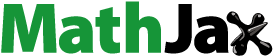
Abstract
Low cost water purification methods are needed in developing countries. Therefore, we have examined the biosorption potential of apple peel immobilised on sodium alginate beads. This was performed using a solution containing seven toxic ions, namely arsenic, cadmium, chromium, copper, mercury, lead and nickel. The effects of pH, contact time, biosorbent concentration and the presence of co-ions was investigated. Results showed that biosorption of all the ions except As and Cr was pH-dependent. At neutral pH the biosorption order for apple peel beads was Cd > Cu > Pb > Ni > Hg > Cr > As with 90, 80, 73, 72, 70, 10 and 2% biosorption, respectively. Biosorption reached equilibrium for Pb (6 h), Hg (10 h), Cd and Ni (24 h), and Cu (48 h). Kinetic models for film diffusion, pore diffusion, pseudo-first order (PFO), pseudo-second order and Elovich equation were applied and PFO was found to be the best fitting model based on coefficient of determination values. The biosorption of ions by apple peel beads was significantly higher than with empty beads throughout the time course (15 min—72 h). Biosorption increased with increasing biosorbent concentration for all ions except As, Cr and Hg. A significant suppression in biosorption for all ions was observed in the presence of co-ions, except for Cd and Cu. In conclusion, apple peel beads have the ability to remove toxic ions from a cocktail solution that has properties that mimic drinking water.
PUBLIC INTEREST STATEMENT
The present study was undertaken with the aim to investigate the biosorption of apple peel immobilised as sodium alginate beads. The aim was to develop an economical but realistic drinking water decontamination method for use in developing countries where heavy metal contamination of drinking water is problematic. Thus, the capacity of the biosorbent beads to remove seven different ions in simulated drinking was examined. The simulation included realistic contamination levels and a neutral pH range as both would be characteristics of drinking water. This ensured that the results with the apple peel beads would be specific and relevant to drinking water.
1. Introduction
Heavy metals are a serious health concern because they are non-biodegradable, persistent and have a very long biological half-life (Barbier, Jacquillet, Tauc, Cougnon, & Poujeol, Citation2005). They can accumulate in the body and are associated with cancer risk and the development of other diseases (Mudhoo, Garg, & Wang, Citation2012; Tchounwou, Yedjou, Patlolla, & Sutton, Citation2012). Conventional methods for removing heavy metals from water are expensive and also generate large quantities of toxic chemical sludge (Acharya, Kumar, & Rafi, Citation2018; Azimi, Azari, Rezakazemi, & Ansarpour, Citation2017). Therefore, polysaccharide based agricultural wastes containing carboxyl, hydroxyl, phenols, amines and other functional groups have been explored as potential low-cost biosorbents for removing heavy metals from solution (Khatoon & Rai, Citation2016; Mudhoo et al., Citation2012).
The consumption of food has been increasing with the growing world population and the disposal of fruit peel as a solid waste is also a growing concern. (Cheok et al., Citation2018; Pathak, Mandavgane, & Kulkarni, Citation2017). Land pollution from dumping solid wastes into landfills as well as the production of methane from anaerobic decomposition has stimulated the interest in converting agricultural and industrial waste material into commercial products (Chand & Pakade, Citation2013; Kandari & Gupta, Citation2017). One such product is apple waste, as it is generated in large quantities in food processing industries and it has also been used to prepare biosorbents for removal of toxic metals from water (Chand, Bafana, & Pakade, Citation2015a; Enniya, Rghioui, & Jourani, Citation2018). Common methods of processing biosorbents includes drying (Mallampati & Valiyaveettil, Citation2013), reducing particle size (Chand & Pakade, Citation2015b; Lee, Jung, Chung, Lee, & Yang, Citation1998; Lee & Yang, Citation1997; Maranon & Sastre, Citation1992a) and preparing activated carbon from apple peel/pomace (Enniya et al., Citation2018). This results in the production of fine biosorbent particles that remain suspended in water and are difficult to separate and thus immobilising them as easily separable beads is recommended (Jakóbik-Kolon, Bok-Badura, Karoń, Mitko, & Milewski, Citation2017).
Sodium alginate is a natural polysaccharide derived from brown algae (Zhao, Wang, Zhang, Gu, & Gao, Citation2018). This polymer has biosorption properties of its own because of the presence of functional groups on its surface (Jawad, Sha, Siajam, Ismail, & Siajam, Citation2016). It forms a gel in water and a very stable polymeric complex in the presence of Ca+2 ions (Mousa et al., Citation2016; Singh, Pavankumar, Lakshmanan, & Rajarao, Citation2012; Vandenbossche, Jimenez, Casetta, & Traisnel, Citation2015). Therefore, very fine biosorbent particles can be mixed with sodium alginate in water and sturdy beads can be produced instantaneously in calcium chloride solution (Lai, Annadurai, Huang, & Lee, Citation2008). The resulting beads are very easy to remove by simple decantation or filtration and hence are very convenient.
In the present work, apple peel was immobilised with sodium alginate and its biosorbent performance was compared to an empty sodium alginate bead. Most of the previously published work has examined single metals in solutions for biosorption studies but recently attention has shifted to multi-ion biosorption (Deng et al., Citation2017; Lessa, Medina, Ribeiro, & Fajardo, Citation2017; Massimi et al., Citation2018; Milani, Consonni, Labuto, & Carrilho, Citation2018; Neris, Luzardo, Da Silva, & Velasco, Citation2018; Paul, Nwoken, & Anumonye, Citation2018; Petrella et al., Citation2018). In the present work, biosorption was studied in a cocktail of arsenic (As V) and six heavy metal ions, namely, cadmium (Cd II), chromium (Cr VI), copper (Cu II), mercury (Hg II), lead (Pb II) and nickel (Ni II), as all are potential drinking water contaminates. Lastly, the metal concentrations previously studied were very high relative to those found in drinking water and were more relevant for industrial waste water (Febrianto et al., Citation2009; Petrovič & Simonič, Citation2016; Yasim, Ismail, Zaki, & Azis, Citation2016). Very few studies have been conducted with low heavy metal contamination levels (Irem, Islam, Khan, Ul Haq, & Hashmat, Citation2017). Additionally, no previous work has examined the use of unmodified apple peel immobilised onto alginate beads for biosorption of seven toxic ions in a low concentration cocktail solution at drinking water pH. Therefore, this study aimed to determine the efficacy of these beads under these conditions. Kinetic modelling of the biosorbent data was also performed in order to elucidate the biosorption process.
2. Materials and methods
2.1. Preparation of metal solutions
Deionised water was spiked with standard solutions of seven ions, namely, As (V), Cd (II), Cr (VI), Cu (II), Hg (II), Pb (II) and Ni (II) at the same concentration (i.e., 0.1 mgL−1). Single ion solutions and cocktail solutions were prepared in the same manner. The pH of the solution was adjusted using 0.1 M nitric acid and 0.1 M sodium hydroxide.
2.2. Biosorbent preparation
Red Eve apples were purchased from a supermarket in Dunedin, New Zealand. The peels were removed, washed thoroughly, once with hot water to remove any wax content, twice with tap water and twice with deionised distilled water, and allowed to air-dry at room temperature for 24 h. They were then cut into small pieces and oven dried at 50°C for 24 h. The dried peel pieces were milled and sieved to a particle size of <240 µm. Apple peel particles were then mixed with 1% sodium alginate slurry in the ratio 6:1 and added dropwise to 0.1M calcium chloride solution. Sodium alginate control beads were prepared similarly without apple peel. Beads formed instantaneously and were allowed to cure in the solution at 4°C for 24 h. They were washed thoroughly and allowed to dry to a constant weight at 37°C for 48 h. The beads were stored in airtight containers away from moisture until further use. After the biosorption process, beads were removed from the solution by decantation and dried again to a constant weight at 37°C for 48 h.
2.3. Surface imaging
The surface morphology of the apple peel particles and the beads before and after biosorption was examined using SEM-EDS. The samples were mounted on an aluminium stub using double sided carbon tape and sputter coated with 10 nm of gold palladium using an Emitech K575x sputter coater (EM Technologies Ltd, Kent, England). The surface morphology was examined using a JEOL 6700F Field emission SEM (JSM-6700F, JEOL Ltd., Japan) at 3.0 kV. Samples for EDS were coated with 10 nm of carbon using an Emitech 250X carbon coater attachment on the sputter coater. EDS analysis was performed using a JEOL 2300F EDS system (JEOL Ltd, Tokyo, Japan) at 20.0 kV.
2.4. Biosorption experiments
To study the biosorption process, cocktail solutions of seven ions were shaken with the beads. The difference in the concentrations measured before and after biosorption was used for calculating various parameters. One important assumption was that the decrease in the concentration of ions from the solution was equal to the uptake of ions by the biosorbent (Kratochvil & Volesky, Citation1998). The following equations were used:
where, co and ct (mg L−1) are the initial concentration and concentration at time “t” respectively, cad (mg L−1) is the concentration biosorbed, qt (mg g−1) is the amount of ions biosorbed per gram of bead, V (mL) is the volume of solution and m (g) is the weight of the biosorbent. The time point beyond which there was no significant increase in biosorption was defined as the equilibrium time, (qt) while qe was the experimentally observed equilibrium biosorption capacity.
