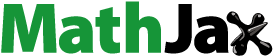
ABSTRACT
Although heavy metal pollution significantly impacts living organisms, the monitoring biomarkers can guide remediation. This study examined the response of Pb²⁺, Cd²⁺, Cu²⁺, Zn²⁺, Cr⁶⁺, Ni²⁺, and Hg²⁺ to soil microorganisms. Bacterial counts were measured at three metal concentrations (T₁, T₂, and T₃). Results revealed that Hg²⁺-T₁ caused a 91.4% reduction in bacterial counts within 10 days, while the other metals reduced counts by 32.9% to 71.8%. Higher concentrations (T₂ and T₃) of all metals, except Ni²⁺ (48.8%) at T₂, reduced counts by 92.1% to 99.6%. After 40 days, reductions were 74.5% for Hg²⁺-T₁, 97.0% for Cr6+-T2, and 95.2 to 98.0 % for Hg2+, Cr6+, Cu2+ and Zn2+-T3. Intrinsically resistant bacteria were observed after 40 days, constituting 34.2%–71.8% in T₁ of most metals, except Hg²⁺ (25.5%). These percentages decreased in T2 and T3. 55.3% of the initial organic-N was oxidized after 20 days in untreated soil, while Pb²⁺ was the least toxic for ammonification, and Hg²⁺ was the highest. In untreated soil, 67.4% of ammoniacal-N was oxidized after 20 days. Nitrification was unaffected by T₁, except for Hg²⁺. At T₂, Hg²⁺, Ni²⁺, and Cd²⁺ were the most suppressive, while at T₃, Hg²⁺, Zn²⁺, and Cd²⁺ showed the most harmful effects.
Introduction
Rapid technological progress, the growth of the world’s population, and the consumption of natural resources to meet food, energy, and other needs have upset the balance of nature. Pollution has become one of the world’s most important problems since the industrial revolution. Water, soil, and air pollution are the main types of pollution caused by various emerging pollutants that threaten the health and well-being of millions of people and global ecosystems [Citation1]. From an ecological perspective, soil is a fundamental resource for human life. It is the most basic element of human production and the carrier that can connect various human economic relations [Citation2]. Thus, soil pollution has become a global risk that directly threatens all soil functional parameters [Citation3] as soil is the link for organic pollutants and heavy metals from crops to humans and their bioaccumulation along the food chain increases the potential risks to human health.
Anthropogenic activities such as mining and metal smelting have increased heavy metals in soils and food crops cultivated within industrial areas [Citation4]. They added that well-developed transportation and agricultural activities are also important sources of heavy metals. The soil quality of arable and cultivated lands near industrial and abandoned multiple metal mines is concerning. As reported by the Ministry of Environment Protection [Citation5] about 16.1% of soils, including 19.4% of the arable lands and 36.3% of enterprise surrounding soils were over the Grade II of the environment quality standard.
Heavy metals are one of the largest sources of pollution in the environment and have increased dramatically in recent years due to various human activities such as agriculture, mining, and other industrial processes [Citation6]. They generally refer to a metal with a density greater than 5.0 g/cm3, including 45 elements such as Pb2+, Cu2+, Zn2+, Cd2+, and Hg2+, which were common and important refractory pollutants. Moreover, they are usually present in relatively low concentrations in soil, whereas they are known to be toxic to most organisms when present in too high concentrations. Consequently, the higher concentrations of these elements above threshold levels have a very detrimental effect on microbial communities and their vital activities. Moreover, once they enter the soil, they become irreversibly immobilized soil components. From a biochemical point of view, heavy metals in soil can be divided into two categories. One is harmful to plants, humans, microorganisms, and animals, such as Pb2+, Cd2+, and Hg2+; the other is beneficial when present in constant amounts, but when present in excess it damages biological activity, such as Cu2+ and Zn2+.
The toxicity of heavy metals to microorganisms, as mentioned by [Citation7,Citation8] is primarily about the bioavailability of metals, i.e. the amount of the metal eventually absorbed by the microorganism. In addition, heavy metals and microorganisms have a strong affinity to bind easily with some biological macromolecules such as the activity center of enzymes and electron-donating groups, nucleic acid bases, and phosphate combinations, leading to their inactivation. Recently [Citation9] added that once heavy metals enter the soil, they primarily affect the number of soil bacteria, fungi, actinomycetes, and other microbial populations as they affect microbial quality and quantity. Their contamination can lead to different microbial community patterns. Long-term soil contamination with heavy metals will select microorganisms that can adapt specifically to the contaminated soil. The higher the organic carbon content in heavily polluted soils, the lower the efficiency of microbial populations in organic mineralization. This may be a simple indication of the impact of heavy metal pollution on soil microbial communities and biological activities.
In addition, as a key limiting nutrient in terrestrial ecosystems, nitrogen plays an important role in determining soil quality [Citation10]. It is an essential nutrient for plant growth and ecosystem functioning, and its cycling is closely related to soil microbial activity and plant productivity [Citation11,Citation12]. However, heavy metals in soil can affect the nitrogen cycle by altering soil microbial communities, absorbing nitrogen, and changing nitrogen transformation pathways. As soil heavy metal pollution can inhibit the activity of nitrogen-fixing bacteria, which play an important role in the nitrogen cycle, this can lead to lower nitrogen availability in the soil and negatively affect plant growth and soil productivity [Citation13].
[Citation14,Citation15] found that microbial parameters appear as very useful in monitoring soil pollution by heavy metals. These parameters can be divided into three main groups: The first measures the size of the microbial population at the individual organism, functional group, or whole population level, the second measures the activity of the whole microbial population, e.g. C and N mineralization, and the third option is a combination of activity and biomass data that provides specific activities of the microbial population.
