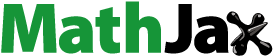
ABSTRACT
Lead-free (1-x) (Bi0.5Na0.5)TiO3-xBi(Mg0.5Ti0.5)O3 or (1-x)BNT-xBMT (x = 0–0.20) piezoelectric ceramics have been investigated for phase evolution, microstructure, dielectric, ferroelectric, piezoelectric, electric field-induced strain, energy storage density, energy harvesting, and magnetic properties. All compositions exhibited high density sintered ceramics (~ 6.13–6.30 g/cm3). With increasing modifier content, the crystal structure changed from rhombohedral to cubic phase. When BMT content was added, the grain size and Tm were found to increase. The x = 0.05 ceramic showed good piezoelectric (low-field d33 = 159 pC/N) and ferroelectric (Pr = 23.84 µC/cm2, Ec = 34.41 kV/cm) properties. The BMT additive also produced an improvement in electric field-induced strain, energy storage efficiency, and magnetic properties. The highest piezoelectric voltage constant (g33 = 26.29 × 10−3 Vm/N) and the off-resonance figure of merit (FoM) for energy harvesting (~ 4.18 pm2/N) were also obtained for the x = 0.05 ceramic, which was ~ 3.4 times (240%) as compared to the pure BNT ceramic. This suggested that the ceramic has a potential to be one of the promising lead-free piezoelectric candidates for further use in piezoelectric energy harvesting applications.
1. Introduction
Lead-based piezoelectric energy harvesters have attracted much attention owing to their high output voltages of 10–30 V, high piezoelectric charge coefficients of 250–900 pC/N, and high piezoelectric voltage coefficient (g33) of 24.89 × 10−3 Vm/N [Citation1–3]. Because of the environmental concerns on PbO toxicity and the new Restriction of Hazardous Substances (RoHS) legislations that have been restricting some lead-based piezoelectrics since 2020, the development of high-electrical performances lead-free piezoceramics is of great urgency [Citation4,Citation5]. Nonetheless, lead-free materials still cannot completely replace lead-based materials in all applications especially high-pressure piezoelectric materials, piezoelectric transformers, or ultrasonic motors. Thus, it became highly intriguing to develop new lead-free materials with improved properties that are suitable for the desired industrial applications [Citation6].
Among several currently available lead-free piezoelectric ceramics, bismuth sodium titanate or Bi0.5Na0.5TiO3 (BNT) is the most representative materials for piezoelectric applications [Citation1] because the BNT exhibits promising electrical features such as relatively high ferroelectricity (Pr = 38 μC/cm2), strong piezoelectric coefficient (d33 ∼ 73–93 pC/N), high Curie point (∼ 320°C), and is induced by the strong hybridization of the 6p orbital of Bi and the 2p orbital of O [Citation7], bringing the possibility to meet the application demands [Citation8]. However, its large coercive field (Ec value more than 70 kV/cm) [Citation8,Citation9] leads to the difficulty to ceramics poling and sufficient domain switching. To deal with these problems, the chemical modifications were employed to tune the phase structure and strain property of BNT-based ceramics, including ion doping/substitution, and the addition of other components, multiple additives, etc [Citation9].
As one of Bi-based perovskites, bismuth magnesium titanate or Bi(Mg0.5Ti0.5)O3 (BMT) ceramic is one of the promising high-temperature Pb-free antiferroelectric materials for future applications because of its antiferroelectric-like displacement of Bi cations and its octahedral tilting characteristics [Citation10]. Khalyavin et al. [Citation11] prepared a single crystal of BMT using a high pressure of 6 GPa. They have concluded that the perovskite BMT is characterized by the orthorhombic Pnnm space group with the unit cell parameters a = 11.3207 Å, b = 5.6433 Å, and c = 7.8314 Å. Purohit et al. [Citation12] fabricated the BMT ceramic by the conventional ceramic technique. Analysis of basic crystal structure using X-ray diffraction data has also exhibited the orthorhombic system as a major phase. In addition, the BMT ceramic showed good stable ferroelectricity [Citation13], and it was used to modify the electrical properties of PbZrO3 [Citation11], BaTiO3 [Citation14–16], and PbTiO3 [Citation17–20], so that the ferroelectricity of these materials could be improved.
The lead-free (K0.5Na0.5)NbO3-Bi(Mg0.5Ti0.5)O3 system was studied by He et al. [Citation13]. They found that when adding BMT, the electrical properties of the KNN ceramic were obviously improved. The optimized piezoelectric and ferroelectric properties with low-field piezoelectric coefficient (d33 = 127 pC/N), planar electromechanical coupling factor (kp = 36.58%), remanent polarization (Pr = 22.1 μC/cm2) were obtained for the KNN-0.01BMT ceramic. Zeb et al. [Citation21] also reported that the addition of Bi(Mg0.5Ti0.5)O3 could increase the depolarization temperature (Td) and piezoelectric performances (d33 = 150 pC/N and the maximum strain (Smax) = 0.35%) of the Bi0.5K0.5TiO3 ceramic. Jing et al. [Citation22] also found that the incorporation of Bi(Mg0.5Ti0.5)O3 (BMT) caused ultrahigh thermally stable dielectric responses in a wide temperature range of the NaNbO3 (NN) ceramic. Especially in the x = 0.03 composition, its permittivity was around 500 at room temperature (RT) and showed a variation less than 4.4% from 30°C to 150°C. In addition, the incorporation of BMT caused the polarization-electric field (P-E) hysteresis loops of the NN-xBMT ceramics to became slender and increased the conversion field. Tian et al. [Citation23] further studied the (1-x)Bi(Mg0.5Ti0.5)O3-xNaNbO3 or (1-x)NN-xBMT lead-free solid solution ceramic. They reported the energy storage properties of the NN were improved by the added BMT. The results obtained in this work suggest that the NN-BMT lead-free antiferroelectric (AFE) solid solution ceramics could be potential candidate dielectrics for the application of energy-storage capacitors in the field of pulsed power electronics [Citation23].
As the above results clearly illustrate, the binary system of (1-x)(Bi0.5Na0.5)TiO3-xBi(Mg0.5Ti0.5)O3 referred to (1-x)BNT-xBMT ceramics is still very interesting. Therefore, in this work, new lead-free of BNT-BMT ceramics were synthesized with the aim of improving their electrical properties. The role of BMT content on the ceramic properties including phase formation, microstructure, electrical (dielectric, ferroelectric, piezoelectric, and electric field-induced strain behavior), and magnetic properties of the BNT ceramic were investigated. Furthermore, since many recent works have focused on the energy storage density and energy harvesting materials due to global energy problems, the energy storage density and energy harvesting behaviors of the presented ceramics were also investigated and discussed in detail in this work.
2. Experimental procedure
The solid-state reaction technique was used to synthesize the (1-x)(Bi0.5Na0.5)TiO3-xBi(Mg0.5Ti0.5)O3 referred to (1-x)BNT-xBMT ceramics (where x = 0, 0.05, 0.10, 0.15, and 0.20 mol fraction). The starting materials used in this study were Bi2O3 (99.9%), Na2CO3 (99%), TiO2 (99.9%), and MgO (99.9%). The Na2CO3 carbonate powder was firstly dried at 120°C for 24 h in order to remove any moisture. The raw materials were stoichiometrically weighed according to the (1-x)BNT-xBMT formula, and then vibratory milled with yttrium stabilized zirconia media for 6 h in an ethanol solution. The slurry was then dried in an oven. After drying, the obtained powder was calcined at 900°C for 2 h at a heating/cooling rate of 5°C/min. Afterward, a few drops of 3 wt% polyvinyl alcohol (PVA) binders were added to the obtained powders before being uniaxially pressed into discs 10 mm in diameter and ~ 1.3 mm in thickness. The green pellets were sintered at 1100–1200°C for 4 h dwell time by using a heating and cooling rate of 5°C/min.