An aliquot of cocktail solution with no bead was used as a quality control sample, to rule out the possibility of any ion adsorption by the walls of the flasks. Similarly, deionised water with beads was used to detect any background levels of the ions of interest leaching from the beads into the solution. Both of these controls were included in each experiment.
2.5. Metal detection by ICP-MS
At the end of the biosorption process, the solutions were decanted into Digitubes (50 mL ICP MS grade polypropylene and certified with no metallic additives). They were then acidified with 2% nitric acid and stored at 4°C overnight. An Agilent 7900 quadrupole inductively coupled plasma detector coupled with mass spectrometry (ICP-MS) was used to measure the concentration of the metals. The sensitivity of the instrument required dilution of the samples with 2% nitric acid prior to injection. A general purpose tune was selected for robust conditions to minimise interferences and instrumental drift. A cocktail of six reference elements was added online to compensate for any drift or possible matrix effects. The instrument was calibrated using NIST traceable ICP-MS standards.
2.6. Effect of ph
To investigate the effect of pH on biosorption, 25 mL aliquots of cocktail solutions containing 0.1 mg L−1 of each ion were added to glass flasks and the pH of each flask was adjusted to either 6.5, 7.0, 7.5. 8.0 or 8.5. Each flask was shaken in an orbital mixer incubator at 25°C and 250 rpm with one apple peel bead for 24 h. The concentration of solutions was then measured by ICP-MS.
2.7. Effect of contact time
To study the effect of contact time, duplicate glass flasks containing cocktail solution (25 ml) at 0.1 mg L−1 concentration of each metal ion and pH 7.0 were incubated with one apple peel bead or with one sodium alginate bead. Flasks were kept at 25°C and were shaken at 250 rpm. Duplicate flasks were removed at various time points (0.25–72 h) and the concentration of the metals remaining in the solution was measured.
2.8. Kinetic models
All kinetic models were analysed by linear regression using the same set of biosorption data The values for the constants kFD, kPD, k1, k2, a and b were calculated from the slopes and y-intercepts of the respective straight line graphs (Table ). Goodness of fit for each model was demonstrated by the closeness of R2 values to unity, which represents the percentage of variability in the dependent variable (Foo & Hameed, Citation2010), and data is presented for R2 ≥ 0.93. The fitting of the experimental data to any of the models suggests that the process can be explained by the assumptions proposed by that particular model (Mousa et al., Citation2016).
Table 1. Kinetic models used
2.9. Effect of biosorbent concentration
To investigate the effect of biosorbent concentration, 25 mL aliquots of cocktail solutions containing 0.1 mg L−1 of each ion were added to glass flasks and the pH was adjusted to 7.0. An increasing number of apple peel beads (1–10) were added to individual flasks which were then shaken in an orbital mixer incubator at 25°C and 250 rpm for 10 h. The concentration of solutions was then measured by ICP-MS.
2.10. Effect of co-ions
To examine the effect of the presence of co-ions, 25 mL aliquots of the seven single ions in solution each at an initial concentration 0.1 mg L−1 were added to individual glass flasks and these were compared to a cocktail solution containing all seven ions together (each at 0.1 mg L−1). The pH of all the solutions was adjusted to pH 7.0 and one apple peel bead was then added to each flask and the flasks were shaken in an orbital mixer incubator at 25°C and 250 rpm. The concentrations of the ions in the solutions were then measured at 1.5, 10 and 24 h by ICP-MS.
2.11. Statistics
All the experiments were performed in triplicate and the results are expressed as mean ± SEM. Statistically significant difference (p < 0.05) with a 95% confidence interval was calculated using a two-way analysis of variance (ANOVA) coupled with a Bonferroni post-hoc test.
3. Results and discussion
3.1. Surface imaging
SEM-EDS were used to compare the surface morphology of sodium alginate beads with and without apple peel, particularly the variation in structure and geometry that contributed to the binding of the various ions to their surface. The micrographs of apple peel particles at 250X showed the presence of clusters of smaller particles adhering to each other (Figure )), while at 2000X the surface of these particles were uneven and rugged with smaller three-dimensional particles closely adhering to each other forming spaces between them (Figure )). The spectrograms obtained from EDS analysis showed the absence of the metals of interest (Figure )).
Figure 1. SEM-EDS images of apple peel particles. (a) micrograph at 250X (b) micrograph at 2000X (c) spectrogram showing C, O and K.
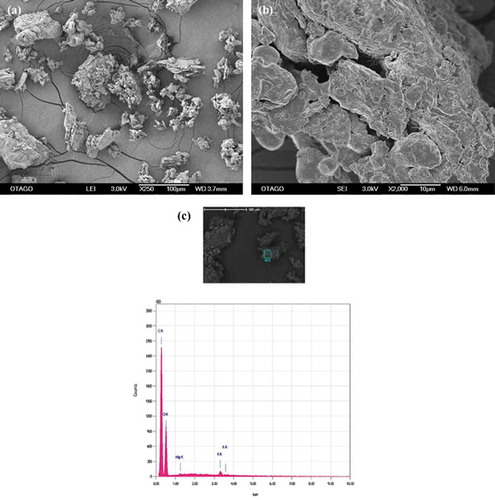
Apple peel and sodium alginate beads before biosorption were viewed at 50X and 2000X magnification. While the apple peel bead showed a rugged surface made of hills, valleys and dimples of various sizes (Figure )), the surface of the sodium alginate bead appeared smooth with dents, tiny pores and cracks distributed unevenly throughout (Figure )). The beads were spherical in shape and apple peel beads had a diameter > 1.8 mm, which were approximately twice the size of control beads (diameter < 1.0 mm) (Table ). Furthermore, apple peel beads had folds distributed throughout the surface whereas control beads had a much smoother surface, although crevices, pores and wrinkles were still visible at higher magnifications (Figure )). Similar results have been reported for calcium alginate beads (Song, Park, Kang, Park, & Han, Citation2013). The shape and texture of the peel immobilised and non-immobilised beads is in agreement with published work (Kumar et al., Citation2018) but not the size. This is due to the high peel particle to sodium alginate ratio (6:1) used to prepare the beads. This allowed the formation of dense sodium alginate-particle cross-linkages in the alginate matrix and hence increased the size of the beads. The texture of other beads was also reported to be rough and heterogeneous following the incorporation of lemon peel (Aichour, Zaghouane-Boudiaf, Iborra, & Polo, Citation2018) and coconut shell (Hassan, Abdel-Mohsen, & Fouda, Citation2014) particles, as compared to the smoother empty beads. The SEM image of dried lemon peel beads (Aichour et al., Citation2018) was very similar to the apple peel bead produced in this study.
Table 2. Summary of SEM-EDS analysis
Figure 2. SEM-EDS of beads before biosorption. (a) apple peel bead micrograph at 50X (b) apple peel bead micrograph at 2000X (c) control bead micrograph at 50X (d) control bead micrograph at 500 X (e) apple peel bead spectrogram (f) control bead spectrogram.
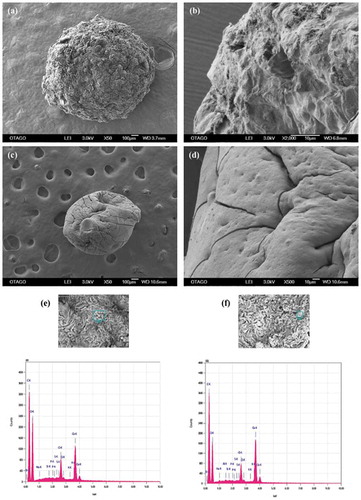
The EDS spectrograms before biosorption showed the absence of any of the ions of interest. However, other elements were identified throughout the bead surface (Figure )). The presence of calcium peaks on the bead surface confirms the contribution of ion-exchange in the biosorption process (Lessa et al., Citation2017). Prominent peaks of carbon, oxygen, sodium, magnesium, potassium and sulphur were expected as they are common elements found in alginate as well as apple peel. These were observed in the spectrograms and this has also been reported by other researchers examining orange peel (Lugo-Lugo, Barrera-Díaz, Ureña-Núñez, Bilyeu, & Linares-Hernández, Citation2012), mangrove-alginate composite bead (Abas, Ismail, Siajam, & Kamal, Citation2015), alginate and algal-biomass bead (Wang, Vincent, Faur, & Guibal, Citation2016).
SEM-EDS analysis of beads at 50X magnification after biosorption showed that the beads were swollen with a relatively smoother surface (Figure ), Table ). Apple peel bead at 2000X magnification revealed fewer hills and valleys and the outer layers were turned over partially closing the pores and dimples that were present prior to incubation (Figure )). The surface of the control bead at 2000X was folded inwards forming dents at many sites along with existing cracks on the surface of the bead (Figure )). Overall, the surface of the beads became smoother, the folds were more relaxed and the pores were smaller indicating fewer surface binding sites (Lessa et al., Citation2017; Song et al., Citation2013). This is explained by the interaction of biosorbate ions with the biosorbent functional groups that brings the polymeric chains closer and thus leads to the formation of a more compact, homogenous and regular surface (Lessa et al., Citation2017). Similar results have been reported for calcium alginate beads (S. Banerjee & A. Banerjee et al., Citation2018).The elements identified in the EDS spectrogram of apple peel beads after biosorption were As, Cr, Hg and Pb (Figure )), while control beads showed the presence of As, Cr, Cu, Pb and Ni (Figure )). The metals of interest were mostly stacked together at localised regions that were heterogeneously distributed and appeared as brighter spots when analysed by EDS.