Microbial parameters serve as sensitive indicators of soil pollution with heavy metals, providing early detection of the impact of heavy metals on soil health by evaluating changes in microbial populations and activities. Moreover, soil monitoring reveals how heavy metals shape the structure and function of microbial communities, highlighting shifts toward metal-tolerant species. One of the most sensitive indicators for heavy metal soil pollution is nitrogen transformations, such as ammonification and nitrification, which are vital for soil fertility and ecosystem function. This provides insights into the dynamics of soil ecosystems under heavy metal stress, aiding in understanding long-term contamination effects and informing the development of strategies for sustainable soil management and effective remediation techniques. Finally, all of these parameters contribute to broader environmental health assessments by linking soil microbial health with ecosystem services.
From this point of view, the present work was carried out to evaluate the effects of soil pollution with elevated concentrations of seven heavy metals (Pb2+, Cd2+, Cu2+, Zn2+, Cr6+, Ni2+, and Hg2+) on the microbial population density and some microbial activities, as sensitive indicators of heavy metal pollution in soil. The changes in the total microbial population were estimated at regular intervals and the nitrogen transformation processes (ammonification and nitrification), affected by heavy metals stress, were determined as well.
Materials and methods
Heavy metals
Seven heavy metals were used: Pb2+ as Pb(CH,COO)2.3 H₂O, Cd2+ as CdCl2 H₂O, Cu2+ as CuSO4.5 H₂O, Zn2+ as ZnSO4.7 H2O, Cr6+ as K2Cr₂O7, Ni2+ as NiCl2.6 H₂O and Hg2+ as HgCl2. Standard stock solutions of each metal salt were prepared and used to supplement either soil or culture media to achieve the desired metal-ion concentrations.
Soil sampling
Soil samples were collected from an experimental farm in the Faculty of Agriculture, Cairo University at Giza, Egypt. This site is specialized for field experiments and research, so it doesn’t provide any treatments e.g. pesticides or fertilizers. Moreover, the experimental field is away from any source of pollution which makes it considered uncontaminated soil. The fertile clay-loam soil (top 10 cm layer) was obtained, air-dried and 2 mm sieved in preparation for the experimental part.
Chemical determinations
Ammoniacal-, nitrate- and total-inorganic nitrogen were determined according to [Citation16].
Determination of NH4+-N
40 g of a representative soil sample was mixed with 1 M KCl, shaken for 1 hour and the suspension filtered. 50 ml of the extract was distilled in the presence of 0.5 g MgO and 10 ml boric acid and titrated against 0.01 M HCl with methyl red-bromocresol green indicator. The blank test was carried out with 50 ml 1 M KCl solution. The determined titration should be subtracted from the sample titration to obtain a corrected titration volume.
Determination of NO3−-N
After NH4+-N distillation, the solution was cooled, provided with 0.5 g Devara’s alloy, and the distillation was continued. The distillate was then received in 5 ml boric acid and titrated against 0.01 M HCL using methyl red-bromocresol green indicator. Blank was carried out using 50 ml of 1 M KCl solution. The determined titration should be subtracted from the sample titration to obtain a corrected titration volume.
Determination of total mineral-N
Total mineral-N was determined by adding 1 drop of Octan-2-ol, 0.5 g of Devarda’s alloy followed by 0.5 g MgO and the procedure was carried out as described above.
Calculations:
NH4+-N, NO3−-N, and total mineral-N were each calculated using the following equation:
Data analysis
In the present study, the results were presented as means using Microsoft Excel from the Microsoft Office 365 suite.
Experiments
Effect of soil pollution with heavy metals on the total microbial population
A laboratory experiment was conducted to evaluate the effects of seven heavy metals on the total microbial population by determining the periodic changes in the microbial counts and the metal(s) resistant ones, as well.
Soil was distributed in glass jars at the rate of 150 g and supplemented with different concentrations of each of the test heavy metals. Three jars were prepared for each treatment as replicates. After that, soil moisture was adjusted at 75% water holding capacity (WHC) using distilled water with thorough mixing and incubated at 28°C for 40 days.
In addition to the control (untreated) soil (C), three other treatments were prepared using the standard stock salt solutions. The first treatment was T1 (low-metal concentration); in which the soil was supplied with 25 µg.g−1soil of each of the seven metals, the second one was T2 (moderate-metal concentration); it included the addition of 250 µg.g−1 soil of Pb2+, Cd2+, Cu2+ and Zn2+, 1000 µg.g−1 soil of Cr6+ and Ni2+ and 500 µg.g−1 soil of Hg2+, and the third one was T3 (high-metal concentration): comprised the addition of 500 µg.g−1 soil of Pb2+, Cd2+, Cu2+, and Zn2+; 200 µg.g−1soil of Cr6+ and Ni2+ and 75 µg.g−1 soil of Hg2+. These concentrations which caused a reduction in total microbial counts by < 25, ca. 50, and > 90% of the initial population were chosen based on results obtained from preliminary experiments.
A representative soil sample of 20 g was taken at 10-day intervals by mixing equal parts of the three replicates representing a single treatment. Total plate counts were determined on metal-free nutrient agar medium according to [Citation17]. The same medium provided with known concentrations of heavy metal ions was used to determine the periodical changes in the intrinsically resistant microorganisms during the 40-day incubation period.
Impact of heavy metals on some nitrogen transformations processes in soil
Ammonification
The same clay-loam soil used in the previous experiment was used to elucidate the effect of Pb2+, Cd2+, Cu2+, Zn2+, Cr6+, Ni2+, and Hg2+ on the ammonification process. Two-hundred-gram portions of the air-dried soil were distributed in glass jars and mixed with 1% (w/w) peptone (12.6% N). In addition to the control (untreated) soil, the experiment comprised three other treatments prepared by adding 200 µg.g−1 soil (low-metal concentration, T₁), 1000 µg.g−1 soil (moderate-metal concentration, T2) and 2000 µg.g−1 soil (high metal concentration, T3) of the seven heavy metals under investigation. These metal-ions concentrations were achieved by adding certain volumes of the standard stock solutions and sufficient amounts of distilled water to adjust WHC at 75%. Three jars were prepared as triplicates for each treatment, the soil was then thoroughly mixed and incubated at 28°C for 20 days. A representative soil sample of 40 g was taken from each of the three replicates at intervals of 5 days and subjected to the determination of total mineral nitrogen (NH4-N and NO3-N) according to [Citation16].