An X-ray diffractometer (Rigaku) was used to identify the phase of both powders and ceramics. The SmartLab Studio II program was used to analyze the phase structure. The tolerance factor (t), lattice parameter (a), unit cell volume, the average ionic radius of the A-site and B-site (rA and rB) values were also calculated in this work. Bulk density was measured in accordance with the Archimedes’ method. The Raman spectra were obtained on polished sintered pellets with a Raman spectrometer (T6400 JY, Horiba Jobin Yvon). A scanning electron microscope (SEM, JEOL JSM- 6335 F) was used to study microstructural features of the ceramics. The grain size of the ceramics was measured by using the linear intercept method (ASTM E112-88). For the electrical measurements, the samples were polished to obtain parallel surfaces before being coated with silver paste on both sides of the ceramics and fired at 700°C for 20 min. Temperature dependence of dielectric properties was carried out using 4284A LCR-meter connected to a high temperature furnace from room temperature (RT) to 500°C with a frequency range of 1–500 kHz. The ferroelectric properties were investigated by a Radiant Precision ferroelectric tester both at RT and high temperatures (HT) (25–150°C). An AC electric field of 50 kV/cm at a frequency of 1 Hz was utilized in the hysteresis measurement. The remanent polarization (Pr), maximum polarization (Pmax), and coercive field (Ec) were determined from the hysteresis loops. By using data from ferroelectric properties, the recoverable energy storage (Wrec) and energy storage coefficient (η) were also calculated. Strain-electric field (S-E) data were taken with the Radiant Technology ferroelectric system in conjunction with the MTI Instruments 2100 Fotonic Sensor, measured with a frequency of 0.1 Hz and electric field of 70 kV/cm. The negative strain (Sneg) and the maximum strain (Smax) were determined from the bipolar S-E curve. The normalized strain coefficient (d*33) was also obtained using the following relationship: d*33 = Smax/Emax. For piezoelectric characterization, all ceramics were poled at RT in silicone oil under a DC electric field of 5 kV/mm for 15 min. The low-field piezoelectric coefficient (d33) was recorded from the 1-day aged samples using a d33 meter at a frequency of 50 Hz. The piezoelectric voltage constant (g33) and the off-resonance figure of merit (FoM) for energy harvesting were also calculated in this work. The magnetic properties were obtained with the magnetic field of −10 kOe ≤ H ≤ 10 kOe using the vibrating sample magnetometer or VSM (Model 7404, Lake Shore Cryotronics, Inc., Westerville, OH, USA).
3. Results and discussion
3.1. Densification and phase analysis
X-ray diffraction patterns of the mixed (1-x)BNT-xBMT calcined powders with 2θ = 20–80° are shown in . In these XRD patterns, it can be seen that a single perovskite structure without any extra or secondary peaks was observed for the x = 0–0.15. This suggests that the BMT effectively diffused into the BNT lattice to form completely solid solution during the sintering process, and that the reagents already reacted to form the end compounds thus releasing CO2 from the carbonates. However, a secondary phase of Na2Ti6O13 (marked as *) was observed with increasing BMT content up to the x = 0.20 composition.
The densification of all ceramics was investigated using Archimedes’ method. Plots of density value as a function of different sintering temperatures from 1100°C to 1200°C are shown in . The data clearly show that the variation of sintering temperature had a significant influence on the ceramic density. At lower sintering temperature of 1100°C, the lower density was observed for all compositions. As the sintering temperature increased, the density value of all ceramics rapidly increased and reached their maximum values at 1150°C for 4 h dwell time. However, after sintering the pellet-shaped samples at a higher temperature of 1175–1200°C, it was found that some ceramics started to twist and melt. This caused a slight decrease in the density value as the temperature went over 1150°C. Therefore, an optimum sintering temperature for all ceramic samples was found to be 1150°C for 4 h dwell time at which all samples had densities ranging from 6.13 to 6.30 g/cm3. Thus, the ceramics sintered at 1150°C were selected for further electrical characterizations. In addition, based on density data at the optimum sintering temperature of 1150°C, the data clearly displayed that the addition of BMT into the BNT ceramic caused an increase in the samples’ density (see ).
Table 1. Physical property, Microstructure, and XRD parameters of the (1-x)BNT-xBMT ceramics analysis by SmartLab Studio II
Figure 2. Plots of density value as a function of sintering temperature (1100–1200°C) of the (1-x)BNT-xBMT ceramics.
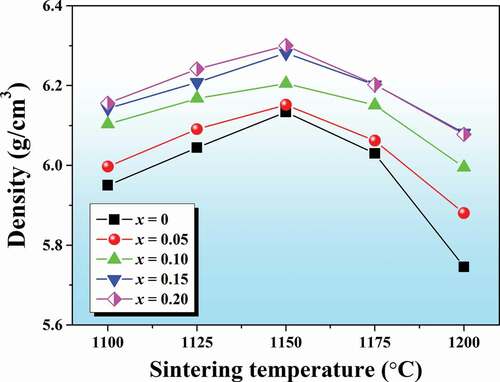
In order to check the phase formation, all ceramics were investigated by the X-ray diffraction technique at RT, and the XRD pattern with 2θ = 20–80° are shown in . Within the detection limits of the XRD instrument, all compositions exhibited a single perovskite phase with no trace of secondary phases. The XRD parameters of all ceramics were analyzed by SmartLab Studio II program, and all data are listed in . The pure BNT ceramic had a rhombohedral structure [Citation24–26]. This can be evidenced by the splitting of (003)R/((021)R peaks at 2θ = 39–41°, and it showed a single (202) peak at 2θ = 45–48° (see ). This result also agrees with the result observed earlier by Difeo et al. [Citation27]. However, with a further increase in the BMT content up to x = 0.15–0.20, the (003)R/((021)R peaks gradually merged into a single one and also showed a single (202) peak at 2θ = 45–48°. This suggests that there was a change from rhombohedral phase to cubic phase as the modifier content was increased [Citation28]. In addition, with an increasing amount of BMT content, the diffraction peaks slightly shifted to lower 2θ angles. The overall shift of XRD patterns was possibly attributed to the differences in the ionic radii between Bi3+ (1.17 Å) and Na+ (1.18 Å) at A-site position and Mg2+ (0.72 Å) and Ti4+ (0.605 Å) at B-site position [Citation29]. This resulted in accordance with an enlargement of lattice parameter a and the unit cell volume, as shown in and ). A similar peak slightly shifted by partial substitution of Ba2+ (1.42 Å) and Sr2+ (1.26 Å) for [Bi0.5(Na0.80K0.20)]2+ which was reported by Jaita et al. [Citation30] in the BNKT-BSrT system. They also found that all peaks shifted to lower 2θ angles could produce an increase in the lattice constant and the unit cell dimension with increasing BSrT fraction.
Figure 4. X-ray diffraction patterns of the (1-x)BNT-xBMT ceramics where x = 0–0.20 with 2θ = 20–80°, 39–41°, and 2θ = 45–48°.
