Abstract
The objective of this work was to examine the influence of compressive force magnitude on a functional spinal unit’s (FSU) flexion–extension neutral zone measured during pure moment (PM) tests. Each porcine cervical FSU received four repeats of a PM test with 10, 300, 900 and 1,800 N of compressive force, in a randomized order. Increasing the magnitude of compression significantly decreased the neutral zone range (p < 0.001), while increasing passive stiffness (p < 0.001). The flexion limit at 10 N was significantly lower (p < 0.05) than the other loading conditions. Reporting the compressive force magnitude is important when posture is a standardized experimental factor considered in the design of in vitro spine biomechanics studies.
Public Interest Statement
Low-back pain originating from intervertebral discs accounts for a large proportion of cases. Both the type and location of the intervertebral disc damage are influenced by posture during repetitive loading, and therefore it is an important factor to consider when conducting in vitro spine biomechanics tests. The limits (maximum flexion and extension angles) of spine posture can be standardized by identifying each specimen’s neutral zone range, which is defined as the region of minimal passive stiffness. However, this range significantly decreases as the magnitude of compressive force increases. Therefore, based on the results of this work, explicitly stating the magnitude of compressive force in the methodology section is encouraged in order to allow for meaningful comparisons of the effect of test posture across studies.
1. Introduction
The neutral zone, defined as the region of minimal passive stiffness (Panjabi, Citation1992), has been used to standardize the test posture of functional spinal units (FSUs) for in vitro biomechanical studies (Balkovec & McGill, Citation2012; Callaghan & McGill, Citation2001; Gooyers, McMillan, Howarth, & Callaghan, Citation2012; Howarth, Gallagher, & Callaghan, Citation2013). Low-back pain originating from intervertebral discs accounts for a large proportion of cases (DePalma, Ketchum, & Saullo, Citation2011; Schwarzer et al., Citation1995) and, therefore, specimen posture, which influences both the type and location of intervertebral disc injury, is an important factor to consider when conducting in vitro studies (Aultman, Scannell, & McGill, Citation2005; Callaghan & McGill, Citation2001; Veres, Robertson, & Broom, Citation2009). Deviated postures alter a FSU’s ultimate compressive failure tolerance (Adams & Hutton, Citation1981; Gunning, Callaghan, & McGill, Citation2001), the stress distribution on passive tissues (Adams, Freeman, Morrison, Nelson, & Dolan, Citation2000; Adams, McNally, Wagstaff, & Goodship, Citation1993; Aultman et al., Citation2005; Ayturk, Garcia, & Puttlitz, Citation2010) and the hydrostatic pressure inside the intervertebral disc (Wilke, Neef, Caimi, Hoogland, & Claes, Citation1999). Recent work conducted in our laboratory (Gooyers et al., Citation2012) further emphasizes the impact of posture, illustrating how posture can interact with other mechanical risk factors (e.g. vibration) to alter FSU mechanics. In general, the margin of safety decreases as the deviation from the neutral posture increases (Scannell & McGill, Citation2003); however, individual variability in tissue response still exists. Therefore, standardizing in vitro testing posture based on the neutral zone range accounts for individual structural differences and facilitates comparisons across studies, evaluations of orthopaedic devices or clinical interventions.
The neutral zone range can be identified experimentally using the applied moment in the sagittal plane about the mediolateral axis and the resulting measured angular displacement that is sampled during passive range of motion pure moment (PM) tests. Increasing the magnitude of axial compressive force during this test has been shown to decrease a FSU’s range of motion in the sagittal plane and increase its passive rotational stiffness (Janevic, Ashton-Miller, & Schultz, Citation1991; Tawackoli, Marco, & Liebschner, Citation2004; Wilke, Wolf, Claes, Arand, & Wiesend, Citation1995. The range of motion decreased non-linearly as the compressive follower-load increased from 75 to 975 N (Tawackoli et al., Citation2004). Decreases in neutral zone was found to be higher in flexion (93%) in comparison to extension (85%) when five pairs of symmetrical muscle forces (80 N per pair) were applied (Wilke et al., Citation1995). Reductions in flexibility of functional spine units within the range of motion have been observed at higher loads; flexibility decreased by two- and threefold when the compressive force was increased from 0 to 2,200 and 4,400 N, respectively (Janevic et al., Citation1991). There was a linear relationship between an increase in compressive force magnitude tested in this study and a decrease in flexibility within the range of angular displacements. However, it remains unclear whether these relationships would hold within the neutral zone and the range of physiological loading (10–1,800 N) and the impact changing load would have on the determination of testing ranges. As such, it may be difficult to draw meaningful comparisons between in vitro studies where posture is an independent experimental factor if this compressive force was not standardized during testing range determination.