Nitrification
Using the same soil, a further experiment was carried out to investigate the effect of the seven heavy metals on the nitrification process. The soil was supplemented with 400 µg NH4-N.g−1 soil in the form of (NH4)2SO4 and the experiment proceeded under the same prevailing experimental conditions as described above. At 5-day intervals, a representative soil sample of 40 g was obtained from each three triplicates and the NH4-N and NO3-N were determined individually according to [Citation16].
Results
Effect of soil pollution with heavy metals on the total microbial population
The periodic changes in microbial population given in and illustrated in showed that counts determined on the metal-free agar medium increased in the control soil (C) by about 10-folds compared with the other three soil metal-treatments of the seven heavy metals. After 10 days of incubation, the low-metal treated soil (T1) with Hg2+ recorded the highest reduction percentage in total bacterial counts by 91.4% corresponding to the control soil, while the moderate inhibition in the bacterial counts was 58.1% in the soil treated with Pb2+- and the lowest percentage (32.9%) was observed in soil supplemented with Ni2+. On the other hand, a reduction range in bacterial counts between 48.8 to 71.8% was recorded for the other four heavy metals. A more suppression effect was observed with the other two metal-soil treatments, T2 and T3. Except for Ni2 + where a 48.8% reduction in total bacterial counts in soil was observed, the T2-treatment caused a drastic 92.1 to 99.6% reduction for the other six metals after 10 days. This percentage range increased slightly to 94.6–99.6 in T3-treatment after the same period for the seven tested heavy metals.
Figure 1. Periodical changes in the total bacterial counts (CFU.g−1 air-dried soil) in soil treated with different concentrations of seven heavy metals.
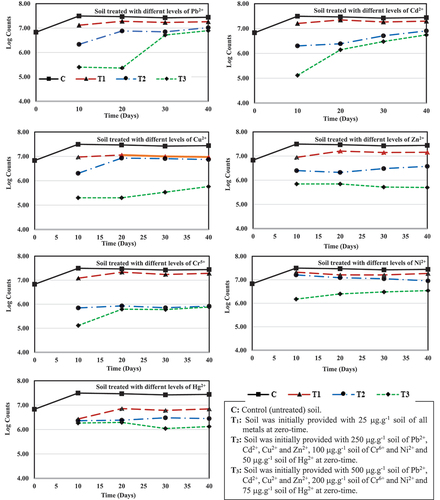
Table 1. Periodical changes in the total bacterial counts (CFU.g−1 air-dried soil) in soil treated with different concentrations of seven heavy metals.
The results indicated that within the three soil metals treatments, the total bacterial counts either remained stable or gradually increased over the 10 days of the experiment. But remained markedly lower than the corresponding counts in the control one. After 40 days of incubation with the three metals treatments, 28.2% in T1- Cd2+, 48.0% in T1- Zn2+, and 74.5% T1- Hg2+ were found to be the lowest, moderate, and highest inhibition percentages, respectively, in reducing the bacterial counts. With the except Cu2+, which caused a percentage reduction in total bacteria counts of 65.8, treatment of the soil with the other three heavy metals resulted in a percentage reduction of between 30.5 and 34.0% compared to the untreated soil.
Providing the soil with different concentrations of heavy metals at the higher level, T2, leads to a greater reduction in the total bacterial count. The highest percentage reduction was observed with soil treated with 100 µgCr6+.g−1 soil with 97.0%, while the least effect was recorded at 250 µgPb2+.g−1 soil with 62.3% compared to the control soil. On the other hand, a percentage reduction ranging from 67.4 to 89.8 was observed in the soils treated with the other five heavy metals at the same concentrations of T2. The strongest suppression effect in the total bacterial counts was recorded after the addition of the metals concentrations at the highest level, T3, where 95.2 to 98.0% of the total bacterial counts were reduced by treating the soils with Hg2+, Cr6+, Cu2+ and Zn2+, while less effect was observed in soil treated with Pb2+, Cd2+, and Ni2+ at the same level of T3 where the reduction in total bacterial counts was 71.7, 79.9 and 87.5% compared to the control soil, respectively.
It is of interest to note that the percentages of inhibition of the total bacterial counts were slightly lower after 40 days than after 10 days but still showed the same pattern. This difference in percentages was translated into the number of bacteria able to tolerate specific concentrations of the respective metal. Concerning this point, it was of importance to test the possible development of metal-tolerant bacteria.
A clear increase in the number of intrinsically resistant bacteria was observed throughout the experiment (). After 40 days in the T1 treatment, they comprised 34.2–71.8% of the initial bacterial counts in the different six metals, except for Hg2+, where they amounted to 25.5%. This range of intrinsically resistant bacterial counts decreased with increasing metal concentration, reaching 26.9–37.7% in T2 treatment, except for Zn2+, Cr6+, and Hg2+ where 13.6, 3.1, and 10.2% of the initial bacterial counts were recorded, respectively. The T3 treatment of the seven tested metals was tolerated by a lower number of bacteria, with 28.3, 20.1 and 12.5% tolerable for Pb2+-, Cd2+- and Ni2+-soil treatments, respectively, while only a few thousands could tolerate the concentrations of this treatment with the other four heavy metals.
Impact of heavy metals on some nitrogen transformation processes in soil
The results of the previous part of this study indicated the negative effects of seven heavy metal salts on soil microorganisms, with the possible development of resistant species that can withstand the toxic effects of these metals. It was of interest to investigate to what extent the toxicity of these metals can influence the biological activity in soil. In this part of the study, the mineralization of nitrogen-rich organic compounds (ammonification) and the subsequent oxidation of the resulting NH4-N (nitrification), were investigated, which could be influenced by the increasing load of heavy metal salts in fertile soils.