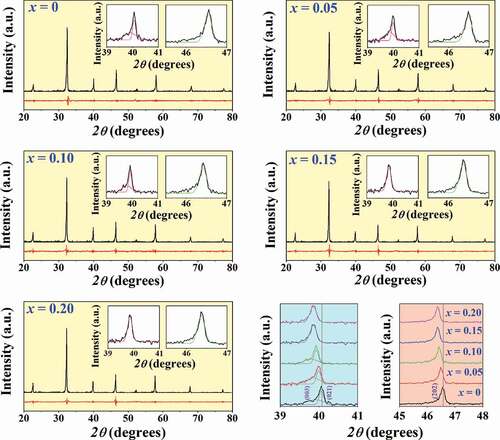
Figure 5. Plots of (a) unit cell volume and tolerance factor (t) values as a function of the BMT content, and (b) the rA and rB as a function of the BMT content of the (1-x)BNT-xBMT ceramics.
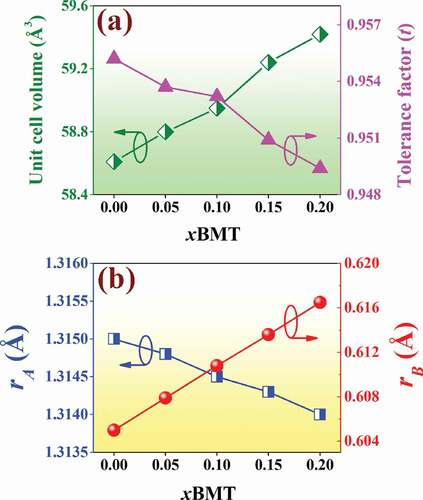
Plots of rA and rB as a function of the BMT content of the (1-x)BNT-xBMT ceramics are shown in ). For the BNT-BMT system, the average ionic radius of A-site (rA) and B-site (rB) can be calculated from the following two equations [Citation31,Citation32]:
where ,
,
, and
are the ionic radii of Bi3+, Na+, Mg2+ and Ti4+, respectively. The rA decreased while the rB increased with increasing modifier content (see ). This was also consistent with the BMT additive that produced a lattice distortion of the BNT ceramic as mentioned above.
The tolerance factor (t) was also calculated in this work. Normally, the concept of tolerance factor (t) describes the stability and relationship between cations and anions in the perovskite structure, which is given by the following equation [Citation32,Citation33]:
where rA,rB and rO are the ionic radii of A, B cations and oxygen for the ABO3 perovskite structure, respectively. However, for the complex perovskite system, rA and rB are the ionic radii of composed ions normalized by the atomic ratio. The ionic radii refer to those reported by Shannon [Citation29]. Normally, the perovskite structure is stable in the region 0.880 < t < 1.090, and the symmetry increases as the t value is close to 1 [Citation33]. In the present work, the t values were calculated to be 0.9494–0.9552 for a composition with x = 0–0.20 ceramics ( and )). Therefore, the t values in the studied materials are found to lie well within the limit indicating the materials belong to stable perovskite structure.
To further investigate the phase formation of the ceramics, a Raman spectroscopy technique was employed. The Raman spectra (from 100 to 1400 cm−1) of the (1-x)BNT-xBMT ceramics measured at RT is presented in . About four regions were detected in the spectra, and they are nearly identical to those previously reported for the BNT-based ceramics [Citation27,Citation34–37]. First, the Raman peak around 139 cm−1 is noted, which can be associated with vibration of A-site cations such as Bi and Na in the ABO3 perovskite structure. Second, the peak at ~ 272 cm−1 can be related to the vibrations of O-Ti-O [Citation27,Citation35]. The Raman peak in the range of 500–650 cm−1 is mainly attributed to the stretching symmetric vibration mode of TiO6 octahedron [Citation37]. Finally, the peak at the wavenumber region containing more than 700 cm−1 suggests the overlapping for longitudinal optical of A1 and E bands [Citation35]. It is clear that with an increase in the BMT content, broadened Raman-active peaks can be found in the bands at ~ 272 and 500–650 cm−1, respectively. The broadened peaks result from the structural disorder in the perovskite lattice, supporting the presence of cubic phase with increasing BMT content [Citation34]. Therefore, this Raman result in the present work agrees with the XRD result which confirmed the phase change from rhombohedral to cubic phase as the modifier content was increasing.
3.2. Microstructure
The microstructures of the (1-x)BNT-xBMT ceramics are presented in ). The average grain size was calculated by the linear intercept method and the value is shown in ). The SEM observation confirmed that all ceramics displayed a highly dense and compact microstructure without a trace of porosity. The pure BNT ceramic showed a dense microstructure with mixed equiaxed and cubic-like grains shape. The addition of BMT allowed a more cubic grains shape. The average grain size increased from 3.00 μm for the pure BNT ceramic to a maximum grain size value of 5.28 μm for the x = 0.20 ceramic, (by approximately 1.76 times), as listed in and ). This implies that the addition of BMT can promote grain growth for the BNT ceramic. The Mg ions, mostly with +2 valence, enter the perovskite lattice to replace the Ti4+ site. Therefore, the imbalance in ion valence leads to the creation of oxygen vacancies, which enhance the transfer of mass and energy between reactants, thus improving sintering behavior and inducing an increase in the grain size value [Citation38]. A similar observation was made in the lead-free ZnO doped Bi0.5Na0.5TiO3 ceramics which were studied by Lee et al. [Citation39]. They reported the ionic radius of Zn2+ (0.74 Å), which is larger than that of Ti4+ (0.605 Å) [Citation29]. When Zn is substituted into Ti4+ sites within the perovskite ABO3 structure, it will create oxygen vacancies. As is generally recognized, the presence of oxygen vacancies is beneficial to mass transport during the sintering process. This is assumed to be responsible for the promoted grain growth as the content of ZnO increases [Citation39].
Table 2. Microstructure, dielectric, ferroelectric, and electric field-induced strain properties of the (1-x)BNT-xBMT ceramics
3.3. Dielectric properties
Temperature dependence of the dielectric constant (εr) and dielectric loss (tan δ) of the (1-x)BNT-xBMT ceramics measured at various frequencies from 1 to 500 kHz are displayed in ). The related dielectric values are also summarized in . Obviously, these ceramics exhibited relaxor ferroelectric characteristics featured by the diffuseness, and frequency-dependent dielectric constant [Citation40]. For the pure BNT ceramic, two dielectric anomalies can be detected, which corroborated dielectric behavior of other BNT ceramics [Citation41]. The first dielectric anomaly was a frequency dispersive hump located at the lower temperature, which was reported to arise from the thermal evolution of R3c and P4bm PNRs [Citation42]. The dielectric maximum (εmax) located at maximum temperature (Tm) was the second dielectric anomaly [Citation41]. The pure BNT ceramic had εmax and Tm values of 3158 and 328°C, respectively. The Tm value in this work was close to 321°C observed earlier by Kantha et al. [Citation28]. It can be seen that the εmax decreased with increasing the additive while the Tm increased with the addition of BMT, accompanied by the obvious broadening of the dielectric peak at Tm (see ). Therefore, the introduction of BMT was believed to induce diffuse phase transitions. This can be evidenced by the calculation of the diffuseness parameter (δA). This equation was used to quantify the degree of diffuseness at the phase transition at Tm, as the following equation [Citation43–45]:
Figure 8. Temperature dependence of the dielectric constant (εr) and dielectric loss (tan δ) of the (1-x)BNT-xBMT ceramics measured at various frequencies from 1–500 kHz where (a) x = 0, (b) x = 0.05, (c) x = 0.10, (d) x = 0.15 (e) x = 0.20, and (f) plot of Tm and εmax values as a function of BMT content (inset: δA as a function of the BMT content).