Therefore, the purpose of this study was to examine the impact of compressive force magnitude on an FSU’s neutral zone, as measured during PM tests. Considering previous research, it was hypothesized that increasing the magnitude of applied compressive force would: (i) significantly alter the neutral zone range (Janevic et al., Citation1991; Wilke et al., Citation1995), thus changing flexion and extension limits (Tawackoli et al., Citation2004) and (ii) significantly impact a FSU’s passive stiffness within this range (Tawackoli et al., Citation2004).
2. Methods
2.1. Specimen preparation and instrumentation
Thirty-three porcine cervical FSUs (19 c34, 14 c56; mean age ~6 months), each consisting of two adjacent vertebrae, the intervening intervertebral disc (IVD) and surrounding ligaments, were included in this study. Porcine cervical FSUs were used as a surrogate for the human lumbar spine, as previous work has shown that they have similar anatomical and functional structures, while providing superior experimental control over potential confounding factors that can impact the integrity of the tissues surrounding the IVD (e.g. age, diet, and activity level) (Yingling, Callaghan, & McGill, Citation1999). Anatomically, porcine cervical vertebrae have similar ligamentous structures and facet joint orientation as that of humans, except that porcine spines have non-load-bearing anterior processes (Oxland, Panjabi, Southern, & Duranceau, Citation1991; Yingling et al., Citation1999). Geometrically, the porcine vertebrae are approximately one third smaller in all dimensions (Yingling et al., Citation1999); therefore, the components making up the intervertebral disc fall in the lower range of human discs (Tampier, Citation2006). Further, they have similar range of motion and stiffness values in compression and shear (Wilke, Geppert, & Kienle, Citation2011; Yingling et al., Citation1999), justifying the use of porcine cervical spine as a surrogate of the human lumbar spine, especially for methodological investigations examining responses to mechanical exposures, where transfer of magnitudes for human exposure are not the desired outcomes. The specimens were obtained immediately following death and stored at −20°C. Prior to testing, frozen specimens were thawed at room temperature for approximately 12 h. All FSUs met a non-degenerated disc quality (Grade 1) as outlined by Galante (Citation1967). The musculature surrounding the IVD was removed from each specimen, leaving only the osteoligamentous structures intact, which was then fixed in custom-machined aluminium cup using a combination of 18-gauge stainless steel wire, 1" screws (inserted into the exposed superior and inferior endplates), and non-exothermic dental plaster (Denstone®, Miles, South Bend, IN, USA) (Aultman et al., Citation2005; Callaghan & McGill, Citation2001; Gooyers et al., Citation2012; Gunning et al., Citation2001; Yingling et al., Citation1999). A 0.9% saline misted solution was applied to each specimen every 20 min to prevent dehydration during the experiment.
2.2. PM test protocol
Each FSU received four repeats of a PM test with varying magnitudes of applied compressive force (10, 300, 900 and 1800 N) presented in a randomized order. This wide range of load was chosen to represent the physiological loading because it has been shown that up to approximately 2000 N of compressive force may be experienced during daily activities and light manual labour (Nachemson, Citation1981; Sato, Kikuchi, & Yonezawa, Citation1999), therefore, representing the physiological loading. The compressive force was applied in load control using a servo-hydraulic materials testing system (8872, Instron, Canton, MA), and a pure-moment in the sagittal plane was applied simultaneously in displacement control using an independent brushless servomotor (AKM23D, Kollmorgen/Danaher Motion, Radford, VA) connected in series with a torque cell (T120-106-1K, SensorData Technologies, Sterling heights, MI). This testing apparatus was designed to allow the centre of rotation to be aligned (vertically and horizontally) with the geometric centre of the IVD. Each specimen was free to translate in the anterior–posterior and mediolateral directions (via a bearing surface), which enabled the centre of rotation to translate within the joint during the cyclic loading protocol (Figure ). Since the stiffness of a specimen was expected to increase with increases in compressive force, the sagittal plane angle was used as the flexion–extension loading range. On average, the range of motion that a specimen went through was 24.8° (SD 6.10). The range of moment was varied across the magnitude of applied compressive force; that is, at 10, 300, 900 and 1,800 N, approximately ± 3, ± 7, ± 12 and ± 18 Nm was applied, respectively. The 10 N testing condition was intended to simulate an unloaded condition to ensure that the FSU remained under minimal compression. It is also important to note that the magnitude of the applied compressive force is not the load experienced across the joint (except in a neutral position), as this force is partitioned into compressive and shear components through the carriage system designed for the modified materials testing system.