Ammonification
Ammonification is the process by which organic N is mineralized to NH4+. It is the first step in organic N decomposition and is often referred to as N mineralization. This process is carried out by microorganisms in the soil that mineralize dissolved low molecular weight, dissolved, organic molecules containing amine or amide groups (with the general formula R-NH2) and produce ammonium (NH4+).
In the present experiment, peptone, as a nitrogen-rich organic matter was added to the same fertile soil under investigation at the rate of 1% (W/W) (12.6% N). Then, in addition to the untreated one, the soil was supplemented with heavy metal salts at the aforementioned three levels of 200, 1000, and 2000 µg.g−1 soil. The changes in total inorganic-N in four consecutive periods successive periods were followed as a reliable indicator of organic nitrogen mineralization in the soil under optimal conditions.
The periodic increase in total inorganic nitrogen was shown in () and recorded in (). It is quite clear that, apart from the type of heavy metal and its concentration applied in the soil, the periodical changes in inorganic nitrogen production followed a more or less constant pattern in all soil treatments with few exceptions.
Figure 2. Periodical changes in the total inorganic-N (µg. g−1 soil) in soil treated with three concentrations of each of the tested seven heavy metals.
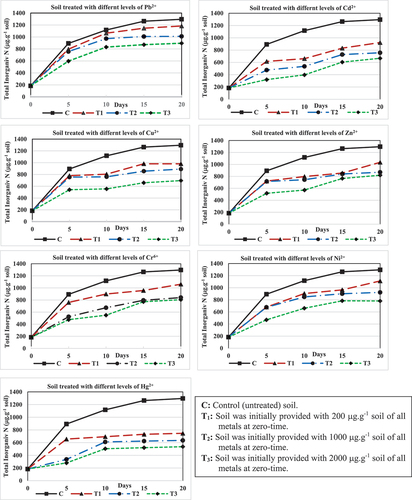
Table (2): Periodical changes in the total inorganic-N (µg.g−1 soil) in soil treated with three concentrations of each of the tested seven heavy metals.
The amounts of mineralized nitrogen reached their maximum in the control soil within the first period (5 days), including all metal treatments, followed by a rather slow increase until the end of the period of 20 days. The total inorganic nitrogen in the control soil (C) increased from 184.0 µg.g−1 soil at 0-time to 893 µg.g−1 soil after 5 days, which means about 35.3% of the organic nitrogen in the soil was transformed to the inorganic form. The application of 200, 1000, or 2000 µg.g−1 of the seven metals (T1, T2, and T3) resulted in a marked reduction in the mineralization rate where the increase in the amount of inorganic nitrogen within this period (5 days) ranging from 432 to 613, 152 to 573 and 96 to 413 µg.g−1 soil for treatments T1, T2 and T3, respectively. The corresponding amounts of mineralized nitrogen represented 21.5–30.5, 7.6–28.5, and 4.8–20.1% of the initial organic nitrogen content. On the other hand, it was noticeable that the greatest reduction in nitrogen mineralization was observed after 5 days with Hg2+ at the three levels (T1, T2, and T3), which recorded 23.5, 7.6, and 4.8% of the initial organic-N. Hg2+ was followed, in its inhibitory effect, by Cd2+ except at T1, as the percentages of mineralized nitrogen in the three soil treatments were 21.5, 14.5, and 6.8 of the initial organic-N, respectively.
As recorded in (), mineralization increased in all treatments with metals at the beginning of the experiment, then decreased significantly after 5 days, and then increased slightly until the end of the experiment. After 20 days, 28.0–49.8, 22.4–41.1, and 17.5–35.4% of the initial organic nitrogen were mineralized in T1, T2, and T3, respectively in the different metal-treated soils against 55.3% in the untreated soil. Moreover, Hg2+ recorded the lowest percentages of mineralized nitrogen after 20 days, namely 28.0, 22.4, and 17.5 in the three levels (T1, T₂, and T3) of initial organic nitrogen.
Moreover, the maximum values of inorganic nitrogen production were recorded in 0–5-day intervals of 709 µg.g−1 soil in control soil and in the other three metals treatments. In contrast with the untreated soil, the amounts of inorganic-N decreased and ranged from 613.0 to 432.0 µg.g−1 soil in T1, 573.0 to 152.0 µg.g−1 soil in T2 and 413.0 to 96.0 µg.g−1 soil in T3. On the other hand, and because the mineralization rate decreased until the end of the experiment, the lowest values of inorganic-N produced were observed in the 15–20 day interval.
Nitrification
The present experiment was conducted to study the effect of the investigated heavy metals on the biological oxidation of ammonium salts in the same fertile soil under optimal conditions of aeration (75% WHC) and temperature of (28°C). The end product of NH4-N oxidation yields NO3-N, the most appropriate form of inorganic nitrogen for plants. The fertile clay-loam soil was amended with 400 mg NH4-N.g−1 soil in the form of (NH4)2SO4 as the sole energy source for the nitrifying bacteria as a group, in addition to the elevated levels of the seven heavy metals studied.
It is worth mentioning that the assessment of nitrification can be achieved either by the determination of NH4-N or by the increase in NO3-N on condition that no other biological processes that cause any quantitative changes in soil inorganic nitrogen may occur. Actually, under the prevailing experimental conditions, immobilization of inorganic nitrogen and denitrification are not expected to take place where suitable oxidation is provided and at the same time, no poor-N organic materials are present in soil. At any rate, and apart from an experimental error that may take place equally in the different soil treatments, the comparison between treatments in terms of the amounts of NO3-N produced was considered a reliable parameter for nitrification which could be influenced by the addition of heavy metals in soil.