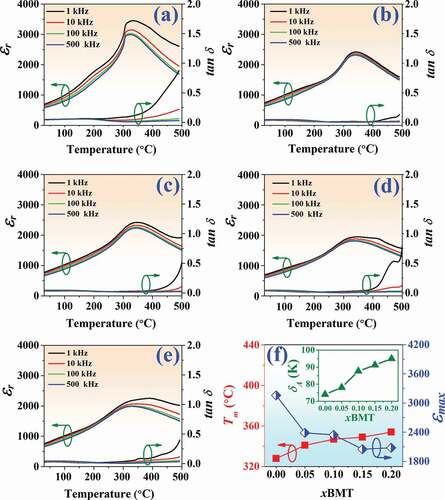
whereδA is the diffuseness parameter, TA and δA are extrapolated parameters, T is the absolute temperature, and ε is the dielectric constant. The δA value was calculated from a plot of ln (εA/ε – 1) versus the ln (T – TA)2, and the value is listed in . Based on the data in , the δA increased with increasing BMT content and reached a maximum value of 94.96 K for the x = 0.20 ceramic. This confirmed that the diffuse-phase transition is stronger for the compositions with a higher BMT content.
3.4. Ferroelectric and energy storage density properties
In this work, the polarization-electric field (P-E) hysteresis loop of the (1-x)BNT-xBMT ceramics, measured at RT under electric field of 50 kV/cm and a frequency of 1 Hz where x = 0–0.20 are plotted in ). The related ferroelectric values of the maximum polarization (Pmax), remanent polarization (Pr), and coercive field (Ec) as a function of BMT content are listed in . Plot of Pr and Ec values as a function of BMT content is also displayed in ). The P-E hysteresis loop for the pure BNT ceramic exhibited a near rhombus shape with the Pmax = 12.31 μC/cm2, Pr = 7.54 μC/cm2, and Ec = 34.54 kV/cm. It should be noted that the Pr and Ec values of this composition were close to the Pr (~ 5.64 μC/cm2) and Ec (~ 36.25 kV/cm) of BNT ceramic which observed previously by Jarupoom et al. [Citation46]. Current vs electric field (I-E) data are also shown in ). However, the addition of BMT into the BNT ceramic slightly affected the shape of the P-E loop and other ferroelectric properties. The Pmax and Pr values increased with increasing BMT content and reached the maximum values of 27.90 and 23.84 μC/cm2, respectively, for the x = 0.05 ceramic, then slightly decreased with further increase in the additive. Moreover, the Ec value decreased with increasing BMT content. It should be noted that the x = 0.05 ceramic has large improvements of Pr and Pmax values (enhanced from the based composition by 216% and 120%, respectively), indicating that this ceramic has a ferroelectric ordering. Many reports have suggested that the large grains have a stronger “binding force”, which will create a more irreversible electric dipole after the cancellation of the external electric field, thus leading to the improvement of the Pr value [Citation47–49].
Figure 9. Polarization-electric field (P-E) hysteresis loop and current-electric field (I-E) data of the (1-x)BNT-xBMT ceramics, measured at 50 kV/cm and a frequency of 1 Hz where (a) x = 0, (b) x = 0.05, (c) x = 0.10,(d) x = 0.15, (e) x = 0.20, and (f) plot of Pr and Ec values as a function of BMT content.
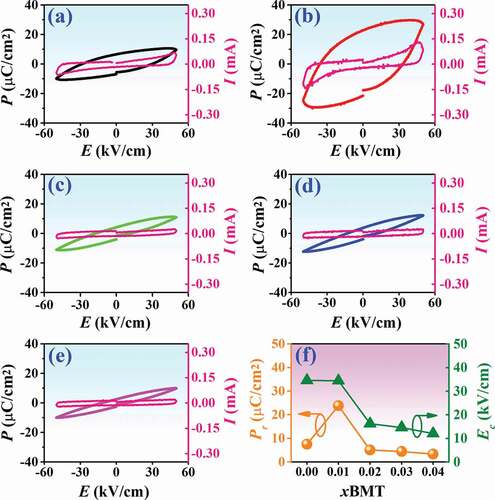
To better understand the ferroelectric behavior of (1-x)BNT-xBMT system, the P-E hysteresis loops were measured above RT. Temperature dependence on P-E hysteresis loops of the (1-x)BNT-xBMT ceramics where x = 0–0.20 are shown in . The effect of temperature (RT – 150°C) on the P-E hysteresis loops were measured under an electric field of 50 kV/cm and a frequency of 1 Hz. All samples exhibited well-saturated P-E loops at RT (25°C). For the pure BNT ceramic, the Pr increased with increasing the temperature from RT to 150°C. However, for the doped-samples, the Pr and Ec values decreased with increasing the temperature up to 150°C. Thus, the temperature can disrupt the ferroelectric order leading to a decrease in the polarization states. Such type of behaviors in the temperature-dependent hysteresis loops was also observed in the other BNT-based materials [Citation25,Citation30,Citation50,Citation51].
Figure 10. Temperature dependence on polarization-electric field (P-E) hysteresis loops of the (1-x)BNT-xBMT ceramics where x = 0–0.20, measured under an electric field of 50 kV/cm and a frequency of 1 Hz.
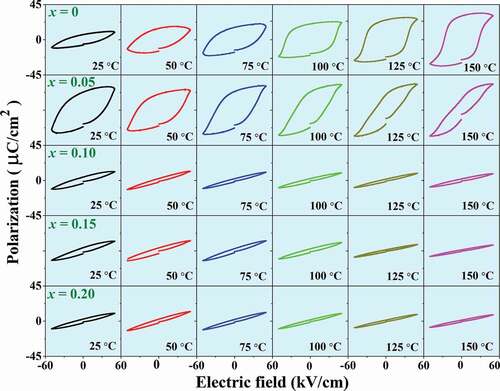
The energy density and efficiency of a dielectric ceramic can be calculated in this work. The energy storage density of any ferroelectric ceramic can be estimated from its P-E hysteresis loops by using the following expression [Citation52–54]:
where Wrec,Wloss and η represent recoverable energy storage density, energy loss density, and energy storage efficiency, respectively. The Pr is remnant polarization, Pmax is the maximum polarization, and E is electric field applied to the ceramic material. Plots of Wrec and η values as a function of the temperature @ E = 50 kV of the studied ceramics are shown in ) and (b), respectively. The related energy storage density values are also summarized in . It can be seen that the Wrec and η values of all ceramics increased with increasing temperatures from RT (25°C) to HT (150°C). At RT, The Wrec and η values increased with increasing BMT content, and reached the maximum values of 0.15 J/cm3 and 47.63%, respectively, for the x = 0.20 ceramic. This indicated that the addition of BMT can help to improve the energy storage density and energy storage efficiency performance of the BNT ceramic. At a high temperature of 125°C and E = 50 kV, the x = 0.05 ceramics exhibited the maximum value of Wrec (0.24 J/cm3) while the η value increased with increasing BMT content and reached the maximum value of 63.92% for the x = 0.20 ceramic. In addition, plots of the Wrec values obtained at 125°C as a function of the electric field (until breakdown strength was reached) are shown in ). At 125°C and the maximum electric field, the x = 0.05 ceramic showed the maximum value of Wrec (0.37 J/cm3). This value is higher than the Wrec value of the x = 0.05 ceramic (0.24 J/cm3), measured at E = 50 kV by 54% (see )). Yu et al. [Citation55] have pointed out that grain size is a factor that affects the Wrec value. They reported that the grain size reduction lowers the Pr and enhanced the dielectric breakdown strength (Eb) leading to a drastic increase of the Wrec value. However, the reason for the improvement of Wrec for the x = 0.05 ceramics in this study was likely due to large Pmax and the highest value of ΔP (ΔP = Pmax – Pr), when compared with those of other samples (see ) [Citation56,Citation57].