Figure 3. SEM of beads after biosorption. One bead was incubated in a 0.1 mg L−1 cocktail solution of all seven ions for 10 h at pH 7.0, 25ºC and 250 rpm shaking. (a) apple peel bead micrograph at 50X (b) apple peel bead micrograph at 2000X (c) control bead micrograph at 50X (d) control bead micrograph at 500 X (e,f) EDS spectrogram of As, Cr, Hg and Pb attached to apple peel bead (g,h) EDS spectrogram of As, Cr, Cu, Pb and Ni attached to control beads.
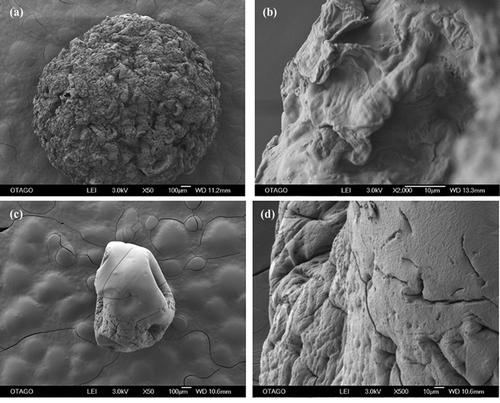
3.2. Effect of initial ph
The pH of the solution has a direct influence on biosorption as it affects the surface functional groups on the biosorbent as well as the degree of ionisation and solubility of the biosorbate ions (Da Silva Correia et al., Citation2018; Park et al., Citation2017; Ren et al., Citation2016; Vu et al., Citation2017; Zanin et al., Citation2017). Biosorbent functional groups also dissociate and their surface charge alters with a change in solution pH (Semerjian, Citation2018). In the present study, biosorption was studied in the pH range recommended by the World Health organisation for drinking water (i.e., 6.5 to 8.5). The results showed that the biosorption capacity of Cd dipped at pH 7.5 but was consistent at other pH values. pH 7.0 has been reported as optimal for Cd removal (Ince, Ince, Yonten, & Karaaslan, Citation2016). However, in other biosorption systems such macroalga (Mohamed et al., Citation2019), nano zerovalent iron (Boparai, Joseph, & O’Carroll, Citation2013) and activated biochar (Park et al., Citation2017), it decreased at pH 8.0 due to the formation of soluble hydroxyl complexes such as Cd(OH)+. Similar behavior was observed for Ni with a significant decrease at pH 7.5. But this increased again at higher pH values. This may be because of the onset of precipitate formation and at higher alkaline pH values this decrease in concentration of Ni ions in solution is a result of precipitation and may not be related to biosorption. This has been shown using pistachio hull waste (Beidokhti, Naeeni, & AbdiGhahroudi, Citation2019), inorganic oxide adsorbent (Ciesielczyk et al., Citation2013) and banana peel carbon (Van Thuan, Quynh, Nguyen, & Bach, Citation2017).
Biosorption capacities for Cu and Hg decreased significantly at alkaline pH. Precipitation of Cu(OH)2 and Hg(OH)2 occurs at pH > 5.0, thus decreasing its adsorption on the biosorbent (Deng, Zhang, Wang, Zheng, & Wang, Citation2015; Raza et al., Citation2015). However, we aimed to examine solutions having pH similar to that of drinking water, therefore results are reported for pH 6.5 to 8.5 where optimal pH was found to be 7.0 (Figure ); this is in agreement with results reported for other biosorbents (Santana, Dos Santos, Silva, & Das Virgens, Citation2016; Semerjian, Citation2018). For Pb there was a significant increase in the removal of ions from cocktail solution at pH 7.5 and 8.0. pH 8.0 has been reported to be optimal for Pb biosorption by potato peel (Chidi & Kelvin, Citation2018). Biosorption percentage of Cr and As by apple peel bead did not change significantly over the entire pH range. Furthermore, both Cr and As were the least adsorbed ions. The highest apple bead biosorption capacity was observed for Cd (1.05 mg g−1) followed by Cu (0.95 mgg−1), Ni (0.90 mg g−1), Hg (0.76 mg g−1) and Pb (0.75 mg g−1) (Figure ). Thus, qt values are lower compared to other experiments conducted in acidic pH environments.
Figure 4. Effect of initial pH on apple peel bead biosorption. One bead was incubated in a 0.1 mg L−1 cocktail solution of all seven ions for 24 h at 25ºC and 250 rpm shaking. The initial pH of each solution was adjusted to 6.5 to 8.5 ± 0.1. The points represent mean ± SEM for n = 3. *significantly different to all other pH conditions for Pb, p < 0.05.
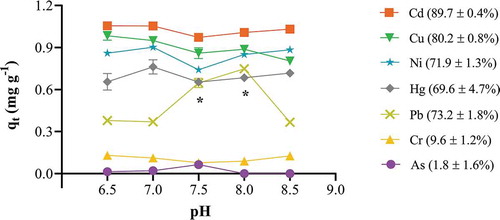
3.3. Effect of contact time (biosorption kinetics)
When conducting biosorption studies, other researchers have used various contact times ranging from one minute to seventy five days, depending on the biosorption system and the time required to reach equilibrium (Khiari, Wakkel, Abdelmoumen, & Jeguirim, Citation2019; Kim et al., Citation2016). Our studies focused on how control and apple peel beads behaved between 15 min and 72 h, as we aimed to develop an efficient drinking water purification system for home use. For apple peel beads equilibrium was ion-dependent. Specifically, equilibrium was reached after 6 and 10 h for Pb and Hg, respectively. For Cd and Ni equilibrium was reached after 24 h, while Cu took 48 h (Figure )). Apple peel beads provided superior biosorption because for control beads all ions required 48 h to reach equilibrium (Figure )). Initially rapid biosorption occurred and was followed by a slower removal of ions from cocktail solution. It has been postulated that this results from the increased probability of collision and migration of the ions to the surface of the biosorbent possessing free binding sites at the beginning, which gradually get occupied over time (Basu, Guha, & Ray, Citation2018; Deng et al., Citation2015; Li, Zeng, & Xue, Citation2018). The cumulative biosorption of all ions by apple peel beads was significantly higher (p ≤ 0.05) than control beads at all times except 15 min and 1 h (Figure ). Both As and Cr failed to show significant biosorption by either bead even though the EDS spectra revealed their presence on the bead surface (Table ). Similarly, even though Cd had the highest biosorption, it was missing from the EDS spectra for both beads and Cu and Ni were also absent from the surface of apple peel beads (Table ). This may be because the ions diffuse completely through the surface pores and into the centre of the bead. In contrast, the ions with significantly smaller biosorption remained adhered to the outer surface layer and were easily identified by EDS. Thus, the EDS spectra by itself is not a reliable measure of the efficiency of the biosorption and the results must be paired with a more direct method of ion detection in the solution.
Figure 5. Effect of contact time on bead biosorption. One bead was incubated in a 0.1 mg L−1 cocktail solution of all seven ions at pH 7.0, 25 ºC, for 15 min-72 h continuous shaking at 250 rpm. The points represent mean ± SEM for n = 3 with data analysis by nonlinear regression. (a) apple peel bead (b) control bead.
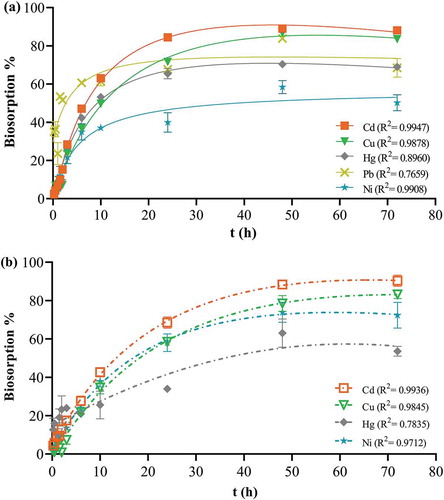
Figure 6. Cumulative biosorption at different contact time points. One bead was incubated in a 0.1 mg L−1 cocktail solution of all seven ions at pH 7.0, 25ºC, for 15 min-72 h continuous shaking at 250 rpm. The bars represent the mean ± SEM for n = 3. *Significantly different cumulative biosorption compared to corresponding control, p < 0.05. Only ions with significant biosorption are shown. AP = apple peel bead, C = control bead.