() and show the periodical increase in NO3-N throughout the successive periods. Nitrification started in all soil treatments from the early beginning and continued, albeit at relatively lower rates, until the end of the experiment. The results recorded a progressive increase in NO3-N production, with the highest net nitrified nitrogen occurred within the first 5 days (0–5 days) in some treatments, while in other treatments, it occurred at the 5–10, 10–15 and 15–20 day intervals. It was found that, in untreated soil (C) nitrification led to the production of 145 µg NO3-N.g−1 soil within the initial five days, indicating that approximately 29% of the soil’s Ammoniacal nitrogen was oxidized during this short period. Subsequently, the oxidation process continued at a comparatively slower pace, resulting in the oxidation of about two-thirds of NH+4-N in soil by the end of the 20-day period, corresponding to the production of 337 µg NO3-N.g−1 soil.
Figure 3. Periodical changes in the total nitrate-N (µg.g−1 soil) in soil treated with three concentrations of each of the tested seven heavy metals.
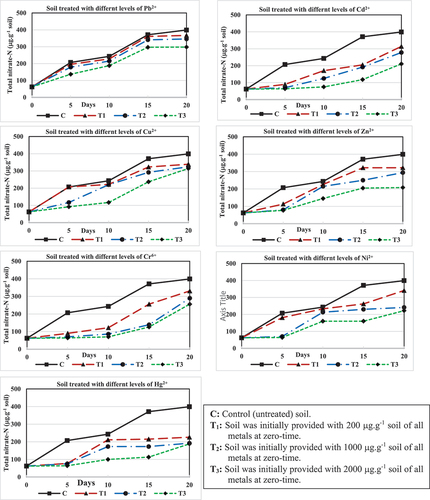
Table (3): Periodical changes in the total nitrate-N (µg. g−1 soil) in soil treated with three concentrations of each of the tested seven heavy metals.
Providing the soil with any of the test heavy metals resulted in a slowdown in the biological oxidation of NH4-N. Within the 5-day period, the T1 soil treatment showed a reduction in the biological oxidation of initial NH+4-N content, ranging from 10.2 to 29.0% in Pb2+, Cu2+, Zn2+, and Ni2+, except for Cd2+, Cr6+, and Hg2+ which exhibited a more inhibitory effect (5.6, 5.6 and 3.0% of the initial NH4-N oxidation, respectively). The low concentration (200 µg.g−1 soil, T1) of any test metal had a relatively minor negative effect on nitrification, with NO3-N production after 20 days ranging from 252 to 305 µg.g−1 soil, representing 50.4% to 61.0% oxidation of the initial NH4-N content. Comparatively, it was clear that mercury proved to be more toxic than other metals under investigation, as the addition of 200 µg Hg2+.g−1 soil reduced nitrification output to 164 µg NO3-N.g−1 soil, equivalent to 33% oxidation of the initially found NH4-N in soil.
As might be expected, an increase in the concentration of any test heavy metal in the soil led to a corresponding decrease in the rate of ammonium oxidation. Results showed that the presence of 1000 µg.g−1 soil (T2) was more toxic to the nitrification process than the lower concentration (T1). Among the metals, Hg2+ followed by Ni2+ and Cd2+ exhibited the most suppressive effects resulting in the production of 130, 179, and 216 µg NO3-N.g−1 soil, respectively throughout the 20-day experimental period, representing 26.0, 35.8 and 43.2% of the initial NH4-N. On the other hand, the other four metals, Pb2+, Cu2+, Zn2+, and Cr6+ were comparatively less toxic, with a range of 45.6 to 56.7% of the original NH4-N transformed to NO3-N.
More retardation in the biological oxidation of NH4-N was observed when the soil was supplemented with 2000 µg.g−1 soil (T3). Results showed that Pb2+ and Cu2+ at this concentration were less toxic where 47.2 and 50.4% of the initial NH4-N were oxidized after 20 days, respectively, compared to 67.4% in the control soil (C). The more toxic effect was given by Ni2+ and Cr6+ at the same concentration (T3), with a total NO3-N found at the end of the experiment reaching 160 and 194 µg.g-1 soil, respectively, equivalent to 32.0 and 38.8% of the NH4-N oxidized. Meanwhile, the highest suppressive effect on nitrification, at this concentration (T3) was observed in soil treated with Hg2+, Zn2+, and Cd2+ where 125, 146, and 149 µg NO3-N. g−1soil were produced, representing 25.1, 29.2, and 29.8% oxidation of the initial soil NH4-N, respectively.
Discussion
Effect of soil pollution with heavy metals on the total microbial population
Soil contamination by heavy metals is a prevalent environmental concern, primarily stemming from human activities like mining, smelting, and agriculture [Citation10]. As a result, the buildup and harmful impacts of heavy metals at elevated levels can lead to a deterioration in soil quality, soil microorganisms, and a decrease in soil fertility.
The findings of the current research indicate a substantial decline in the overall bacterial populations across the three soil treatments (T1, T2, and T3). As the concentrations of metals increased, the reduction in bacterial populations also escalated, with a reduction of 71.8% in the initial bacterial counts in the T1-treatment, and a range of 92.1% to 99.6% in the 92.1–99.6% in T2- and T3-treatments after 10 days. A minor decrease in the total bacterial count was noted after the experiment. This decline underscores the detrimental influence of heavy metals on the microbial community [Citation2] attributed the harmful effect of heavy metals on microorganisms to their bioavailability highlighting a strong binding affinity between heavy metals and microorganisms. Furthermore, he suggested that as the concentration of heavy metals rises, the activity of numerous enzymes experiences a significant decrease, with this decline potentially being a direct result of the interaction between the enzyme and the heavy metals, leading to a direct impact on the spatial structure of the enzyme’s active groups and ultimately causing its degradation. Consequently, the growth and reproduction of microorganisms are inhibited. Another explanation was introduced by [Citation7,Citation8,Citation14]who suggested that the toxicity of heavy metals, such as lead, occurs due to its interaction with sulfhydryl groups, leading to the destruction of nucleic acids and proteins. This interaction also inhibits microbial respiration, enzyme activities, transcription processes, and disrupts cell membranes. Heavy metals like lead enter bacterial cells through pathways used for essential divalent metals like Mn2+ and Zn2+, causing toxic effects by altering the osmotic balance within the bacterial cells [Citation18].