Table 3. Energy storage density, piezoelectric, and energy harvesting properties of the (1-x)BNT-xBMT ceramics
Figure 11. Plots of (a) Wrec as a function of temperature @ E = 50 kV, (b) η as a function of temperature @ E = 50 kV, (c) Wrec @ 125°C as a function of the electric field (until breakdown strength reached), and (d) Wrec @ 125°C as a function of BMT content and measured under E = 50 kV and E= Emax of the (1-x)BNT-xBMT ceramics.
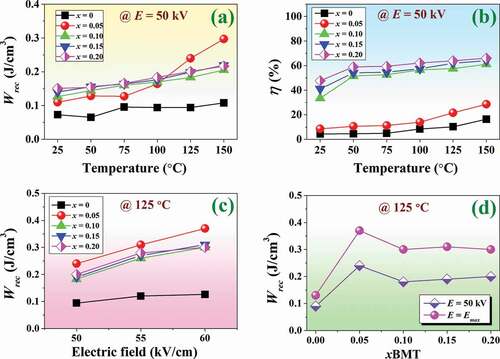
3.5. Electric field-induced strain behavior
Bipolar strain-electric field (S-E) data of the (1-x)BNT-xBMT ceramics, measured under electric field of 70 kV/cm and a frequency of 0.1 Hz where x = 0–0.20 are shown in ). Higher values of applied electric field (> 70 kV) caused the samples to undergo a dielectric breakdown. The maximum strain (Smax), the negative strain (Sneg, i.e. denotes as the difference between zero field strain and the lowest strain, which is closely related to domain back switching during the bipolar cycle) [Citation58] and the normalized strain coefficient (d*33 = Smax/Emax) [Citation59] are summarized in . Plot of Smax and d*33 values as a function of the BMT content is also presented in ). The pure BNT ceramic exhibited a typical ferroelectric behavior with butterfly-shaped strain loop and a visible Sneg related to the domain back switching during bipolar cycles [Citation60]. The Smax,Sneg and d*33 values of the pure BNT ceramic are 0.06%, – 0.02% and 86 pm/V, respectively. With increasing BMT content up to x = 0.05, the Sneg gradually decreased and is accompanied by a concurrent increased in Smax and d*33 to around 0.10% and 143 pm/V, respectively. Meanwhile, the normal butterfly loop drastically changes into the sprout-shaped loop. However, in the present work, a slight decreasing of the Smax and d*33 values as observed at higher BMT content greater than x > 0.05. The Smax and d*33 values were then decreased to the minimum value of 0.05% and 72 pm/V, respectively, for the x = 0.20 ceramic. Therefore, the small amount of BMT additive (x = 0.05) could improve the electric field-induced strain properties of the BNT ceramic.
3.6. Piezoelectric and energy harvesting properties
Plots of low-field piezoelectric coefficients (d33) as a function of BMT content of the (1-x)BNT-xBMT ceramics are shown in and the values are also summarized in . The low-field d33 value of the pure BNT ceramic in this study was 86 pC/N, which was close to 71–72 pC/N observed earlier by Kantha et al. [Citation28]. The low-field d33 value increased with increasing BMT content and reached a maximum value of 159 pC/N for the x = 0.05 ceramic. It should be noted that the low-field d33 value of this composition was higher than the low-field d33 (125 pC/N) of the (1-x)Bi0.5Na0.5TiO3-xBaTiO3 system observed previously by Takenaka et al. [Citation61]. The improvement of low-field d33 for the x = 0.05 ceramic confirmed by the improvement of ferroelectric properties. This can be explained by the thermodynamic theory of ferroelectrics [Citation62]. According to this theory, the low-field d33 can be expressed as:
Figure 13. Plots of low-field d33 and g33 values as a function of BMT content of the (1-x)BNT-xBMT ceramics (the inset shows: FoM as a function of BMT content).
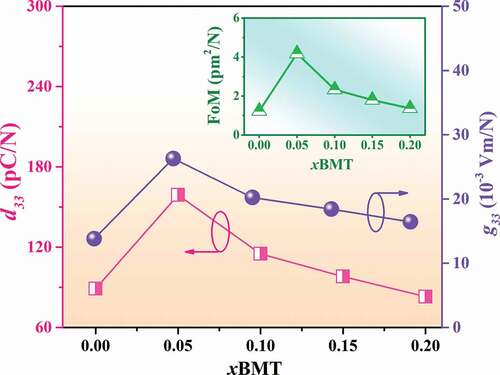
where ε33 represented the dielectric constant of material and ε0 was the vacuum permittivity. The Q11 represented the electrostrictive coefficient, which was constant for the perovskite materials, and Pr was the remnant polarization [Citation62]. It can be seen that the low-field d33 was proportional to the Pr value. For the x = 0.05 ceramic, the Pr drastically increased from 7.54 µC/cm2 to 23.84 µC/cm2 (see ), thus resulted in a significant improvement of the low-field d33 value. With a further increase in the BMT content up to the x = 0.20 ceramic, a decrease in the low-field d33 to a minimum value of 72 pC/N was observed. This was due to the fact that the Pr value in this composition was so small that it results in the reduction of their piezoelectric constant.
At present, the piezoelectric materials are attractive for the development of energy harvesting applications. In this work, the energy harvesting properties of the studied ceramics were carried out. It is known that the piezoelectric voltage constant (g33) is an important factor for an energy harvester [Citation63]. The g33 value was calculated using the following equation [Citation63–67]:
where ε0 is the permittivity of free space, and εr is the dielectric constant. For this equation, the piezoelectric voltage coefficient is dependent on the piezoelectric coefficient and the dielectric constant of the studied material. Furthermore, it was suggested that the sample which has a higher piezoelectric charge constant should generate a higher voltage. Plots of the g33 value as a function of BMT content of the (1-x)BNT-xBMT ceramics are shown in and the values are also summarized in . The g33 value of the pure BNT sample ceramic in this study is 13.82 × 10−3 Vm/N. The small BMT additive produced an increase in the g33 value and then decreased with a further increase in the modifier content that was greater than x > 0.05. The highest g33 value of 26.29 × 10−3 Vm/N was observed for the x = 0.05 ceramic, which was ~ 1.9 times (or 90.23%) as compared to the pure BNT ceramic. It should be noted that the g33 value of this composition was higher than the g33 (25.76 x 10−3 Vm/N) of the 0.99KNLN0.97-xSTx-0.01CZ (x = 0.07) ceramics observed previously by Liu et al. [Citation67]. The trend of g33 value agrees with the trend of low-field d33 value, as shown in . Therefore, the improvement of g33 value for the x = 0.05 ceramic is due to the increase of low-field d33 value.