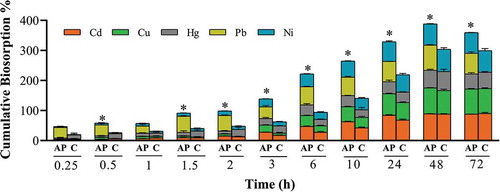
Kinetic data when modelled by standard biosorption models give an indication of the rate determining step in the process. Various kinetic models with specific and unique features explaining the type of mechanism and the efficiency of the biosorption system were used. The ions migrate from the bulk of the solution to the biosorbent surface diffusing initially through the liquid film surrounding the biosorbent particles governed by film diffusion (FD) followed by diffusion through the pores on the biosorbent surface known as particle or pore diffusion (PD). The ions then get attached to the functional groups on the biosorbent surface by either physical forces explained by pseudo-first order (PFO) or by forming chemical bonds given by pseudo-second order (PSO) (Sahmoune, Citation2018; Tan & Hameed, Citation2017) (Figure ). For chemisorption processes, the Elovich equation gives the initial biosorption velocity and the number of sites suitable for biosorption, assuming that the process is chemisorption (Schwantes et al., Citation2016). Modified biosorption models for multi-element systems were not applicable to this study due to the large variation in equilibrium times for the range of ions examined. Therefore, standard equations designed for single element systems were applied and this approach has been used for binary solutions by others (Hadi, Barford, & McKay, Citation2013).
Figure 7. Biosorption process. Representation of the migration of ions from the bulk of the solution to the inner layers of the apple peel bead.
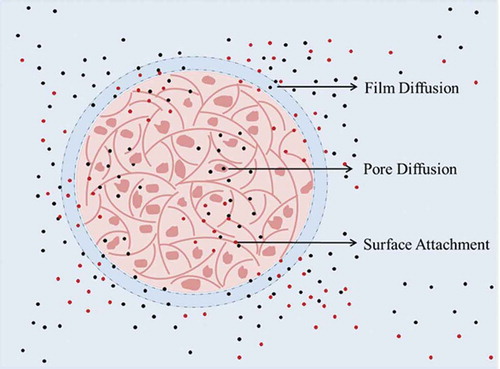
3.3.1. Film diffusion (FD) model
This model assumes that diffusion of the ions from the bulk of the solution to the biosorbent surface is the rate determining step (Mashkoor & Nasar, Citation2019). When the kinetic data was analysed by the FD model high R2 values for Cu and Ni biosorption by apple peel beads were produced. This was valid for the first 10 and 6 h, respectively, and Ni had the fastest rate of film diffusion (Figure )). For biosorption of Cd, Cu and Ni by control beads, the model was valid for the first 24 h (Table ). Since FD is the first step in the biosorption process, the validity of this model for 24 h confirms the slower uptake of ions by non-immobilised control beads. The FD rate was also faster for uptake of ions by apple peel beads as compared to control (Table ).
Table 3. Biosorption kinetic model constants
Figure 8. Biosorption kinetic models. One bead was incubated in a 0.1 mgL−1 cocktail solution of all seven ions at pH 7.0, 25ºC, for 15 min-72 h continuous shaking at 250 rpm. The points represent mean ± SEM for n = 3 with data analysis by linear regression. (a) Film diffusion showing results for contact time up to 10 h (b) pore diffusion (c) pseudo-first order showing results for contact time up to 10 h (d) pseudo-second order (e) Elovich plot showing results for contact time up to 3 h.
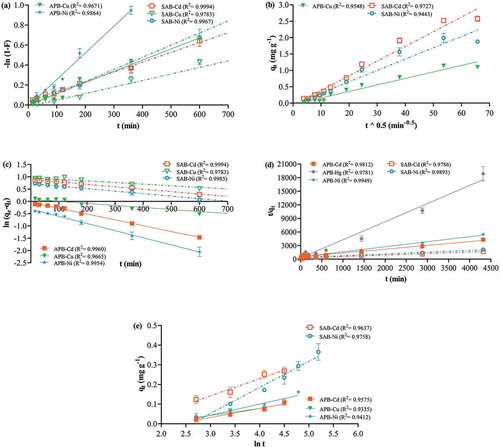
3.3.2. Particle or pore diffusion (PD)—weber-morris model
This model is based on the assumption that the internal mass transfer of the ions through surface pores and into the binding sites is the rate determining step (Sahmoune, Citation2018). This model was only valid for Cu biosorption by apple peel beads and for Cd and Ni for control beads (Figure )) and Cd exhibited the highest rate of pore diffusion (Table ).
3.3.3. Pseudo-first order (PFO) model
The PFO model is based on the assumption that the rate of the reaction is directly proportional to the difference in biosorption capacities at equilibrium at any given time point in the reaction (Ho & McKay, Citation1998). It incorporates the role of both film and pore diffusion in its biosorption rate mechanism (Deng et al., Citation2015; Plazinski, Citation2010). This model suggests that the electropositive ions are physisorbed (i.e. attracted and bound to the negatively charged functional groups on the biosorbent surface with weak van der Waal forces) (Romero-Cano, García-Rosero, Gonzalez-Gutierrez, Baldenegro-Pérez, & Carrasco-Marín, Citation2017). Results showed that the PFO model was valid for apple peel beads for the first 10 h for Cd and Ni and for Cu for 24 h. Furthermore, the rate of diffusion and physical adsorption of Cd and Ni was thrice as fast on apple peel bead as it was on the control bead (Table ), suggesting the presence of additional binding sites that reduced the overall time of biosorption by reaching equilibrium faster for immobilised apple peel beads. Additionally, Ni had the highest PFO rate followed by Cd and Cu (Figure )). The difference in biosorption rates among ions in a multi-ion solution is attributed to the chemical properties such as ionic radii, atomic weight etc of each metal ion as well as the competition for the binding sites on the surface (Lessa et al., Citation2017) and is unique to each biosorption system. This was also an observation made in our preliminary studies with other fruit peels where the biosorption rates of ions changed as the solution composition changed (Singh, Martin, & Rosengren, Citation2018). The PFO model suggests that the electropositive ions are physisorbed (Romero-Cano et al., Citation2017). This explains the electrostatic repulsion at the surface of the biosorbent beads and therefore the insignificant biosorption of the anions As and Cr.
3.3.4. Pseudo-second order (PSO) model
PSO is the preferred model that has been reported to fit biosorption data for most lignocellulosic biosorbents at low biosorbate concentrations (Khiari et al., Citation2019; Tan & Hameed, Citation2017). It validates chemical biosorption or sharing of electrons between biosorbent and biosorbate species as the rate determining step (Ho & McKay, Citation1999; Mashkoor & Nasar, Citation2019; Mondal, Samanta, Chakraborty, & Shaikh, Citation2018; Tejada-Tovar, González-Delgado, & Villabona-Ortíz, Citation2018; Wang et al., Citation2018). The PSO model relies on pore diffusion to explain the rate determining mechanism of the reaction (Plazinski, Dziuba, & Rudzinski, Citation2013). Results showed that the PSO model fit well until the last time point for Cd and Ni with both bead types and for Hg with apple peel beads (Figure )). Data for Cd and Ni also showed good fitting with PFO model which incorporates pore diffusion in determining the rate of reaction, thus strengthening the assumption that diffusion of ions through the pores of the beads may have been the slowest step in the biosorption process. Furthermore, the PSO rate for Cd and Ni was faster for apple peel beads compared to control, which was also seen with PFO model. The initial biosorption rate at time zero was higher for Cd and Ni uptake by control beads as compared to apple peel beads (Table ). This suggests that although diffusion through the pores of control beads prior to surface attachment is fast, there may be fewer active binding sites present compared to apple peel beads. This again, is in agreement to the conclusion from the kinetic observations in the PFO model. Among the seven ions in a cocktail solution, Hg had the highest rate of reaction. It appears, therefore, that Hg biosorption by apple peel bead may be limited by PSO reaction that suggests chemical interactions between the metal ion and the binding groups on the apple peel bead surface. PSO model fitting for Hg has also been demonstrated with other biosorbents such as Egyptian mandarin peel (Husein, Citation2013), Pachira aquatic (Santana et al., Citation2016), modified cassava and lemon peels (TejadaTovar et al., Citation2015), activated carbon prepared from Theobroma cacao pod husk (Kede et al., Citation2015) and chemically modified water hyacinth (El-Wakil et al., Citation2014).
3.3.5. Elovich equation
The Elovich equation was first used for describing adsorption of gases on solids but its use has been extended to the solid-liquid biosorption systems (Romero-Cano et al., Citation2017). This model supports the chemisorption theory of biosorption and assumes a heterogeneous biosorbent surface. However, it is not suitable for time points close to equilibrium, as the model does not take into account the possibility of desorption (Tan & Hameed, Citation2017; Tejada-Tovar et al., Citation2018). The Elovich equation could not be validated beyond the first 3 h for any ion with both bead types (Figure )). Chemisorption is not likely to take place within this time period because ion diffusion dominates during this time. Therefore, the uptake of ions is not via chemical interactions and desorption may also occur.