Considering the concentrations of metals introduced into the soil in the study, the toxicity toward soil microorganisms can be ranked as follows: Hg2+ ≥Cr2+> Ni2+ > Cu2+ ≥Zn2+ > Cd2+> Pb2+. The present study reported that mercury and, to some extent, chromium showed the highest reduction percentage in total bacterial counts compared with the other five metals. Treatments with Hg+2 recorded reductions of 91.4, 92.7, and 94.6% reduction in total bacterial counts in the T1-,T2-, and T3- treatment, respectively, after 10 days. On the other hand, the Cr6+ treatment showed a reduction of 61.4, 97.8, and 97.7% in the same corresponding treatments. Concerning this point [Citation19] mentioned that mercury ions induce toxic effects through protein precipitation, enzyme inhibition, and corrosive actions. Mercury binds not only to sulfhydryl groups but also to phosphoryl, carboxyl, amide, and amine groups. Proteins, including enzymes, containing such groups are highly susceptible to reactions with mercury, leading to their inactivation upon binding.
The study was extended to investigate the possible development of metal-tolerant bacteria throughout the relatively short period of metal-soil contact (40 days). The results obtained from the experiment after 40 days claimed that the presence of heavy metal salts in soil may encourage the development of metal-tolerant microorganisms which are adapted to the toxic effect of heavy metal pollution. The highest percentage of metal-tolerant bacteria was recorded in the T1-treatment of all tested metals and ranged from 25.5 to 71.8% of the total initial bacterial count. However, as the concentration of metals increased (T2 and T3), the metal-tolerant bacteria percentages decreased. This finding aligns with the study of [Citation20,Citation21] who suggested that the development of heavy metal-resistant microorganisms can take place in a relatively short time. On the other hand [Citation2] reported that heavy metal contamination can produce different microbial community patterns. Despite significant changes in the chemical and biological properties of the soil, the original microorganisms in the soil’s microbial community persist. In the case of long-term heavy metal-contaminated soil, the selection process favors microorganisms that possess specific adaptations to thrive in polluted soil.
Impact of heavy metals on some nitrogen transformation processes in soil
Ammonification
According to the saying of [Citation10] soil nitrogen and heavy metals are two distinct components that can interact and influence each other in various ways. The relationship between soil nitrogen content, form, and heavy metals is indeed complex and influenced by various factors, including soil characteristics, environmental conditions, and management practices.
The results obtained in this experiment demonstrated the suppressive effects of the tested seven heavy metals on the biological activity of ammonification. A quite marked differences between treatments were observed which could be attributed to the toxic nature of the heavy metal salt and its concentration. [Citation14] explained the reduction in the ammonification process by stating that the bacterial community is highly sensitive to heavy metals, leading to a decrease in N transformation in soil. Ammonifying bacteria, which are crucial for nitrogen cycling, were found to be more abundant in uncontaminated soil compared to soil treated with Zn2+, Cd2+, and Cu2+, treatments. The presence of heavy metals in the soil also inhibits the growth of Azotobacter, which plays a key role in the nitrogen cycle and subsequently hinders nitrogen mineralization.
The comparison of the seven test heavy metals in terms of their impact on organic nitrogen mineralization revealed that Pb2+ exhibited the lowest suppressive effect. After 5 days at the three different levels (T1, T2, and T3) of the initial organic-N, nitrogen mineralization percentages were recorded as 30.5, 28.5, and 20.1, respectively. However, these percentages increased after 20 days to reach 49.8, 41.1, and 35.4 of the initial organic-N for the same treatments. This result appears to be similar to the findings of a previous investigation of [Citation21] who suggested that Pb2+ has a limited toxic effect due to its rapid binding to the soil matrix, resulting in minimal impact on microbial activity. In contrast, Hg2+ proved to be the highest toxicity among the metals in the mineralization process. The remaining five metals exhibited a moderate effect on nitrogen mineralization, with Pb+2 as the least suppressive ion and Hg2+ as the most suppressive one.
Furthermore, Hg2+ displayed the lowest percentages of mineralized nitrogen after 20 days, measuring 28.0, 22.4, and 17.5 at the three levels (T1, T2, and T3) of the initial organic-N. This observation can be attributed to the process of ammonification, which is carried out by a group of bacteria known as ammonifiers, that is considered very sensitive to the toxicity of heavy metals. This result is consistent with the findings of [Citation19] who proposed that the toxicity of mercury stems from its impact on protein precipitation, enzyme inhibition, and binding to various functional groups such as sulfhydryl, phosphoryl, carboxyl, amide, and amine, rendering the proteins inactive.
Nitrification
As reported by [Citation22] nitrification is the process in which NH4+ is oxidized to nitrite (NO2−) by Nitrosomonas and further to nitrate (NO3−) by Nitrobacter bacteria. Other organisms involved are heterotrophic bacteria e.g. Arthrobacter globiformis, and various Pseudomonas spp., fungi (Aspergillus flavus), and autotrophic bacteria e.g. Nitrosococcus, Nitrosospira, and Nitrosovibrio. They added that soil nitrification was often used as an index to evaluate the toxicity of pollutants to microorganisms in virtue of its sensibility to the change of environmental factors, especially to heavy metals.