Normally, a piezoelectric material which has a high figure of merit (FoM) value will generate high power when it is used for energy harvesting. Therefore, in this work, the FoM parameter was evaluated based on the following equation [Citation63,Citation64]:
where ε0 is the permittivity of free space, and εr is the dielectric constant. The d33 and g33 are the low-field piezoelectric coefficients and the piezoelectric voltage constant of the device, respectively. Plots of FoM value as a function of BMT content are shown in the inset of . It was found that the largest improvement for the FoM of 4.18 pm2/N was noted for the x = 0.05 ceramic (see ), which exhibited ~ 3.4 times (or 240%) improvement when compared to the pure BNT ceramic (1.23 pm2/N). This improvement is due to the improvements of the low-field d33 and g33 values for this composition.
Plots of magnetization (M) and magnetic field (H) of the (1-x)BNT-xBMT ceramics, measured at RT and under the magnetic field of −10 kOe ≤ H ≤ 10 kOe are shown in . The x ≤ 0.05 ceramics demonstrated no significant ferromagnetic behavior (based on the resolution of the instrument) with slim and linear hysteresis loop. However, a slight improvement in magnetic behavior for the BMT-added ceramics (x = 0.10–0.20). The increase in the number and size of magnetic region clusters (magnetic contribution) in paramagnetic host (paramagnetic contribution) may be a reason for the magnetic behavior improvement in this work, as expected [Citation68]. The formation of magnetically ordered clusters in solid solutions/composites has been reported in other systems as well [Citation69,Citation70]. Normally, the oxygen vacancy in the ceramic plays an important role in ferromagnetism. For this work, the Mg ions, mostly with +2 valence, enter the perovskite lattice to replace the Ti4+ site. Therefore, the imbalance in ion valence leads to the creation of oxygen vacancies. The oxygen vacancies can locally trap electrons to occupy orbitals interacting with the 3d shell, thus enhancing the ferromagnetism coupling [Citation71]. A similar situation took place with the dilute magnetic semiconductors, such as BNT-Ni-0.025 system, which was reported by Wu et al. [Citation71]. They found that the oxygen octahedral structural distortion induced by the Ni2+ substitution for Ti4+ in the BNT host can partly influence ferromagnetism.
Figure 14. Plots of magnetization (M) and magnetic field (H) of the (1-x)BNT-xBMT ceramics, measured at RT and under the magnetic field of −10 kOe ≤ H ≤ 10 kOe, where (a) x = 0, (b) x = 0.05, (c) x = 0.10,(d) x = 0.15, and (e) x = 0.20.
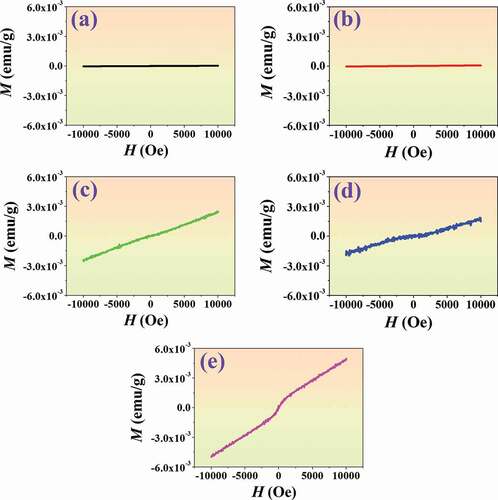
The obtained results suggested that the BMT additive not only enhanced the dielectric, ferroelectric, electric field-induced strain, energy storage density, and magnetic performances but also improved the energy harvesting properties of the studied ceramics. As seen in the previous sections, the addition of BMT provided many advantages to the studied ceramics. Therefore, the addition of BMT with a right content may be a good strategy to improve many electrical properties of other piezoelectric lead-free ceramics.
4. Conclusion
In this work, the (1-x)(Bi0.5Na0.5)TiO3-xBi(Mg0.5Ti0.5)O3 referred to (1-x)BNT-xBMT ceramics (x = 0, 0.05, 0.10, 0.15, and 0.20 mol fraction) have been successfully synthesized using a conventional mixed-oxide route. Phase evolution, microstructure, dielectric, piezoelectric, energy storage density, and energy harvesting properties of all ceramics were examined. There was a change from rhombohedral to cubic phase with an increase of the modifier content. The increase of grain size was observed and the densification was found to improve when the modifier was added. The x = 0.05 ceramic showed good ferroelectric (Pr = 23.84 µC/cm2, Ec = 34.41 kV/cm) and piezoelectric (low-field d33 = 159 pC/N). The incorporation of the BMT significantly improved the electric field-induced strain, magnetic properties, and energy storage efficiency values of the BNT ceramic. The highest g33 of 26.29 × 10−3 Vm/N and the FoM of 4.18 pm2/N were obtained for the x = 0.05 ceramic, which suggests that this ceramic has a potential to be one of the promising lead-free piezoelectric candidates for further use in piezoelectric energy harvesting applications.
Acknowledgments
This work was supported by Office of Research Administration, Chiang Mai University, Department of Physics and Materials Science, Faculty of Science, Chiang Mai University, and Department of Industrial Engineering, Faculty of Engineering, Rajamangala University of Technology Lanna. Materials and Manufacturing Research Center, Faculty of Engineering, Rajamangala University of Technology Lanna is also acknowledged.
Disclosure statement
No potential conflict of interest was reported by the author(s).
References
- Kang WS, Koh JH. (1-x)Bi0.5Na0.5TiO3-xBaTiO3 lead-free piezoelectric ceramics for energy-harvesting applications. J Eur Ceram Soc. 2015;35(7):2057–2064.
- Swallow LM, Luo JK, Siores E, et al. A piezoelectric fibre composite based energy harvesting device for potential wearable applications. Smart Mater Struct. 2008;17(2):025017.
- Shin DJ, Kang WS, Koh JH, et al. Comparative study between the pillar and bulk-type multilayer structures for piezoelectric energy harvesters. Phys Status Solidi A. 2014;211(8):1812–1817.
- Liang G, Zhang Y, Zhu J, et al. Tailoring and improving the strong-electric-field electrical properties of the BNT-BT ferroelectric ceramics by a functional-group-doping. Ceram Int. 2021;47(5):6584–6590.
- Yan B, Fan H, Wang C, et al. Giant electro-strain and enhanced energy storage performance of (Y0.5Ta0.5)4+ co-doped 0.94(Bi0.5Na0.5)TiO3-0.06BaTiO3 lead-free ceramics. Ceram Int. 2020;46(1):281–288.
- Vuong LD, Gio PD. Enhancement in dielectric, ferroelectric, and piezoelectric properties of BaTiO3-modified Bi0.5(Na0.4K0.1)TiO3 lead-free ceramics. J Alloys Compd. 2020;817:152790.
- Wang H, Hu Q, Liu X, et al. A high-tolerance BNT-based ceramic with excellent energy storage properties and fatigue/frequency/thermal stability. Ceram Int. 2019;45(17):23233–23240.
- He H, Lu W, Jas O, et al. Probing the coexistence of ferroelectric and relaxor states in Bi0.5Na0.5TiO3 -based ceramics for enhanced piezoelectric performance. ACS Appl Mater Interfaces. 2020;12(27):30548–30556.
- Wang J, Du Y, Li Z, et al. High permittivity and low dielectric loss of (1-x)Bi0.5(Na0.48K0.52)0.5TiO3-xBaZrO3 lead-free ceramics. J Mater Sci: Mater Electron. 2020;31:10038–10046.
- Schwertfager N, Pandech N, Suewattana M, et al. Calculated XANES spectra of cation off-centering in Bi(Mg0.5Ti0.5)O3. Ferroelectrics. 2016;490(1):159–166.