Ions with the best fit for the various models are shown in Table and an overall summary is represented by Figure . Overall, PFO was the best fitting model followed by FD and PSO, while the Elovich equation was not valid. Apple peel biosorption of Cd, Cu and Ni from a cocktail of seven ions showed that the rate of the reaction was governed by the physisorption-based PFO reaction whereas Hg fit well with the assumptions of the PSO model that supports chemisorption. However, as suggested by Plazinski et al. (Citation2013), a simple fitting of the kinetic data to the PSO model should not be interpreted as the applicability of the model as it also follows the behaviour predicted by other models including pore diffusion. Isotherm and thermodynamic studies indicating the energy exchanges are also required for confirming the chemical nature of Hg binding to the biosorbent bead. For control beads, both FD and PFO were the slowest steps in the uptake of Cd, Cu and Ni from the cocktail solution.
The values of equilibrium biosorption capacity (qe) calculated experimentally were compared with those obtained from kinetic models and were found to be closer to PFO than PSO (Table ), thus further validating the goodness of fit to the PFO model or physisorption. The poor fitting of the ions to Elovich equation supports evidence of the physical nature of the binding of the ions to the biosorbent beads. This weak binding could be advantageous as desorption of ions could be carried out easily using low energy to break the biosorbate-biosorbent bonds and thus, theoretically, the metal could be repurposed. Chatterjee and Schiewer (Citation2014), have stated that peel-immobilised beads are better biosorbents than peel particles and although they appear to be slower than particles, the accurate assessment of their faster kinetics can only be made when equivalent sizes of beads and particles are compared. Thus, it is recommended that the results from this study should be compared to biosorbent beads of a similar nature.
Table 4. Apple peel bead biosorption capacity
3.4. Effect of biosorbent concentration
To study the effect of biosorbent concentration on the removal of ions, 1 to 10 apple peel beads were incubated with the ion cocktail solution for 10 h. The results indicated that biosorbent concentration plays a direct role in the removal of some ions, but not all from a cocktail solution. While Cd, Cu, Pb and Ni showed an exponential decrease, the concentration of As, Cr and Hg did not change significantly with increase in biosorbent concentration (Figure ). Each biosorbent bead has a specific number of active sites and the addition of each bead increases the number of these available binding sites. This explains the high concentration of Cd, Cu, Pb and Ni in solution with lower biosorbent quantities. Therefore, the curves of these ions exponentially decrease and tend to plateau beyond which there was no significant decrease in ion concentration. The straight-line plot for Hg may be due to the simultaneous displacement of biosorbed Hg ions from the binding sites back in the solution by the other ions that may be competing for the same surface functional groups. Additionally, the surface of apple peel beads may not be suitable for binding As and Cr as explained earlier and therefore the concentration of these ions do not change significantly with an increase in the biosorbent concentration. Kołodyńska et al. (Citation2010) have shown pH 9–10 to be ideal for As (V) removal from waste water using ion-exchange mechanisms. Furthermore, Banerjee & Basu et al. (Citation2018) have used pH < 2 for binding of Cr (VI) onto a walnut shell based biosorbent. Since the experiments performed in this study were strictly for drinking water treatment using unmodified biosorbents, it is not surprising that optimal biosorption of As and Cr did not take place.
3.5. Presence of co-ions
Biosorption capacities, qsm and qcocktail were measured in single metal and cocktail solutions at three different time points (1.5, 10 and 24 h). The effect on biosorption capacity whether, synergistic, no effect or antagonistic due to the presence of co-ions was determined from the ratio (qcocktail/qsm)>1, (qcocktail/qsm) = 0 and (qcocktail/qsm)<1, respectively (Mahamadi, Citation2019; Mohan & Chander, Citation2001). The results showed that a near-synergism was found for Cd and Cu with the ratio value bordering one, whereas for all the other ions the ratio was less than one (Table ), thus indicating suppression in biosorption capacity as a result of the antagonistic effect by competing ions. Biosorption suppression could not be calculated for As with this formula as biosorption was non-significant in cocktail solution (Table ). Biosorption percentage was then compared in single metal and cocktail solutions using a two-way ANOVA coupled with a Bonferroni post hoc test. The results showed that all the ions (except Cd and Cu) had a statistically significant decrease in biosorption when in a cocktail (Figure ). Competitive adsorption of ions such as Cd, Cu, Pb, Ni, among others has been demonstrated for other biosorbents such as natural clay (Dho & Lee, Citation2003), mango biomasses (Reynel-Avila, Mendoza-Castillo, Olumide, & Bonilla-Petriciolet, Citation2016), modified biochar (Deng et al., Citation2017) and citrus peels (Singh & Shukla, Citation2017) among others. Several explanations have been given for this antagonistic effect in multiple ion solutions such as (i) the difference in the electronegativity of the various ions in solution competing for the active sites on the biosorbent surface (Basu et al., Citation2018; Petrovič & Simonič, Citation2016), (ii) the effect of hydrated ionic radii on electrostatic attractive forces that are involved in biosorbate and biosorbent interaction (Reynel-Avila et al., Citation2016), and (iii) the ionic radii of the ions (Basu et al., Citation2018; Petrovič & Simonič, Citation2016). Since seven ions with different properties were present in the cocktail solution, biosorption capacities obtained for each ion present were lower than that reported by other groups for single ion solutions. Indeed, biosorption is expected to be less effective in the presence of competing ions (Petrella et al., Citation2018).
Table 5. Apple peel bead biosorption capacity suppression in the presence of co-ions
Figure 10. Effect of the presence of co-ions on apple peel bead biosorption. One bead in 0.1 mg L−1 of either a single ion or cocktail solution containing all seven ions each at 0.1 mg L−1 at pH 7.0, 25ºC and 250 continuous shaking at 250 rpm for 1.5, 10 and 24 h. The points represent the mean ± SEM for n = 3. *Significantly different compared to the corresponding cocktail solution, p < 0.05. (a) arsenic (b) cadmium (c) chromium (d) copper (e) mercury (f) lead (g) nickel.
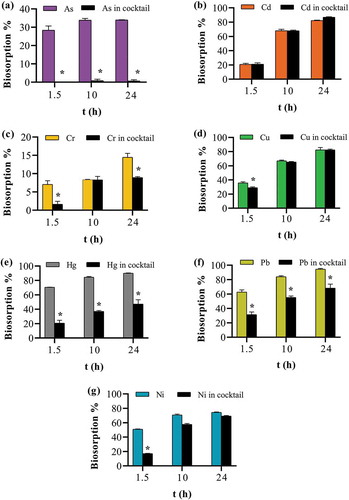
A number of reasons may explain the relatively low values of biosorption capacities obtained in the present study. Firstly, the low initial concentration of ions in solution resulted in a corresponding smaller amount of ions biosorbed per gram of bead. Secondly, a neutral pH of the solution was desired in order to mimic drinking water, which is in contrast to the most studies that rely on a highly acidic pH for optimal biosorption. Lastly, multiple ions in solution likely compete for the same binding sites and thus, equivalent removal compared to single ions cannot be expected. Overall, the efficiency of apple peel immobilised on a sodium alginate bead may be determined by its ability to remove a higher percentage metals from solution compared to control beads. This ability to remove a range of heavy metals and ions indicates that this type of bead preparation has the potential to be adapted to drinking water conditions and warrants further examination.
4. Conclusion
Apple peel was immobilised as beads using sodium alginate and its performance was compared with that of non-immobilised sodium alginate control beads. Results showed that the surface of the apple peel beads was more favourable for the binding of the ions compared to control beads. Biosorption of ions by apple peel beads at neutral pH showed that Cd was readily removed, while Cr and As remained in the simulated drinking water. An increase in the biosorption concentration of apple peel beads increased the removal of Cd, Cu, Pb and Ni, whereas the concentration of As, Cr and Hg did not change. Thus, these beads are not likely to be useful for As Cr or Hg removal from drinking water. However, biosorption kinetics showed that apple peel beads were superior to control beads and could remove a larger percentage of the total metals present. Importantly, these beads retained efficacy in a multi ion solution, which is more representative of actual drinking water. This indicates that these beads have the potential to be used for decontamination of drinking water and thus the utility of these beads warrants further examination and optimisation.
List of Abbreviations
As: | = | Arsenic |
cad: | = | Concentration adsorbed (mg L−1) |
Cd: | = | Cadmium |
co: | = | Initial concentration (mg L−1) |
Cr: | = | Chromium |
ct: | = | Concentration at time “t”(mg L−1) |
Cu: | = | Copper |
EDS: | = | Energy dispersive spectroscopy |
FD: | = | Film diffusion |
Hg: | = | Mercury |
HM: | = | Heavy metals |
ICP-MS: | = | Inductively coupled plasma-mass spectrometry |
k1: | = | Rate constant for PFO |
k2: | = | Rate constant for PSO |
kFD: | = | Rate constant for film diffusion |
kPD: | = | Rate constant for pore diffusion |
m: | = | Weight of biosorbent (g) |
Ni: | = | Nickel ion |
Pb: | = | Lead |
PD: | = | Pore diffusion |
PFO: | = | Pseudo first order |
PSO: | = | Pseudo second order |
qe: | = | Equilibrium biosorption capacity (mg g−1) |
qt: | = | Biosorption capacity at time “t” (mg g−1) |
R2: | = | Coefficient of determination |
SA: | = | Sodium alginate |
SEM: | = | Scanning electron microscopy |
t: | = | Contact time |
V: | = | Volume of solution (mL) |
Competing Interests
The authors declare no competing interests.
