > ST50-20), but the total pore volume did not increase remarkably with decreases in the SiNP size. We should be careful when comparing STXL-10 with ST50-10 or STXL-20 with ST50-20, because larger numbers of SiNPs remained in samples ST50-10 and ST50-20 than in samples STXL-10 and STXL-20, as shown in ), but this result is also understandable if we assume that the total additive weights (i.e. total additive volumes) of SiNPs were almost the same for sample STXL-10 and sample ST50-10 and for sample STXL-20 and sample ST50-20, and that the SiNPs were uniformly dissolved by NaOH treatment to form pores. The almost total absence of pore size distribution in sample np-Ti () is consistent with the FE-SEM image showing a dense, smooth surface (), but the pore sizes in samples STXL-10 and STXL-20 (≅5 nm) and in samples ST50-10 and ST50-20 (≅ 10 nm) were apparently smaller than those observed by FE-SEM, although the reason is unknown.
All the samples showed negative zeta potentials, but samples STXL-10, STXL-20, ST50-10, and ST50-20 had relatively lower zeta potentials than sample np-Ti (). Residual SiNPs in these samples might have been responsible for the lower zeta potentials. It was reported that a TiO2/SiO2 composite showed a lower zeta potential in neutral solution than TiO2, because the isoelectric point of SiO2 was lower than that of TiO2 [Citation26]. A similar interpretation can be applied to the present samples.
4.2. DOX-adsorption capacity and DOX-release profiles of the samples
Sample STXL-20 showed a DOX-adsorption capacity of 0.14 mg·mg−1 (). This capacity can be converted to 150 mg·mL−1 when we assume that the real density of sample STXL-20 was the same as that of anatase-type TiO2 (3.90 g·cm−3), which was higher than the DOX-adsorption capacity of DC Bead® (40 − 80 mg·mL−1) [Citation6,Citation27]. The mechanism of DOX adsorption on porous TiO2 microspheres can be interpreted as follows. The present samples showed negative zeta potentials (), whereas the DOX molecule was positively charged in aqueous solution by protonation of its amine group [Citation6]. DOX molecules may therefore be adsorbed on sample surfaces due to electrostatic interactions. However, DOX has three potential metal-binding sites [Citation28,Citation29]. One of these sites is the nitrogen atom in the sugar moiety, and the other two are the chelating sites of the quinone and phenolic oxygens on either side of the anthracycline aromatic moiety. DOX molecules might also be chemically adsorbed on TiO2 microspheres, therefore, through their potential metal-binding sites. During chemical adsorption, samples with a higher SSA might adsorb more DOX because only monolayer adsorption is possible. Sample STXL-20 (with a higher SSA) showed higher DOX adsorption than ST50-20, however, although the total pore volume of sample STXL-20 was comparable to that of ST50-20 (). It should be noted here that sample ST50-20 had a higher DOX-adsorption capacity than sample STXL-10, although sample STXL-10 had a higher SSA than sample ST50-20. This result suggests that the DOX-adsorption capacity was affected not only by the SSA, but also by the total pore volume. As discussed above, DOX molecules might be adsorbed on TiO2 by electrostatic interaction as well as by chemical adsorption; hence, positively charged DOX molecules may also be trapped in the surface pores of negatively charged porous TiO2 microspheres by electrostatic attractions.
The present samples released 81.2% of the adsorbed DOX within 5 days (), and their release profile was similar to that of DC bead® (≅ 10%) [Citation30]. The linear correlation between the cumulative amount of DOX released and the square root of the soaking times () indicates that the release of DOX was diffusion-controlled by the soaking time and that the present porous TiO2 microspheres can release DOX and act as matrix-type drug-delivery devices.
4.3. Cytotoxicity of samples for Rat-1 cells
Samples np-Ti and STXL-20 were non-cytotoxic because they showed no significant difference in DNA concentration from the control group, irrespective of quantity (). Large amounts (≥10 mg) of sample ST50-20 were also cytotoxic, and the cell compatibility of sample ST50-20 was lower than that of np-Ti. The mechanism causing the low cell compatibility of sample ST50-20 is still unknown, but the residual SiNPs in sample ST50-20, based on the FT-IR spectrum (), might reduce the cell compatibility. It is interesting to note here that the DNA concentration after 3 days of culture with 100 mg of sample ST50-20 was significantly higher than that in the control group. It was reported that an appropriate amount of silicon (Si) released from akermanite ceramics stimulates L929 fibroblast cell proliferation [Citation31]. We speculate that Si released from sample ST50-20 after 3 days of culture promoted Rat-1 fibroblast cell proliferation, but that the excessive amount of Si released from samples after 7 days of culture inhibited cell proliferation.