Prior to collecting the PM tests, a 300 N static compressive force was applied to all FSUs for 15 min to reduce post-mortem swelling in the IVD (Aultman et al., Citation2005; Callaghan & McGill, Citation2001; Gooyers et al., Citation2012; Gunning et al., Citation2001; Yingling et al., Citation1999). During this preload test, the angular position of minimal passive stiffness was identified in the sagittal plane using moment angle data that were sampled from an independent servomotor (AKM23D; Danaher Motion, Radford, VA, USA) connected in series with a torque cell (T120-106-1K; SensorData Technologies Inc., Sterling Heights, MI, USA). This position was used as the FSU’s neutral position (i.e. 0°). Following the preload test, four repeats of the PM test were collected, each consisting of three cycles of passive flexion–extension applied at 0.5 degree/s (Figure ), while the applied moment (Nm) and angular displacement (degrees) were sampled at 15 Hz. Fifteen minutes of unloaded rest (i.e. 0 N) was provided between each PM test condition.
2.3. Data analysis
Four dependent measures were compared across loading conditions: (i) the neutral zone range (i.e. flexion limit–extension limit; degrees), (ii) the neutral zone passive stiffness (Nm/degree), as well as the angular displacement measured at the (iii) flexion and (iv) extension neutral zone limits (degrees). To establish the neutral zone range, the first derivative was taken from a fourth-order polynomial fit to the moment angle data sampled during each PM test, and the range between ± 0.05 Nm/degree from the minimum point was used to indicate limits (Thompson, Barker, & Pearcy, Citation2003) (Figure ). In addition to the neutral zone range, the limits defining the neutral zone (flexion and extension limits) were examined. The passive rotational stiffness within the neutral zone was described using the slope of a linear fit to the moment angle data within the boundaries that defined the neutral zone. Only the last two cycles applied in each PM test were considered for analysis, as previous research has shown reduced variability between the second and third cycles (Wilke, Wenger, & Claes, Citation1998).
Figure 3. Defining neutral zone range using a method of fitting a fourth-order polynomial curve to the ascending and descending curve (solid line) to the raw data (thin line), and first derivative of the fitted curve (dotted line) was calculated (Thompson et al., Citation2003). The neutral zone range was defined by the angle where it reached ± 0.05 Nm/degree (flexion and extension limit defined).
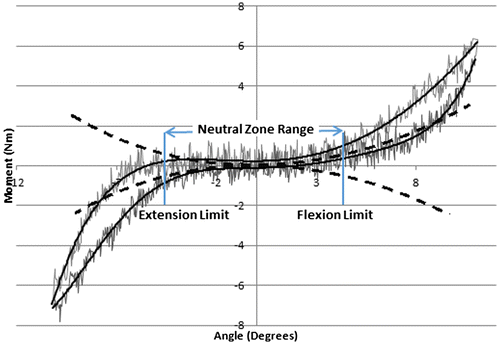
2.4. Statistical analysis
All statistical analyses were performed using IBM SPSS Statistics Version 20 (SPSS Inc., Chicago, IL, USA), with a significance level (α) of 0.05 determined a priori. Dependent measures were collapsed across specimen levels (i.e. c34, c56) for analysis due to the small effect size that existed in the neutral zone range (Cohen’s d = 0.49). Mauchly’s test revealed a violation of sphericity; therefore, the degrees of freedom were corrected for this deviance using the Greenhouse–Geisser method. To examine the impact of compressive force magnitude, a one-way repeated measures, general linear model was used for each dependent measure. Effect sizes for all significant comparisons were evaluated using Cohen’s d, computed using the sample mean and the standard deviation. Pearson’s correlation coefficient (r) was also calculated over averaged data to assess the linearity of the relationship between the magnitude of compressive force and the neutral zone range and stiffness.