The results of the study indicated that an increase in the concentration of any tested heavy metal in the soil led to a corresponding decrease in the rate of ammonium oxidation. The most suppressive effect on nitrification was observed in soil treated with the highest concentration of Hg2+, Zn2+, and Cd2+, where after 20 days of incubation, only 25.2, 29.2 and 29.8% of the ammonium-N were oxidized, respectively. This finding was consistent with the findings of [Citation15,Citation23,Citation24] who also reported a negative effect of Cd2+ on nitrogen transformation. Regarding Zinc, it was found that 100 ppm of Zn2+ in soils can hinder nitrification processes, while approximately 1000 ppm inhibits the majority of microbiological processes in soils. However, the concentration of < 10 ppm Hg2+ was found to have the highest toxic effects on nitrifiers in soils. On the other hand, the results showed that Cu2+, Pb2+, Cr6+, and Ni2+ had the least toxicity, with 50.4, 47.2, 38.3, and 32.0% of the ammonium-N being oxidized, respectively. The trend of metal toxicity obtained in this experiment agrees or may slightly differ from results reported by [Citation24] and any slight difference here could be attributed to variation in soil characteristics in addition to possible variation in the environmental condition prevailing.
In another study by [Citation25,Citation26] they analyzed the response difference of soil potential nitrification rate to Cu2+ stress. The findings revealed that the addition of 1000 µg. g−1 soil of Cu2+ caused a significant reduction in soil nitrification by 40.0%. These results agreed with the obtained results in the present experiment, where the addition of 1000 µg Cu2+.g−1 soil led to a 47.9% reduction in the nitrification biological process after 20 days. This reduction was attributed to the inhibition of growth in ammonia-oxidizing microorganisms, as the heavy metals were found to hinder the amoA functional genes of transcription, replication, and growth of these groups of microorganisms. This gene (amoA) was often chosen as a molecular marker of ammonia-oxidizing microbes to investigate ammonia-oxidizing communities shift in polluted soils. This explanation also was confirmed by [Citation27]; who reported an obvious decrease in soil potential nitrification rate due to the inhibition of amoA functional genes in the ammonia-oxidizing microbes.
As the present study showed that both Cu2+ and Cr6+ soil treatments at the highest level (T3) caused an inhibition in the nitrification by 70.2 and 61.2%, respectively [Citation14], stated that the decrease of mineralization rates as a consequence of Cu2+ pollution might reduce the turnover of organic matter and availability of nutrients in the ecosystem due to the inhibition of a specific group of microorganisms as nitrifying bacteria. Consequently, the nitrification rate appears to serve as a valuable biomarker for soil monitoring of both metals Cu2+ and Cr6+ and other heavy metals.
Conclusion
Summing up the results obtained in the present study, it can be concluded that:
Concerning the effect of different concentrations of heavy metals on the total bacterial counts, after 10 days, Hg2+ caused the highest reduction in T1-treated soil (91.4%), followed by Pb2+ (58.1%) and Ni2+ (32.9%), meanwhile, in T2 treatments, was ranged from 92.1% to 99.6% and increasing to 94.6% to 99.6% in T3. After 40 days, inhibition was at maximum by 74.5% of Hg2+ in T1 and reached 97.0% by Cr6+ in T2 and from 95.2% to 98.0% by Hg2+, Cr6+, Cu2+, and Zn2+ in T3.
The intrinsically resistant bacteria comprised only 25.5% for Hg2+ and 34.2-71.8% in the different six metals after 40 days in the T1 treatment. In T2 treatment, their percentage reached 13.6, 3.1, and 10.2%, for Zn2+, Cr6+, and Hg2+, respectively. On the other hand, 28.3, 20.1 and 12.5% were tolerable for Pb2+-, Cd2+- and Ni2+-soil in T3 treatments, respectively.
About 35.3% of the organic nitrogen in the soil was transformed to the inorganic form after 5 days in the untreated soil. The application of the tree treatments, T1, T2, and T3, resulted in a marked reduction in the mineralization rate of 21.5-30.5, 7.6-28.5, and 4.8-20.1%, respectively. The most nitrogen mineralization reduction percentages were observed after 5 days by Hg2+ at T1, T₂, and T3 of 23.5, 7.6, and 4.8%, respectively.
After 20 days, also, Hg2+ recorded the least percentages in the amount of mineralized nitrogen of 28.0, 22.4, and 17.5 at the three levels (T1, T₂, and T3) of the initial organic-N.
At the period of 5 days, the T1 soil treatment with Cd2+, Cr6+ and Hg2+ resulted in retardation in the biological oxidation of initial NH+4-N content by 5.6, 5.6 and 3.0%, respectively. After 20 days, also, mercury proved to be more toxic than other metals under investigation, where 200 µg Hg2+.g−1 soil resulted in a 33% reduction in the output of nitrification. Presence of 1000 µg. g−1 soil (T2) was more toxic to the nitrification process where, Hg2+ followed by Ni2+ and Cd2+ caused suppressive reduction by 26.0, 35.8 and 43.2%, respectively. On the other hand, the highest suppressive effect on nitrification, at (T3) was observed in soil treated with Hg2+, Zn2+, and Cd2+ of 25.1, 29.2, and 29.8% of initial soil NH4-N were oxidized, respectively.
The results obtained in this study clearly showed that the microbial community was affected, at different levels, by the heavy metals contamination. This led to a change in their number, activity, structure, and function which gives a chance to use these parameters as indicators of soil pollution with heavy metals by monitoring these microbial communities and their biochemical functions in polluted soils to provide valuable insights for sustainable soil management and remediation. More studies in this research field are needed in the future.
Disclosure statement
No potential conflict of interest was reported by the author(s).