Additional information
Funding
Notes on contributors
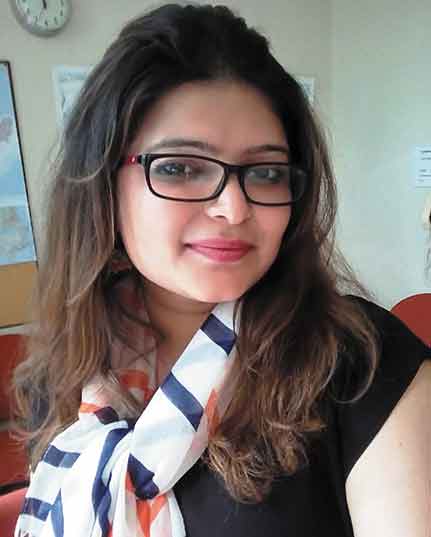
Risha Jasmine Singh
Risha Jasmine Singh is a PhD student in the Departments of Pharmacology and Toxicology and Geology
Candace E. Martin
Candace E. Martin is a Senior Lecturer in the Department of Geology with research interests in environmental geology and trace element geochemistry
Dave Barr
Dave Barr is an Instrument Support Technician in the Chemistry Department
Rhonda J. Rosengren
Rhonda J. Rosengren is a Toxicology Professor in the Department of Pharmacology and Toxicology. She has research interests in environmental toxicology.
References
- Abas, S. N. A., Ismail, M. H. S., Siajam, S. I., & Kamal, M. L. (2015). Development of novel adsorbent-mangrove-alginate composite bead (MACB) for removal of Pb (II) from aqueous solution. Journal of the Taiwan Institute of Chemical Engineers, 50, 182–24. doi:10.1016/j.jtice.2014.11.013
- Acharya, J., Kumar, U., & Rafi, P. M. (2018) Removal of Heavy metal ions from wastewater by chemically modified agricultural waste material as potential adsorbent-a review. International Journal of Current Engineering and Technology, 8(3), 526–530.
- Afroze, S., & Sen, T. K. (2018). A review on heavy metal ions and dye adsorption from water by agricultural solid waste adsorbents. Water, Air, & Soil Pollution, 229(7), 225.
- Aichour, A., Zaghouane-Boudiaf, H., Iborra, C. V., & Polo, M. S. (2018). Bioadsorbent beads prepared from activated biomass/alginate for enhanced removal of cationic dye from water medium: Kinetics, equilibrium and thermodynamic studies. Journal of Molecular Liquids, 256, 533–540. doi:10.1016/j.molliq.2018.02.073
- Azimi, A., Azari, A., Rezakazemi, M., & Ansarpour, M. (2017). Removal of heavy metals from industrial wastewaters: A review. ChemBioEng Reviews, 4, 37–59. doi:10.1002/cben.201600010
- Banerjee, M., Basu, R. K., & Das, S. K. (2018). Cr(VI) adsorption by a green adsorbent walnut shell: Adsorption studies, regeneration studies, scale-up design and economic feasibility. Process Safety and Environmental Protection, 116, 693–702. doi:10.1016/j.psep.2018.03.037
- Banerjee, S., Banerjee, A., & Sarkar, P. (2018). Statistical optimization of arsenic biosorption by microbial enzyme via Ca-alginate beads. Journal of Environmental Science and Health, Part A, 53, 436–442. doi:10.1080/10934529.2017.1409009
- Barbier, O., Jacquillet, G., Tauc, M., Cougnon, M., & Poujeol, P. (2005). Effect of heavy metals on, and handling by, the kidney. Nephron Physiology, 99, p105–p110. doi:10.1159/000083981
- Basu, M., Guha, A. K., & Ray, L. (2018). Adsorption of cadmium on cucumber peel: Kinetics, isotherm and Co-ion effect. Indian Chemical Engineer, 60, 179–195. doi:10.1080/00194506.2017.1341349
- Beidokhti, M. Z., Naeeni, S. T. O., & AbdiGhahroudi, M. S. (2019). Biosorption of Nickel (II) from aqueous solutions onto pistachio hull waste as a low-cost biosorbent. Civil Engineering Journal, 5, 447–457. doi:10.28991/cej-2019-03091259
- Boparai, H. K., Joseph, M., & O’Carroll, D. M. (2013). Cadmium (Cd(2+)) removal by nano zerovalent iron: Surface analysis, effects of solution chemistry and surface complexation modeling. Environmental Science and Pollution Research, 20, 6210–6221. doi:10.1007/s11356-013-1651-8
- Chand, P., Bafana, A., & Pakade, Y. B. (2015a). Xanthate modified apple pomace as an adsorbent for removal of Cd (II), Ni (II) and Pb (II), and its application to real industrial wastewater. International Biodeterioration & Biodegradation, 97, 60–66. doi:10.1016/j.ibiod.2014.10.015
- Chand, P., & Pakade, Y. B. (2013). Removal of Pb from water by adsorption on apple pomace: equilibrium, kinetics, and thermodynamics studies.Journal of Chemistry 2013, 1–9.
- Chand, P., & Pakade, Y. B. (2015b). Utilization of chemically modified apple juice industrial waste for removal of Ni2+ ions from aqueous solution. Journal of Material Cycles and Waste Management, 17, 163–173. doi:10.1007/s10163-014-0236-z
- Chatterjee, A., & Schiewer, S. (2014). Multi-resistance kinetic models for biosorption of Cd by raw and immobilized citrus peels in batch and packed-bed columns. Chemical Engineering Journal, 244, 105–116. doi:10.1016/j.cej.2013.12.017
- Cheok, C. Y., Mohd Adzahan, N., Abdul Rahman, R., Zainal Abedin, N. H., Hussain, N., Sulaiman, R., & Chong, G. H. (2018). Current trends of tropical fruit waste utilization. Critical Reviews in Food Science and Nutrition, 58, 335–361. doi:10.1080/10408398.2016.1176009
- Chidi, O., & Kelvin, R. (2018). Surface interaction of sweet potato peels (Ipomoea batata) with Cd (II) and Pb (II) ions in aqueous medium. Chemie International, 4, 221–229.
- Ciesielczyk, F., Bartczak, P., Wieszczycka, K., Siwińska-Stefańska, K., Nowacka, M., & Jesionowski, T. (2013). Adsorption of Ni(II) from model solutions using co-precipitated inorganic oxides. Adsorption, 19, 423–434. doi:10.1007/s10450-012-9464-5
- Da Silva Correia, I. K., Santos, P. F., Santana, C. S., Neris, J. B., Luzardo, F. H., & Velasco, F. G. (2018). Application of coconut shell, banana peel, spent coffee grounds, eucalyptus bark, piassava (Attalea funifera) and water hyacinth (Eichornia crassipes) in the adsorption of Pb2+ and Ni2+ ions in water. Journal of Environmental Chemical Engineering, 6, 2319–2334. doi:10.1016/j.jece.2018.03.033
- Deng, J., Liu, Y., Liu, S., Zeng, G., Tan, X., Huang, B., … Yan, Z. (2017). Competitive adsorption of Pb(II), Cd(II) and Cu(II) onto chitosan-pyromellitic dianhydride modified biochar. Journal of Colloid and Interface Science, 506, 355–364. doi:10.1016/j.jcis.2017.07.069
- Deng, S., Zhang, G., Wang, X., Zheng, T., & Wang, P. (2015). Preparation and performance of polyacrylonitrile fiber functionalized with iminodiacetic acid under microwave irradiation for adsorption of Cu(II) and Hg(II). Chemical Engineering Journal, 276, 349–357. doi:10.1016/j.cej.2015.04.043
- Dho, N. Y., & Lee, S. R. (2003). Effect of temperature on single and competitive adsorptions of Cu(II) and Zn(II) onto natural clays. Environmental Monitoring and Assessment, 83, 177–203.
- El-Wakil, A., El-Maaty, W . A., & Awad, F. (2014). Studies on biosorption of mercury (ii) from aqueous solution on nitric acid modified activated carbon prepared from water hyacinth. ABC Journal Of Advanced Research, 3(1), 51–71.