3. Results
3.1. Neutral zone range and passive stiffness
Increasing the magnitude of the applied compressive force significantly decreased the FSU neutral zone range from 7.21° (SE 0.41) at 10 N to 2.80° (SE 0.15) at 1,800 N (F(1.51, 46.93) = 113.24; p < 0.001), while increasing the passive stiffness from 0.03 Nm/degree (SE 0.003) at 10 N to 0.41 Nm/degree (SE 0.02) at 1,800 N (F(1.25, 40.09) = 210.84; p < 0.001) (Table ). Specifically, the neutral zone range was reduced by 64 (d = 7.69), 71 (d = 6.70) and 85% (d = 3.25) when the magnitude of compressive force was increased from 10 N to 300 N; from 300 N to 900 N; and from 900 N to 1,800 N, respectively (pairwise p < 0.01). The correlation between the magnitude of compressive force and neutral zone range was −0.67, which indicated a reduction in range with increasing force. Moreover, the neutral zone passive stiffness increased two- to threefold at each compressive force magnitude increase (pairwise p < 0.01; d = 11.00–27.51). An increase in the magnitude of compressive force resulted in an increase in the neutral zone passive stiffness with a correlation coefficient of 0.89.
Table 1. Mean (SE) neutral zone range, passive stiffness and flexion/extension limits (n = 33)
3.2. Neutral zone flexion and extension limit
The neutral zone flexion limit with 10 N of applied compressive force was significantly different from all other loading conditions (p < 0.05; d = 1.19); however, there were no significant differences between the higher compressive forces examined (Table ). On average, the difference between flexion limit at 10 N, compared to all other test conditions (i.e. 300, 900, 1,800 N) was below 2°, where the 1,800 N condition showed the largest mean change of + 1.56° (SE 1.61). The extension limit was significantly different at each magnitude of compressive force (p < 0.005; d = 1.12–6.73), with relative changes of approximately 4–6° (Table ) compared to the 10 N condition.
4. Discussion
Based on the results of this study, it is recommended that the magnitude of compressive force be standardized across in vitro biomechanics studies when conducting PM tests. The neutral zone range and passive stiffness were significantly altered across each magnitude of compressive force examined. To the best of the authors’ knowledge, this is one of the first studies to show that the neutral zone range and passive rotational stiffness are significantly impacted by the magnitudes of applied compressive force during PM range of motion tests. Contrary to our hypothesis, there was minimal change observed in the neutral zone flexion limit across loading conditions. The extension limit significantly shifted towards the neutral angle as the compressive force increased, which may be due to an increase in the facet joint contact area (Panjabi, Krag, White, & Southwick, Citation1977), resulting in an increase in joint rotational stiffness and reduction in neutral zone range. These findings reinforce the need for a standardized protocol for the magnitude of applied compressive force during PM tests, particularly when using the neutral zone range to standardize FSU testing postures.
Findings from this work support the second hypothesis that the neutral zone range would significantly decrease as the applied compressive force increased. This is in agreement with previous work that has shown reduced passive range of motion under increased compressive forces (Janevic et al., Citation1991; Tawackoli et al., Citation2004; Wilke et al., Citation1995). A reduction of approximately 90% in sagittal plane range of motion was found when five pairs (80 N per pair) of surrogate symmetrical muscle forces were applied (Wilke et al., Citation1995). This study also showed a linear, inverse relationship between the compressive force and neutral zone range over a range of physiological loading, indicating that the effect that the magnitude of compressive force has on the neutral zone range among studies can be estimated (neutral zone range and corresponding postures would be comparable between studies as long as the compressive force was reported). Although the mode of force application was different in the study conducted by Wilke et al. (Citation1995), a significant reduction in neutral zone range was noted with compressive force magnitudes under 300 N, which is similar to the findings observed in this experiment. These significant differences noted in the lower magnitudes of compressive force (between 10 and 300 N) reinforce the notion that neutral zone range is sensitive to the magnitude of compressive force applied.