- Khalyavin DD, Salak AN, Vyshatko NP, et al. Crystal structure of metastable perovskite Bi(Mg1/2Ti1/2)O3: bi-based structural analogue of antiferroelectric PbZrO3. Chem Mater. 2006;18(21):5104–5110.
- Purohit V, Padhee R, Choudhary RNP. Structural and electrical properties of Bi(Mg0.5Ti0.5)O3 ceramic. J Mater Sci.: Mater Electronics. 2018;29:5224–5232.
- He F, Chen X, Chen J, et al. (K0.5Na0.5)NbO3-Bi(Mg0.5Ti0.5)O3 solid solution: phase evolution, microstructure and electrical properties. J Mater Sci: Mater Electron. 2013;24:4346–4350.
- Xiong B, Hao H, Zhang S, et al. Structure, dielectric properties and temperature stability of BaTiO3-Bi(Mg1/2Ti1/2)O3 perovskite solid solutions. J Am Ceram Soc. 2011;94(10):3412–3417.
- Sun R, Wang X, Shi J, et al. Dielectric and polar order behaviors of BaTiO3-Bi(Mg1/2Ti1/2)O3 ceramics. Appl Phys A. 2011;104(1):129–133.
- Si F, Tang B, Fang Z, et al. Nb-Doped 0.8BaTiO3-0.2Bi(Mg0.5Ti0.5)O3 ceramics with stable dielectric properties at high temperature. Crystals. 2017;7(6):168.
- Rai R, Sinha A, Sharmac S, et al. Investigation of structural and electrical properties of (1-x)Bi0.5Mg0.5TiO3-(x)PbTiO3 ceramic system. J Alloys Compd. 2009;486(1–2):273–277.
- Ansell TY, Nikkel J, Cann DP, et al. High temperature piezoelectric ceramics based on xPbTiO3-(1-x)Bi(Sc1/2Me1/4Ti1/4)O3 (Me = Zn, Mg) ternary perovskites. Jpn J Appl Phys. 2012;51:101802.
- Suchomel MR, Davies PK. Predicting the position of the morphotropic phase boundary in high temperature PbTiO3-Bi(B′B″)O3 based dielectric ceramics. J Appl Phys. 2004;96(8):4405–4410.
- Sharma S, Rai R, Hall DA, et al. Nonlinear ferroelectric and dielectric properties of Bi(Mg0.5Ti0.5)O3-PbTiO3 perovskite solid solutions. Adv Mat Lett. 2012;3(2):92–96.
- Zeb A, Hall DA, Milne SJ. Lead-free piezoelectric K0.5Bi0.5TiO3-Bi(Mg0.5Ti0.5)O3 ceramics with depolarisation temperatures up to ~ 220 °C. J Mater Sci: Mater Electron. 2015;26:9516–9521.
- Jing R, Jin L, Tian Y, et al. Bi(Mg0.5Ti0.5)O3-doped NaNbO3 ferroelectric ceramics: linear regulation of Curie temperature and ultra-high thermally stable dielectric response. Ceram Int. 2019;45(17):21175–21182.
- Tian A, Zuo R, Qi H, et al. Large energy-storage density in transition-metal oxide modified NaNbO3-Bi(Mg0.5Ti0.5)O3 lead-free ceramics through regulating the antiferroelectric phase structure. J Mater Chem A. 2020;8(17):8352–8359.
- Yoneda Y, Noguchi Y. Nanoscale structural analysis of Bi0.5Na0.5TiO3. Jpn J Appl Phys. 2020;59(SP): PA01.
- Lin D, Kwok KW, Chan HLW. Structure and electrical properties of Bi0.5Na0.5TiO3-BaTiO3-Bi0.5Li0.5TiO3 lead-free piezoelectric ceramics. Solid State Ion. 2008;178:1930–1937.
- Nesterović A, Vukmirović J, Stijepović I, et al. Structure and dielectric properties of (1-x)Bi0.5Na0.5TiO3–xBaTiO3 piezoceramics prepared using hydrothermally synthesized powders. R Soc Open Sci. 2021;8(7):202365.
- Difeo M, Osinaga S, Febbo M, et al. Influence of the (Bi0.5Na0.5)TiO3-BaTiO3 lead-free piezoceramic geometries on the power generation of energy harvesting devices. Ceram Int. 2021;47(8):10696–10704.
- Kantha P, Pisitpipathsin N. Effect of KNbO3 addition on diffuse phase transition and dielectric properties of Bi0.5Na0.5TiO3 ceramics. Int Ferroelectrics. 2018;187(1):129–137
- Shannon RD. Revised effective ionic radii and systematic studies of interatomie distances in halides and chaleogenides. Acta Cryst. 1976;A32(5):751–767.
- Jaita P, Watcharapasorn A, Kumar N, et al. Lead-Free (Ba0.70Sr0.30)TiO3-Modified Bi0.5(Na0.80K0.20)0.5TiO3 ceramics with large electric field-induced strains. J Am Ceram Soc. 2016;99(5):1615–1624.
- Jaita P, Jarupoom P, Yimnirun R, et al. Phase transition and tolerance factor relationship of lead-free (Bi0.5K0.5)TiO3-Bi(Mg0.5Ti0.5)O3 piezoelectric ceramics. Ceram Int. 2016;42(14):15940–15949.
- Vittayakorn N, Rujijanagul G, Tunkasiri T. Perovskite phase formation and ferroelectric properties of the lead nickel niobate-lead zinc niobate-lead zirconate titanate ternary system. J Mater Res. 2003;18(12):2882–2889.
- Rao KS, Rajulu KCV, Tilak B, et al. Effect of Ba2+ in BNT ceramics on dielectric and conductivity properties. Nat Sci. 2010;2(4):357–367.
- Wang J, Zhou C, Li Q, et al. Dual relaxation behaviors and large electrostrictive properties of Bi0.5Na0.5TiO3-Sr0.85Bi0.1TiO3 ceramics. J Mater Sci. 2018;53(12):8844–8854.
- Zhong M, Feng Q, Yuan C, et al. Photocurrent density and electrical properties of Bi0.5Na0.5TiO3-BaNi0.5Nb0.5O3 ceramics. J Adv Ceram. 2021;10(5):1119–1128.
- Rout D, Moon KS, Kang SJL, et al. Dielectric and Raman scattering studies of phase transitions in the (100-x)Na0.5Bi0.5TiO3-xSrTiO3 system. J Appl Phys. 2010;108(8):084102.
- Prasertpalichat S, Siritanon T, Nuntawong N, et al. Structural characterization of A-site nonstoichiometric (1-x)Bi0.5Na0.5TiO3-xBaTiO3 ceramics. J Mater Sci. 2019;54(2):1162–1170.
- Li L, Fang D, Wang RX, et al. Domain evolution and improved electrical property of BiMn2/3Nb1/3O3 doped 0.8Na0.5Bi0.5TiO3-0.2K0.5Bi0.5TiO3. Ceram Int. 2020;46(6):7947–7953.
- Lee YC, Lee TK, Jan JH. Piezoelectric properties and microstructures of ZnO-doped Bi0.5Na0.5TiO3 ceramics. J Eur Ceram Soc. 2011;31(16):3145–3152.
- Zhou X, Yan Z, Qi H, et al. Electrical properties and relaxor phase evolution of Nb-modified Bi0.5Na0.5TiO3-Bi0.5K0.5TiO3-SrTiO3 lead-free ceramics. J Eur Ceram Soc. 2019;39:2310–2317.