References
- Chaudhery MH, Rüstem K, editors. Environmental pollution and environmental analysis). In: Modern environmental analysis techniques for pollutants. Vol. Chapter 1. Elsevier Inc.; 2020. p. 1–36. doi: 10.1016/C2018-0-01639-4
- Dian C. Effects of heavy metals on soil microbial community. IOP Conf Ser: Earth Environ Sci. 2018;113. doi: 10.1088/1755-1315/113/1/012009 113
- Golia EE. The impact of heavy metal contamination on soil quality and plant nutrition. Sustainable management of moderate contaminated agricultural and urban soils, using low-cost materials and promoting circular economy. Sustainable Chem Pharm. 2023;33:33. doi: 10.1016/j.scp.2023.101046
- Lian C, Shenglu Z, Yaxing S, et al. Heavy metals in food crops, soil, and water in the Lihe River Watershed of the Taihu Region and their potential health risks when ingested. Sci Total Environ. 2018;615(15):141–149. doi: 10.1016/j.scitotenv.2017.09.230
- Wendan X, Xuezhu Y, Qi Z. Evaluation of cadmium transfer from soil to leafy vegetables: influencing factors, transfer models, and indication of soil threshold contents. Ecotoxicol Environ Saf. 2018;164(30):355–362. doi: 10.1016/j.ecoenv.2018.08.041
- Briffa J, Sinagra E, Blundell R. Heavy metal pollution in the environment and their toxicological effects on humans. Heliyon. 2020;6(9):e04691. doi: 10.1016/j.heliyon.2020.e04691
- Igiri B, Okoduwa S, Idoko G, et al. Toxicity and bioremediation of heavy metals contaminated ecosystem from tannery wastewater: a review. J Toxicol. 2018;2018:1–16. doi: 10.1155/2018/2568038
- Tarekegan M, Salilih F, Ishetu A, et al. Microbes used as a tool for bioremediation of heavy metal from the environment. Cogent Food Agric. 2020;6(1):1783174. doi: 10.1080/23311932.2020.1783174
- Ayele A, Haile S, Digafe A, et al. Comparative utilization of dead and live fungal biomass for the removal of heavy metal: a concise review. Sci World J. 2021;1–10. doi: 10.1155/2021/5588111
- Zhang L, Kangning X, Panteng W. Effects of heavy metals on nitrogen in soils of different ecosystems in the Karst Desertification of South China. Forests. 2023;14(7):1497. doi: 10.3390/f14071497
- Wan P, Xiong K, Zhang L. Heterogeneity of spatial-temporal distribution of nitrogen in the Karst Rocky desertification soils and its implications for ecosystem service support of the desertification control—a literature review. Sustainability. 2022;14(10):6327. doi: 10.3390/su14106327
- Zhang L, Xiong K, Wang X. Study of soil nitrogen in karst rocky desertification areas: a literature review. Pol J Environ Stud. 2022;31(6):5533–5547. doi: 10.15244/pjoes/152450
- Jach ME, Sajnaga E, Ziaja M. Utilization of legume-nodule bacterial symbiosis in phytoremediation of heavy metal contaminated soils. Biology (Basel). 2022;11(5):676. doi: 10.3390/biology11050676
- Hamsa N, Yogesh GS, Usha K, et al. Nitrogen transformation in soil: effect of heavy metals. Int J Curr Microbiol App Sci. 2017;6(5):816–832. doi: 10.20546/ijcmas.2017.605.092
- Kamrun N, Md MA, Azmerry K, et al. Levels of heavy metal concentrations and their effect on net nitrification rates and nitrifying archaea/bacteria in paddy soils of Bangladesh. Appl Soil Ecol. 2020;156:103697. doi: 10.1016/j.apsoil.2020.103697
- Rowell L. Soil science: “methods and application”. Vol. 605. Third Avenue (NY): John Welly & Sons, Inc.; 1994. p. 10158.
- Allen ON. Experiments in soil bacteriology. Soil Sci. 1959;85(3):60. Burgess Publishing Co. Minneapolis 15 Minnesota.
- Fashola MO, Ngole JVM, Babalola OO. Heavy metal pollution from gold mines: Environmental effects and bacterial strategies for resistance. Int J Environ Res Public Health. 2016;13(11):1047. doi: 10.3390/ijerph13111047
- Yuan-Seng W, Ahmed IO, Mohamed H, et al. The toxicity of mercury and its chemical compounds: molecular mechanisms and environmental and human health implications: a comprehensive review. ACS Omega. 2024;9(5):5100–5126. doi: 10.1021/acsomega.3c07047
- Muhammad S, Naila A, Saraj B, et al. Variation and succession of microbial communities under the conditions of persistent heavy metal and their survival mechanism. Microbial Pathogenesis. 2021;150:104713. doi: 10.1016/j.micpath.2020.104713
- Abel-Inobeme. Effect of heavy metals on activities of soil microorganism. In: Charles OA, Deepak GP and Yogeshvari KJ, editors. Microbial rejuvenation of polluted environment. Vol. 3. Springer link; 2021. p. 115–142. doi: 10.1007/978-981-15-7459-7_6
- Liu A, Fang D, Wang C. Primary research on the recovery of soil nitrification and its key factors under the Cu stress. Ecol Environ Sci. 2014;23:1986–1990.
- Afzal M, Yu M, Tang C, et al. The negative impact of cadmium on nitrogen transformation processes in a paddy soil is greater under non-flooding than flooding conditions. Environ Int. 2019;129:451–460. doi: 10.1016/j.envint.2019.05.058
- Anna LB, Piotr B. The effect of industrial heavy metal pollution on microbial abundance and diversity in soils —a review. IntechOpen; 2019. Chapter 26.
- Chen C, Tan K, Min W, et al. Long-term metal pollution shifts microbial functional profiles of nitrification and denitrification in agricultural soils. Sci Total Environ. 2022;830(15):154732. doi: 10.1016/j.scitotenv.2022.154732
- Qiang L, Mingzhu L, Yuanpeng D, et al. Impacts of Cu and sulfadiazine on soil potential nitrification and diversity of ammonia-oxidizing archaea and bacteria. Environ Pollut Bioavailability. 2019;31(1):60–69. doi: 10.1080/26395940.2018.1564629
- Kapoor V, Li X, Elk M. Impact of heavy metals on transcriptional and physiological activity of nitrifying bacteria. Environ Sci Technol. 2015;49(22):13454. doi: 10.1021/acs.est.5b02748