In accordance with previous research, neutral zone passive stiffness significantly increased between the 10 and 1,800 N loading conditions (Janevic et al., Citation1991). The stiffness increased by two to three times at lower magnitudes of compressive force. On the other hand, the neutral zone stiffness increase from 10 N to the higher magnitudes of compressive force was much greater (i.e. approximately 2×) in this study, although direct comparison with studies conducted using the follower-load (Patwardhan et al., Citation2003) or examining stiffness over a range of motion (Janevic et al., Citation1991) may not be appropriate. This difference may be attributed to the condition of specimens used, particularly the intervertebral disc. Previous research has attributed an increase in passive stiffness to an increase in facet joint contact (Panjabi et al., Citation1977) and/or an increase in the intradiscal pressure (Janevic et al., Citation1991; Panjabi et al., Citation1977), which has been shown to increase linearly as the applied load increases (Adams, McNally, & Dolan, Citation1996; Nachemson, Citation1966; Wilke et al., Citation1999). Since a strong linear increase in passive stiffness was also observed in this study, it can be hypothesized that the intradiscal pressure may play a dominant role in increasing the stiffness within the neutral zone. The difference in intradiscal pressure response can be caused by the choice of specimens; in this study, healthy, intact porcine spines were used, whereas prior studies have used cadaveric spines (Janevic et al., Citation1991), in which age-related degeneration in the nucleus pulposus could have impacted the intradiscal pressure responses (Yang et al., Citation2010).
Posture is an important mechanical factor to consider when assessing the risk of injury (Adams et al., Citation2000, Citation1993; Adams & Hutton, Citation1981; Aultman et al., Citation2005; Ayturk et al., Citation2010; Callaghan & McGill, Citation2001; Gooyers et al., Citation2012; Gunning et al., Citation2001; Veres et al., Citation2009; Wilke et al., Citation1999). Contrary to our hypothesis, the neutral zone flexion limit was not significantly different above 300 N of compressive force. In fact, when collapsed across all loading conditions, the neutral zone flexion limit varied less than 2°. The extension limit significantly shifted towards the neutral posture (decreased range of motion) as the magnitude of compressive force increased. This shift may have occurred due to the increased amount of facet joint contact (Panjabi et al., Citation1977), which would translate inferior vertebra anteriorly, when rotating the FSU into extension. This hypothesis is supported by previous work suggesting that the instantaneous centre of rotation of a FSU may change depending on the posture (Patwardhan et al., Citation2003). Therefore, reporting the magnitude of compressive force during PM tests is important when posture is a standardized experimental factor considered in the study design and would impact the testing range selected for the experimental assessments. In particular, the compressive force should be carefully chosen to reflect the purpose of the experimental study—for instance, if the reference posture used in the experiment represents the intervertebral disc neutral zone, a lower magnitude of compressive force (< 300 N) should be used to define the passive range of motion in order to minimize the contribution from other structures, including the facet joints.
There are several limitations to this study. First, only four magnitudes of compressive force were tested. Although the Pearson correlation coefficient demonstrated moderate-to-strong effects (r > 0.50) for both the neutral zone range and stiffness data, it may not be appropriate to extrapolate this trend to greater magnitudes of compressive force. Second, some of the noted differences compared to previous work may have been caused by dissimilarities in the test instrumentation (Cripton, Bruehlmann, Orr, Oxland, & Nolte, Citation2000). The test system used in the present work enabled the FSU to move in five degrees of freedom (joint lateral bending was constrained), which has shown on average to increase angular displacement in flexion compared to other test systems (e.g. follower-load apparatus), where the applied force is directed through the balance point of the FSU (Cripton et al., Citation2000). This instrumentation could have induced artifact moments when the specimen deviated from its neutral posture. These artifact moments induced from the compression force would be measured by torque cell and would not have impacted the neutral zone magnitudes as the calculation method was based on the shape of the moment angle curves and not the magnitude of the moments. Finally, given that there are many definitions and methods of identifying neutral zone range (Howarth et al., Citation2013; Panjabi, Citation1992; Smit, van Tunen, van der Veen, Kingma, & van Dieën, Citation2011; Thompson et al., Citation2003; Wilke et al., Citation1998), the results may be significantly affected by our choice of analysis. The method used by Thompson et al. (Citation2003) was chosen based on the flexibility to fit a curve to the data, whereas the sigmoidal curve used by Smit et al. (Citation2011) did not fit to the data at higher compressive forces. For the purpose of examining the changes in neutral zone range and stiffness, the analysis method used in this study was consistent across compressive forces, objective in selecting the end points and therefore considered appropriate.