4.4. Anticancer effects of samples on HeLa cells
The DOX-SOL groups with DOX concentrations of 1, 10, or 100 µg·mL−1 showed 70% inhibition of HeLa cell growth after 3 days in culture, whereas only the DOX-MS group with a DOX concentration of 100 µg·mL−1 showed the same level of cell growth inhibition (). The HeLa cell viability after 1 day in culture for the DOX-SOL group with a DOX concentration of 10 µg·mL−1 was almost the same as that for the DOX-MS group with a DOX concentration of 100 µg·mL−1. As seen in the release profiles of samples shown in , sample STXL-20 released approximately 7% of the adsorbed DOX into PBS within 1 day. We speculate that using sample STXL-20 with a nominal DOX concentration of 100 µg·mL−1 released a similar fraction of the adsorbed DOX in the culture medium and, hence, showed cell viability similar to that of the DOX-SOL group with a DOX concentration of 10 µg·mL−1. In contrast, the cell viability of the DOX-MS group with a nominal DOX concentration of 10 µg·mL−1 was higher than that of the DOX-SOL group with a DOX concentration of 1 µg·mL−1. This might be because the fraction of DOX released in the DOX-MS group decreased due to the extremely small amount (0.008 mg) of DOX adsorbed onto the sample.
5. Conclusions
Porous TiO2 microspheres with an average diameter of approximately 25 − 30 µm were successfully obtained by the present method. The microspheres adsorbed DOX and released DOX gradually into saline. Our results also showed Rat-1 cell compatibility under controlled administration and antitumour activity with respect to HeLa cells. Thus, the present porous TiO2 microspheres are potential candidate DEBs for TACE.
Acknowledgments
This work was partially supported by JSPS KAKENHI [grant numbers 16H03177 and 18K19895] from the Ministry of Education, Culture, Sports, Science, and Technology, Japan. We thank Dr. M. Kamitakahara of Tohoku University for performing the SSA and pore size-distribution measurements. We also thank Mr. M. Takano and Mr. S. Kanaji of MicrotracBEL Corp. for performing the zeta potential and particle size-distribution measurements.
Disclosure statement
No potential conflict of interest was reported by the authors.
Additional information
Funding
References
- Ferlay J, Shin HR, Bray FD, et al. Estimates of worldwide burden of cancer in 2008: GLOBOCAN 2008. Int J Cancer. 2010;127:2893–2917.
- Kettenbach J, Stadler A, Katzler IV, et al. Drug-loaded microspheres for the treatment of liver cancer: review of current results. Cardiovasc Intervent Radiol. 2008;31:468–476.
- Takayasu K. Transcatheter arterial chemoembolization for unresectable hepatocellular carcinoma: recent progression and perspective. Oncology. 2013;84:28–33.
- Brown KT, Nevins AB, Getrajdman GI, et al. Particle embolization for hepatocellular carcinoma. J Vasc Interv Radiol. 1998;9:822–828.
- Laurent A, Wassef M, Chapot R, et al. Location of vessel occlusion of calibrated tris-acryl gelatin microspheres for tumor and arteriovenous malformation embolization. J Vasc Interv Radiol. 2004;15:491–496.
- Lewis AL, Gonzalez MV, Lloyd AW, et al. DC bead: in vitro characterization of a drug-delivery device for transarterial chemoembolization. J Vasc Interv Radiol. 2006;17:335–342.
- Grosso M, Vignali C, Quaretti P, et al. Transarterial chemoembolization for hepatocellular carcinoma with drug-eluting microspheres: preliminary results from an Italian multicentre study. Cardiovasc Intervent Radiol. 2008;31:141–1149.
- Tanaka T, Nishiofuku H, Hukuoka Y, et al. Pharmacokinetics and antitumor efficacy of chemoembolization using 40 µm irinotecan-loaded microspheres in a rabbit liver tumor model. J Vasc Interv Radiol. 2014;25:1037–1044.
- Lammer J, Malagari K, Vogl T, et al. Prospective randomized study of doxorubicin-eluting-bead embolization in the treatment of hepatocellular carcinoma: results of the PRECISION V study. Cardiovasc Intervent Radiol. 2010;33:41–52.