- Enniya, I., Rghioui, L., & Jourani, A. (2018). Adsorption of hexavalent chromium in aqueous solution on activated carbon prepared from apple peels. Sustainable Chemistry and Pharmacy, 7, 9–16. doi:10.1016/j.scp.2017.11.003
- Febrianto, J., Kosasih, A. N., Sunarso, J., Ju, Y.-H., Indraswati, N., & Ismadji, S. (2009). Equilibrium and kinetic studies in adsorption of heavy metals using biosorbent: A summary of recent studies. Journal of Hazardous Materials, 162, 616–645. doi:10.1016/j.jhazmat.2008.06.042
- Foo, K., & Hameed, B. (2010). Insights into the modeling of adsorption isotherm systems. Chemical Engineering Journal, 156, 2–10. doi:10.1016/j.cej.2009.09.013
- Hadi, P., Barford, J., & McKay, G. (2013). Synergistic effect in the simultaneous removal of binary cobalt–Nickel heavy metals from effluents by a novel e-waste-derived material. Chemical Engineering Journal, 228, 140–146. doi:10.1016/j.cej.2013.04.086
- Hassan, A., Abdel-Mohsen, A., & Fouda, M. M. (2014). Comparative study of calcium alginate, activated carbon, and their composite beads on methylene blue adsorption. Carbohydrate Polymers, 102, 192–198. doi:10.1016/j.carbpol.2013.10.104
- Ho, Y., & McKay, G. (1998). A comparison of chemisorption kinetic models applied to pollutant removal on various sorbents. Process Safety and Environmental Protection, 76, 332–340. doi:10.1205/095758298529696
- Ho, Y.-S., & McKay, G. (1999). Pseudo-second order model for sorption processes. Process Biochemistry, 34, 451–465. doi:10.1016/S0032-9592(98)00112-5
- Husein, D. Z. (2013). Adsorption and removal of mercury ions from aqueous solution using raw and chemically modified Egyptian mandarin peel. Desalination and Water Treatment, 51(34–36), 6761–6769.
- Ince, M., Ince, O. K., Yonten, V., & Karaaslan, N. M. (2016). Nickel, lead, and cadmium removal using a low-cost adsorbent-banana peel. Atomic Spectroscopy, 37, 125–130.
- Irem, S., Islam, E., Khan, Q. M., Ul Haq, M. A., & Hashmat, A. J. (2017). Adsorption of arsenic from drinking water using natural orange waste: kinetics and fluidized bed column studies. Water science and Technology: Water Supply.
- Jakóbik-Kolon, A., Bok-Badura, J., Karoń, K., Mitko, K., & Milewski, A. (2017). Hybrid pectin-based biosorbents for zinc ions removal. Carbohydrate Polymers, 169, 213–219. doi:10.1016/j.carbpol.2017.03.095
- Jawad, R. J., Sha, M. H., Siajam, I., Ismail, M. H. S., & Siajam, S. I. (2016). Removal of heavy metals and C industrial wastewater by novel adsorbent of alginate and mang coated by chitosan. Journal of Purity, Utility Reaction and Environment, 5, 118–129.
- Kandari, V., & Gupta, S. (2017). Bioconversion of vegetable and fruit peel wastes in viable product. Journal of Microbiology and Biotechnology Research, 2, 308–312.
- Kede, C. M., Ndibewu, P. P., Kalumba, M. M., Panichev, N. A., Ngomo, H. M., & Ketcha, J. M. (2015). Adsorption of mercury (ii) onto activated carbons derived from theobroma cacao pod husk. South African Journal of Chemistry, 68, 226–235.
- Khatoon, H., & Rai, J. P. N. (2016). Agricultural Waste Materials As Biosorbents For The Removal Of Heavy Metals And Synthetic Dyes-A Review. Octa Journal of Environmental Research, 4(3), 208–229.
- Khiari, B., Wakkel, M., Abdelmoumen, S., & Jeguirim, M. (2019). Dynamics and kinetics of cupric ion removal from wastewaters by tunisian solid crude olive-oil waste. Materials, 12, 365. doi:10.3390/ma12030365
- Kim, Y., Bae, J., Park, H., Suh, J.-K., You, Y.-W., & Choi, H. (2016). Adsorption dynamics of methyl violet onto granulated mesoporous carbon: Facile synthesis and adsorption kinetics. Water Research, 101, 187–194. doi:10.1016/j.watres.2016.04.077
- Kołodyńska, D., Siek, M., Hubicki, Z., Grabarczyk, M., Skwarek, E., & Janusz, W. (2010). Chromium and Arsenic Removal in the Presence of Complexing Agent of New Generation. Paper presented at the 15th International Conference on Heavy Metals in the Environment, Gdansk (Poland).
- Kratochvil, D., & Volesky, B. (1998). Advances in the biosorption of heavy metals. Trends in Biotechnology,16(7), 291–300.
- Kumar, R., Kim, S.-J., Kim, K.-H., Lee, S.-H., Park, H.-S., & Jeon, B.-H. (2018). Removal of hazardous hexavalent chromium from aqueous phase using zirconium oxide-immobilized alginate beads. Applied Geochemistry, 88, 113–121. doi:10.1016/j.apgeochem.2017.04.002
- Lai, Y.-L., Annadurai, G., Huang, F.-C., & Lee, J.-F. (2008). Biosorption of Zn(II) on the different Ca-alginate beads from aqueous solution. Bioresource Technology, 99, 6480–6487. doi:10.1016/j.biortech.2007.11.041
- Lee, S. H., Jung, C. H., Chung, H., Lee, M. Y., & Yang, J.-W. (1998). Removal of heavy metals from aqueous solution by apple residues. Process Biochemistry, 33, 205–211. doi:10.1016/S0032-9592(97)00055-1
- Lee, S.-H., & Yang, J.-W. (1997). Removal of copper in aqueous solution by apple wastes. Separation Science and Technology, 32, 1371–1387. doi:10.1080/01496399708000966
- Lessa, E. F., Medina, A. L., Ribeiro, A. S., & Fajardo, A. R. (2017). Removal of multi-metals fromwater using reusable pectin/cellulose microfibers composite beads. Arabian Journal of Chemistry https://doi.org/10.1016/j.arabjc.2017.07.011.
- Li, S., Zeng, Z., & Xue, W. (2018). Adsorption of lead ion from aqueous solution by modified walnut shell: kinetics and thermodynamics. Environmental Technology 1–11. doi:10.1080/09593330.2018.1480664
- Lugo-Lugo, V., Barrera-Díaz, C., Ureña-Núñez, F., Bilyeu, B., & Linares-Hernández, I. (2012). Biosorption of Cr(III) and Fe(III) in single and binary systems onto pretreated orange peel.. Journal of Environmental Management, 112, 120–127. doi:10.1016/j.jenvman.2012.07.009
- Mahamadi, C. (2019). On the dominance of Pb during competitive biosorption from multi-metal systems: A review. Cogent Environmental Science, 5(1), 1635335.
- Mallampati, R., & Valiyaveettil, S. (2013). Apple peels—A versatile biomass for water purification? ACS Applied Materials & Interfaces, 5, 4443–4449. doi:10.1021/am400901e
- Maranon, E., & Sastre, H. (1992a). Preconcentration and removal of trace metals from water by apple waste. Bioresource Technology, 40, 73–76. doi:10.1016/0960-8524(92)90122-E
- Mashkoor, F., & Nasar, A. (2019). Preparation, characterization and adsorption studies of the chemically modified Luffa aegyptica peel as a potential adsorbent for the removal of malachite green from aqueous solution. Journal of Molecular Liquids, 274, 315–327. doi:10.1016/j.molliq.2018.10.119
- Massimi, L., Giuliano, A., Astolfi, M., Congedo, R., Masotti, A., & Canepari, S. (2018). The effect of plasma pretreatment and cross-linking degree on the physical and antimicrobial properties of Nisin-Coated PVA films. Materials, 11, 334. doi:10.3390/ma11081451
- Milani, P. A., Consonni, J. L., Labuto, G., Carrilho, E. N. V. M. (2018). Environ Sci Pollut Res, 25(36), 35906. https://doi.org/10.1007/s11356-018-1726-7
- Mohamed, H. S., Soliman, N., Abdelrheem, D. A., Ramadan, A. A., Elghandour, A. H., & Ahmed, S. A. (2019). Adsorption of Cd2+ and Cr3+ ions from aqueous solutions by using residue of Padina gymnospora waste as promising low-cost adsorbent. Heliyon, 5, e01287. doi:10.1016/j.heliyon.2019.e01287
- Mohan, D., & Chander, S. (2001). Single component and multi-component adsorption of metal ions by activated carbons. Colloids and Surfaces A: Physicochemical and Engineering Aspects, 177, 183–196. doi:10.1016/S0927-7757(00)00670-1
- Mondal, N. K., Samanta, A., Chakraborty, S., & Shaikh, W. A. (2018). Enhanced chromium(VI) removal using banana peel dust: Isotherms, kinetics and thermodynamics study. Sustainable Water Resources Management, 4, 489–497. doi:10.1007/s40899-017-0130-7
- Mousa, N. E., Simonescu, C. M., Pătescu, R.-E., Onose, C., Tardei, C., Culiţă, D. C., … Lavric, V. (2016). Pb2+ removal from aqueous synthetic solutions by calcium alginate and chitosan coated calcium alginate. Reactive and Functional Polymers, 109, 137–150. doi:10.1016/j.reactfunctpolym.2016.11.001
- Mudhoo, A., Garg, V. K., & Wang, S. (2012). Environmental chemistry for a sustainable world (pp. 379–442). Basal: Springer.
- Neris, J. B., Luzardo, F. H. M., Da Silva, E. G. P., & Velasco, F. G. (2018). Evaluation of adsorption processes of metal ions in multi-element aqueous systems by lignocellulosic adsorbents applying different isotherms: a critical review.Chemical Engineering Journal, 357, 404–420.