The present work supported the hypotheses that neutral zone range and stiffness would be significantly altered by the magnitude of compressive force. The neutral zone flexion limit was not significantly impacted over 300 N of compressive force, while the extension limit varied across each of the four magnitudes (i.e. 10, 300, 900, 1,800 N) of compressive forces tested. Therefore, the results of this work suggest that the magnitude of compressive force should be standardized when conducting PM tests, or at the very least explicitly stated in the methodology section, to allow for meaningful comparisons of the effect of test posture across studies.
Additional information
Funding
Notes on contributors
Jack P. Callaghan
Jack P. Callaghan’s current research is focused on establishing a relationship between cumulative loading and low-back pain, which is important for setting occupational exposure limits and preventing low-back injuries. His research combines a fundamental in vitro research approach, examining the time-varying response of the lumbar spine tissues, with in vivo human research approach, examining biological responses to cumulative loading exposure from both pain generating and tissue altering/injuring perspectives. The work presented in this paper directly impacts the study methods for in vitro studies, since posture in relation to its neutral zone is an important factor to consider when examining the time-varying mechanical response of lumbar spine tissues. This work highlights the considerations that need to be given to posture standardization, since results from in vitro studies could influence the magnitude of exposure limits.
References
- Adams, M. A., Freeman, B. J., Morrison, H. P., Nelson, I. W., & Dolan, P. (2000). Mechanical initiation of intervertebral disc degeneration. Spine, 25, 1625–1636. doi:10.1097/00007632-200007010-00005
- Adams, M. A., & Hutton, W. C. (1981). The effect of posture on the strength of the lumbar spine. ARCHIVE: Engineering in Medicine 1971-1988 (vols 1-17), 10, 199–202.10.1243/EMED_JOUR_1981_010_053_02
- Adams, M. A., McNally, D. S., & Dolan, P. (1996). ‘Stress’ distributions inside intervertebral discs. The Journal of Bone and Joint Surgery, 78, 965–972.10.1302/0301-620X78B6.1287
- Adams, M. A., McNally, D. S., Wagstaff, J., & Goodship, A. E. (1993). Abnormal stress concentrations in lumbar intervertebral discs following damage to the vertebral bodies: A cause of disc failure? European Spine Journal, 1, 214–221.10.1007/BF00298362
- Aultman, C. D., Scannell, J., & McGill, S. M. (2005). The direction of progressive herniation in porcine spine motion segments is influenced by the orientation of the bending axis. Clinical Biomechanics, 20, 126–129.10.1016/j.clinbiomech.2004.09.010
- Ayturk, U. M., Garcia, J. J., & Puttlitz, C. M. (2010). The micromechanical role of the annulus fibrosus components under physiological loading of the lumbar spine. Journal of Biomechanical Engineering, 132, 061007. doi:10.1115/1.4001032
- Balkovec, C., & McGill, S. (2012). Extent of nucleus pulposus migration in the annulus of porcine intervertebral discs exposed to cyclic flexion only versus cyclic flexion and extension. Clinical Biomechanics, 27, 766–770. doi:10.1016/j.clinbiomech.2012.05.006
- Callaghan, J. P., & McGill, S. M. (2001). Intervertebral disc herniation: Studies on a porcine model exposed to highly repetitive flexion/extension motion with compressive force. Clinical Biomechanics, 16, 28–37.10.1016/S0268-0033(00)00063-2
- Cripton, P. A., Bruehlmann, S. B., Orr, T. E., Oxland, T. R., & Nolte, L. P. (2000). In vitro axial preload application during spine flexibility testing: Towards reduced apparatus-related artefacts. Journal of Biomechanics, 33, 1559–1568.10.1016/S0021-9290(00)00145-7
- DePalma, M. J., Ketchum, J. M., & Saullo, T. (2011). What is the source of chronic low back pain and does age play a role? Pain Medicine, 12, 224–233. doi:10.1111/j.1526-4637.2010.01045.x
- Galante, J. O. (1967). Tensile properties of the human lumbar annulus fibrosus. Acta Orthopaedica, 38, 1–91.10.3109/ort.1967.38.suppl-100.01
- Gooyers, C. E., McMillan, R. D., Howarth, S. J., & Callaghan, J. P. (2012). The impact of posture and prolonged cyclic compressive loading on vertebral joint mechanics. Spine,. doi:10.1097/BRS.0b013e318256f9e6
- Gunning, J. L., Callaghan, J. P., & McGill, S. M. (2001). Spinal posture and prior loading history modulate compressive strength and type of failure in the spine: A biomechanical study using a porcine cervical spine model. Clinical Biomechanics, 16, 471–480.10.1016/S0268-0033(01)00032-8
- Howarth, S. J., Gallagher, K. M., & Callaghan, J. P. (2013). Postural influence on the neutral zone of the porcine cervical spine under anterior-posterior shear load. Medical Engineering and Physics, 35, 910–918.10.1016/j.medengphy.2012.08.019
- Janevic, J., Ashton-Miller, J. A., & Schultz, A. B. (1991). Large compressive preloads decrease lumbar motion segment flexibility. Journal of Orthopaedic Research, 9, 228–236. doi:10.1016/0021-9290(90)90212-L
- Nachemson, A. (1966). The load on lumbar disks in different positions of the body. Clinical Orthopaedics and Related Research, 45, 107–122.