- Duran R, Sharma K, Dreher MR, et al. A novel inherently radiopaque bead for transarterial embolization to treat liver cancer - a pre-clinical study. Theranostics. 2016;6:28–39.
- Li Z, Miyazaki T, Kawashita M. Preparation and in vitro apatite-forming ability of porous and non-porous titania microspheres. J Ceram Soc Japan. 2013;121:782–787.
- Kawashita M, Tanaka Y, Ueno S, et al. In vitro apatite formation and drug loading/release of porous TiO2 microspheres prepared by sol-gel processing with different SiO2 nanoparticle contents. Mater Sci Engin C. 2015;50:317–323.
- Liu G, Kawashita M, Li Z, et al. Sol-gel synthesis of magnetic TiO2 microspheres and characterization of their in vitro heating ability for hyperthermia treatment of cancer. J Sol-Gel Sci Tech. 2015;75:90–97.
- Kanetaka H, Liu G, Li Z, et al. TiO2 microspheres containing magnetic nanoparticles for intra-arterial hyperthermia. J Biomed Mater Res Part B. 2017;105:2308–2314.
- Li Z, Kawashita M, Kudo T, et al. Sol-gel synthesis, characterization and in vitro compatibility evaluation of iron nanoparticle encapsulating-silica microspheres for hyperthermia of cancer. J Mater Sci Mater Med. 2012;23:2461–2469.
- Xu C, Houck JR, Fan W, et al. Simultaneous isolation of DNA and RNA from the same cell population obtained by laser capture microdissection for genome and transcriptome profiling. J Mol Diagn. 2008;10:129–134.
- Bertoluzza A, Fagnano C, Morelli MA. Raman and infrared spectra on silica gel evolving toward glass. J Non-Cryst Solids. 1982;48:117–128.
- Liu Z, Davis RJ. Investigation of the structure of microporous Ti-Si mixed oxides by X-ray, UV reflectance, FT-Raman, and FT-IR spectroscopies. J Phys Chem. 1994;98:1253–1261.
- Wan M, Li W, Long Y, et al. Electrochemical determination of tryptophan based on Si-doped nano-TiO2 modified glassy carbon electrode. Anal Methods. 2012;4:2860–2865.
- Dutoit DCM, Schneider M, Baiker A. Titania-silica mixed oxides I. influence of sol-gel and drying conditions on structural properties. J Catal. 1995;153:165–176.
- Langer RS, Peppas NA. Present and future applications of biomaterials in controlled drug delivery systems. Biomaterials. 1981;2:201–214.
- Paul DE. Elaborations on the Higuchi model for drug delivery. Int J Pharm. 2011;418:13–17.
- Ye M, Zheng D, Mengye Wang M, et al. Hierarchically structured microspheres for high-efficiency rutile TiO2-based dye-sensitized solar cells. ACS Appl Mater Interfaces. 2014;6:2893−290.
- Suyama S, Kato A. The inhibitory effect of SiO2 on the anatase-rutile transition of TiO2. J Ceram Soc Japan. 1978;86:119–125.
- Okada K, Yamamoto N, Kameshima Y, et al. Effect of silica additive on the anatase-to-rutile phase transition. J Am Ceram Soc. 2001;84:1591–1596.
- Fu X, Clark LA, Yang Q, et al. Enhanced photocatalytic performance of titania-based binary metal oxides: TiO2/SiO2 and TiO2/ZrO2. Environ Sci Technol. 1996;30:647–653.
- Lewis AL, Gonzalez MV, Leppard SW, et al. Doxorubicin eluting beads-1: effects of drug loading on bead characteristics and drug distribution. J Mater Sci Mater Med. 2007;18:1691–1699.
- Chen Y, Wan Y, Wang Y, et al. Anticancer efficacy enhancement and attenuation of side effects of doxorubicin with titanium dioxide nanoparticles. Int J Nanomed. 2011;6:2321–2326.
- Barick KC, Nigam S, Bahadur D. Nanoscale assembly of mesoporous ZnO: a potential drug carrier. J Mater Chem. 2010;20:6446–6452.
- Gonzalez MV, Tang Y, Phillips GJ, et al. Doxorubicin eluting beads-2: methods for evaluating drug elution and in-vitro: in-vivocorrelation. J Mater Sci Mater Med. 2008;19:767–775.
- Wu C, Chang J, Ni S, et al. In vitro bioactivity of akermanite ceramics. J Biomed Mater Res Part A. 2006;76A:73–80.