- Park, C. M., Han, J., Chu, K. H., Al-Hamadani, Y. A., Her, N., Heo, J., & Yoon, Y. (2017). Influence of solution pH, ionic strength, and humic acid on cadmium adsorption onto activated biochar: Experiment and modeling. Journal of Industrial and Engineering Chemistry, 48, 186–193. doi:10.1016/j.jiec.2016.12.038
- Pathak, P. D., Mandavgane, S. A., & Kulkarni, B. D. (2017). Fruit peel waste:Characterization and its potential uses. Current Science, 113, 1–11. doi:10.18520/cs/v113/i03/444-454
- Paul, E., Nwoken, N., & Anumonye, F. (2018). Biosorption of heavy metals (Cd 2+, Cr 3+, Cu 2+, Ni2+, Pb 2+ And Zn2+) from aqueous solution onto activated carbon prepared from chicken feather. ATBU Journal of Science, Technology and Education, 6, 194–205.
- Petrella, A., Spasiano, D., Acquafredda, P., De Vietro, N., Ranieri, E., Cosma, P., … Petruzzelli, D. (2018). Heavy metals retention (Pb(II), Cd(II), Ni(II)) from single and multimetal solutions by natural biosorbents from the olive oil milling operations. Process Safety and Environmental Protection, 114, 79–90. doi:10.1016/j.psep.2017.12.010
- Petrovič, A., & Simonič, M. (2016). Removal of heavy metal ions from drinking water by alginate-immobilised Chlorella sorokiniana. International Journal of Environmental Science and Technology, 13, 1761–1780. doi:10.1007/s13762-016-1015-2
- Plazinski, W. (2010). Applicability of the film-diffusion model for description of the adsorption kinetics at the solid/solution interfaces. Applied Surface Science, 256, 5157–5163. doi:10.1016/j.apsusc.2009.12.083
- Plazinski, W., Dziuba, J., & Rudzinski, W. (2013). Modeling of sorption kinetics: The pseudo-second order equation and the sorbate intraparticle diffusivity. Adsorption, 19, 1055–1064. doi:10.1007/s10450-013-9529-0
- Raza, M. H., Sadiq, A., Farooq, U., Athar, M., Hussain, T., Mujahid, A., & Salman, M. (2015). Phragmites karka as a biosorbent for the removal of mercury metal ions from aqueous solution: Effect of modification. Journal of Chemistry, 2015, 1–12.
- Ren, H., Gao, Z., Wu, D., Jiang, J., Sun, Y., & Luo, C. (2016). Efficient Pb(II) removal using sodium alginate-carboxymethyl cellulose gel beads: Preparation, characterization, and adsorption mechanism. Carbohydrate Polymers, 137, 402–409. doi:10.1016/j.carbpol.2015.11.002
- Reynel-Avila, H. E., Mendoza-Castillo, D. I., Olumide, A. A., & Bonilla-Petriciolet, A. (2016). A survey of multi-component sorption models for the competitive removal of heavy metal ions using bush mango and flamboyant biomasses. Journal of Molecular Liquids, 224, 1041–1054. doi:10.1016/j.molliq.2016.10.061
- Romero-Cano, L. A., García-Rosero, H., Gonzalez-Gutierrez, L. V., Baldenegro-Pérez, L. A., & Carrasco-Marín, F. (2017). Functionalized adsorbents prepared from fruit peels: Equilibrium, kinetic and thermodynamic studies for copper adsorption in aqueous solution. Journal of Cleaner Production, 162, 195–204. doi:10.1016/j.jclepro.2017.06.032
- Sahmoune, M. N. (2018). Green adsorbents for pollutant removal (pp. 193–213). Basal: Springer.
- Santana, A. J., Dos Santos, W. N., Silva, L. O., & Das Virgens, C. F. (2016). Removal of mercury(II) ions in aqueous solution using the peel biomass of Pachira aquatica Aubl: Kinetics and adsorption equilibrium studies. Environmental Monitoring and Assessment, 188, 293. doi:10.1007/s10661-016-5266-7
- Schwantes, D., Gonçalves, A. C., Coelho, G. F., Campagnolo, M. A., Dragunski, D. C., Tarley, C. R. T., … Leismann, E. A. V. (2016). Journal of Chemistry, 2016, 1–15. doi:10.1155/2016/3694174
- Semerjian, L. (2018). Removal of heavy metals (Cu, Pb) from aqueous solutions using pine (Pinus halepensis) sawdust: Equilibrium, kinetic, and thermodynamic studies. Environmental Technology & Innovation, 12, 91–103. doi:10.1016/j.eti.2018.08.005
- Singh, L., Pavankumar, A. R., Lakshmanan, R., & Rajarao, G. K. (2012). Effective removal of Cu2+ ions from aqueous medium using alginate as biosorbent. Ecological Engineering, 38, 119–124. doi:10.1016/j.ecoleng.2011.10.007
- Singh, R. J., Martin, C. E., & Rosengren, R. J. (2018). Biomedical postgraduate symposium (pp. 10). New Zealand: University of Otago.
- Singh, S., & Shukla, S. R. (2017). Theoretical studies on adsorption of Ni (II) from aqueous solution using Citrus limetta peels. Environmental Progress & Sustainable Energy, 36(3), 864–872.
- Song, D., Park, S.-J., Kang, H. W., Park, S. B., & Han, J.-I. (2013). Recovery of Lithium(I), Strontium(II), and Lanthanum(III) Using Ca–Alginate Beads. Journal of Chemical & Engineering Data, 58, 2455–2464. doi:10.1021/je400317v
- Tan, K., & Hameed, B. (2017). Insight into the adsorption kinetics models for the removal of contaminants from aqueous solutions. Journal of the Taiwan Institute of Chemical Engineers, 74, 25–48.
- Tchounwou, P. B., Yedjou, C. G., Patlolla, A. K., & Sutton, D. J. (2012). Heavy metal toxicity and the environment. Molecular, clinical and environmental toxicology (pp. 133–164). Basel: Springer.
- Tejada-Tovar, C., González-Delgado, Á., & Villabona-Ortíz, Á. (2018). Adsorption Kinetics of Orange Peel Biosorbents for Cr (VI) Uptake from Water. Contemporary Engineering Sciences, 11(24), 1185–1193.
- Tejada-Tovar, C., Villabona-Ortiz, Á., & Garcés-Jaraba, L. E. (2015). Kinetics of adsorption in mercury removal using cassava (manhiot esculenta) and lemon (Citrus limonum) wastes modified with citric acid. Ingeniería Y Universidad, 19(2), 283–298.
- Van Thuan, T., Quynh, B. T. P., Nguyen, T. D., & Bach, L. G. (2017). Response surface methodology approach for optimization of Cu 2+, Ni 2+ and Pb 2+ adsorption using KOH-activated carbon from banana peel. Surfaces and Interfaces, 6, 209–217. doi:10.1016/j.surfin.2016.10.007
- Vandenbossche, M., Jimenez, M., Casetta, M., & Traisnel, M. (2015). Remediation of heavy metals by biomolecules: A review. Critical Reviews in Environmental Science and Technology, 45, 1644–1704. doi:10.1080/10643389.2014.966425
- Vu, H. C., Dwivedi, A. D., Le, T. T., Seo, S.-H., Kim, E.-J., & Chang, Y.-S. (2017). Magnetite graphene oxide encapsulated in alginate beads for enhanced adsorption of Cr(VI) and As(V) from aqueous solutions: Role of crosslinking metal cations in pH control. Chemical Engineering Journal, 307, 220–229. doi:10.1016/j.cej.2016.08.058
- Wang, R.-Z., Huang, D.-L., Liu, Y.-G., Zhang, C., Lai, C., Zeng, G.-M., … Luo, H. (2018). Investigating the adsorption behavior and the relative distribution of Cd2+ sorption mechanisms on biochars by different feedstock. Bioresource Technology, 261, 265–271. doi:10.1016/j.biortech.2018.04.032
- Wang, S., Vincent, T., Faur, C., & Guibal, E. (2016). Alginate and algal-based beads for the sorption of metal cations: Cu(II) and Pb(II). International Journal of Molecular Sciences, 17, 1453. doi:10.3390/ijms17091453
- Yasim, N. S. E. M., Ismail, Z. S., Zaki, S. M., & Azis, M. F. A. (2016). Adsorption of Cu, As, Pb and Zn by banana trunk. Malaysian Journal of Analytical Sciences, 20, 187–196. doi:10.17576/mjas
- Zanin, E., Scapinello, J., de Oliveira, M., Rambo, C. L., Franscescon, F., Freitas, L., … Dal Magro, J. (2017). Adsorption of heavy metals from wastewater graphic industry using clinoptilolite zeolite as adsorbent. Process Safety and Environmental Protection, 105, 194–200. doi:10.1016/j.psep.2016.11.008
- Zhao, L., Wang, J., Zhang, P., Gu, Q., & Gao, C. (2018). Absorption of Heavy Metal Ions by Alginate. Bioactive seaweeds for food applications (pp. 255–268). Amsterdam: Elsevier.