- Zhou X, Qi H, Yan Z, et al. Superior thermal stability of high energy density and power density in domain-engineered Bi0.5Na0.5TiO3 NaTaO3 relaxor ferroelectrics. ACS Appl Mater Interfaces. 2019;11(46):43107–43115.
- Jo W, Schaab S, Sapper E, et al. On the phase identity and its thermal evolution of lead free (Bi1/2Na1/2)TiO3−6 mol% BaTiO3. J Appl Phys. 2011;110(7):074106.
- Ke S, Fan H, Huang H, et al. Lorentz-type relationship of the temperature dependent dielectric permittivity in ferroelectrics with diffuse phase transition. Appl Phys Lett. 2008;93(11):112906.
- Fang P, Xi Z, Long W, et al. Structural and dielectric relaxor behavior of Ba1-xNdxBi4Ti4O15 ceramics. Solid State Commun. 2016;231-232:1–5.
- Bokov AA, Ye ZG. Phenomenological description of dielectric permittivity peak in relaxor ferroelectrics. Solid State Commun. 2000;116(2):105–108.
- Jarupoom P, Jaita P. Enhanced magnetic performance of lead-free (Bi0.5Na0.5)TiO3-CoFe2O4 magnetoelectric ceramics. Electron Mater Lett. 2015;11(5):788–794.
- Li Q, Wang J, Ma Y, et al. Enhanced energy-storage performance and dielectric characterization of 0.94Bi0.5Na0.5TiO3-0.06BaTiO3 modified by CaZrO3. J Alloys Compd. 2016;663:701–707.
- Randall CA, Kim N, Kucera JP, et al. Intrinsic and extrinsic size effects in fine-grained morphotropic-phase-boundary lead zirconate titanate ceramics. J Am Ceram Soc. 1998;81(3):677–688.
- Mudinepalli VR, Feng L, Lin WC, et al. Effect of grain size on dielectric and ferroelectric properties of nanostructured Ba0.8Sr0.2TiO3 ceramics. J Adv Ceram. 2015;4(1):46–53.
- Jaita P, Watcharapasorn A, Kumar N, et al. Large electric field-induced strain and piezoelectric responses of lead-free Bi0.5(Na0.80K0.20)0.5TiO3-Ba(Ti0.90Sn0.10)O3 ceramics near morphotropic phase boundary. Electron Mater Lett. 2015;11(5):828–835.
- Jaita P, Watcharapasorn A, Cann DP, et al. Dielectric, ferroelectric and electric field-induced strain behavior of Ba(Ti0.90Sn0.10)O3-modified Bi0.5(Na0.80K0.20)0.5TiO3 lead-free piezoelectrics. J Alloys Compd. 2014;596:98–106.
- Chauhan A, Patel S, Vaish R. Mechanical confinement for improved energy storage density in BNT-BT-KNN leadfree ceramic capacitors. AIP Adv. 2014;4(8):087106.
- Prado A, Camargo J, Öchsner P, et al. Synthesis and characterization of Bi0.5Na0.5TiO3-BaTiO3-K0.5Na0.5NbO3 ceramics for energy storage applications. J Electroceram. 2020;44(3–4):248–255.
- Yu Y, Zhang Y, Zhang Y, et al. High-temperature energy storage performances in (1-x)(Na0.50Bi0.50TiO3)-xBaZrO3 lead-free relaxor ceramics. Ceram Int. 2020;46(18):28652–28658.
- Yu Z, Zeng J, Zheng L, et al. Microstructure effects on the energy storage density in BiFeO3-based ferroelectric ceramics. Ceram Int. 2021;47(9):12735–12741.
- Huang Y, Li F, Hao H, et al. (Bi0.51Na0.47)TiO3 based lead free ceramics with high energy density and efficiency. J Materiomics. 2019;5(3):385–393.
- Yang H, Yan F, Lin Y, et al. A lead free relaxation and high energy storage efficiency ceramics for energy storage applications. J Alloys Compd. 2017;710:436–445.
- Malik RA, Hussain A, Maqbool A, et al. Temperature-insensitive high strain in lead-free Bi0.5(Na0.84K0.16)0.5TiO3−0.04SrTiO3 ceramics for actuator applications. J Am Ceram Soc. 2015;98(12):3842–3848.
- Jaita P, Jarupoom P. Enhanced electric field-induced strain and electrostrictive response of lead-free BaTiO3 -modified Bi0.5(Na0.80K0.20)0.5TiO3 piezoelectric ceramics. J Asian Ceram Soc. 2021;9(3):975–987.
- Li L, Hao J, Chu R, et al. Dielectric, ferroelectric and field-induced strain response of lead-free (Fe,Sb)-modified (Bi0.5Na0.5)0.935Ba0.065TiO3 ceramics. Ceram Int. 2016;42(8):9419–9425.
- Takenaka T, Maruyama K, Sakata K. (Bi1/2Na1/2)TiO3 -BaTiO3 system for lead-free piezoelectric ceramics. Jpn J Appl Phys. 1991;30(Part 1, No. 9B):2236–2239.
- Ullah A, Ahn CW, Hussain A, et al. Structural transition and large electric field-induced strain in BiAlO3-modified Bi0.5(Na0.8K0.2)0.5TiO3 lead-free piezoelectric ceramics. Solid State Com. 2010;150(25–26):1145–1149.
- Shin DJ, Kim J, Koh JH. Piezoelectric properties of (1-x)BZT-xBCT system for energy harvesting applications. J Eur Ceram Soc. 2018;38(13):4395–4403.
- Choi YJ, Yoo MJ, Kang HW, et al. Dielectric and piezoelectric properties of ceramic-polymer composites with 0-3 connectivity type. J Electroceram. 2013;30(1–2):30–35.
- Shin DJ, Koh JH. Comparative study on storing energy for (Ba,Zr)TiO3 and CuO-(Ba,Zr)TiO3 ceramics for piezoelectric energy harvesting applications. Ceram Int. 2017;43:S649–S654.
- Zhao H, Hou Y, Yu X, et al. A wide temperature insensitive piezoceramics for high temperature energy harvesting. J Am Ceram Soc. 2019;102(9):5316–5327.
- Liu B, Li P, Shen B, et al. Simultaneously enhanced piezoelectric response and piezoelectric voltage coefficient in textured KNN-based ceramics. J Am Ceram Soc. 2018;101(1):265–273.
- Mishra KK, Satya AT, Bharathi A, et al. Vibrational, magnetic, and dielectric behavior of La-substituted BiFeO3 -PbTiO3. J Appl Phys. 2011;110(12):123529.
- Wang Y, Pu Y, Shi Y, et al. Ferroelectric, magnetic, magnetoelectric properties of the Ba0.9Ca0.1Ti0.9Zr0.1O3/CoFe2O4 laminated composites. J Mater Sci: Mater Electron. 2017;28:11125–11131.
- Rani J, Yadav KL, Prakash S. Dielectric and magnetic properties of xCoFe2O4-(1-x)[0.5Ba(Zr0.2Ti0.8)O3-0.5(Ba0.7Ca0.3)TiO3] composites. Mater Res Bull. 2014;60:367–375.
- Wu X, Liu C, Tse MY, et al. Luminescent-electrical-magnetic performances of sol-gel-derived Ni2+-modified Bi0.5Na0.5TiO3. J Mater Sci: Mater Electron. 2017;28:12021–12025.