- Nachemson, A. (1981). Disc pressure measurements. Spine, 6, 93–97.10.1097/00007632-198101000-00020
- Oxland, T. R., Panjabi, M. M., Southern, E. P., & Duranceau, J. S. (1991). An anatomic basis for spinal instability: A porcine trauma model. Journal of Orthopaedic Research, 9, 452–462. doi:10.1002/jor.1100090318
- Panjabi, M. M. (1992). The stabilizing system of the spine. Part II. Neutral zone and instability hypothesis. Journal of Spinal Disorders, 5, 390–397, discussion 397. doi:10.1097/00002517-199212000-00002
- Panjabi, M. M., Krag, M. H., White, A. A., & Southwick, W. O. (1977). Effects of preload on load displacement curves of the lumbar spine. The Orthopedic Clinics of North America, 8, 181–192.
- Patwardhan, A. G., Havey, R. M., Carandang, G., Simonds, J., Voronov, L. I., Ghanayem, A. J., … Paxinos, O. (2003). Effect of compressive follower preload on the flexion-extension response of the human lumbar spine. Journal of Orthopaedic Research, 21, 540–546.10.1016/S0736-0266(02)00202-4
- Sato, K., Kikuchi, S., & Yonezawa, T. (1999). In vivo intradiscal pressure measurement in healthy individuals and in patients with ongoing back problems. Spine, 24, 2468–2474. doi:10.1097/00007632-199912010-00008
- Scannell, J. P., & McGill, S. M. (2003). Lumbar posture–should it, and can it, be modified? A study of passive tissue stiffness and lumbar position during activities of daily living. Physical Therapy, 83, 907–917.
- Schwarzer, A. C., Aprill, C. N., Derby, R., Fortin, J., Kine, G., & Bogduk, N. (1995). The prevalence and clinical features of internal disc disruption in patients with chronic low back pain. Spine, 20, 1878–1883.10.1097/00007632-199509000-00007
- Smit, T. H., van Tunen, M. S., van der Veen, A. J., Kingma, I., & van Dieën, J. H. (2011). Quantifying intervertebral disc mechanics: A new definition of the neutral zone. BMC Musculoskeletal Disorders, 12, 1–10.10.1186/1471-2474-12-38
- Tampier, C. (2006). Progressive disc herniation: An investigation of the mechanism using histochemical and microscopic techniques (Master’s thesis). University of Waterloo, Waterloo.
- Tawackoli, W., Marco, R., & Liebschner, M. A. K. (2004). The effect of compressive axial preload on the flexibility of the thoracolumbar spine. Spine, 29, 988–993. doi:10.1097/00007632-200405010-00007
- Thompson, R. E., Barker, T. M., & Pearcy, M. J. (2003). Defining the neutral zone of sheep intervertebral joints during dynamic motions: An in vitro study. Clinical Biomechanics, 18, 89–98.10.1016/S0268-0033(02)00180-8
- Veres, S. P., Robertson, P. A., & Broom, N. D. (2009). The morphology of acute disc herniation. Spine, 34, 2288–2296. doi:10.1097/BRS.0b013e3181a49d7e
- Wilke, H. J., Neef, P., Caimi, M., Hoogland, T., & Claes, L. E. (1999). New in vivo measurements of pressures in the intervertebral disc in daily life. Spine, 24, 755–762. doi:10.1097/00007632-199904150-00005
- Wilke, H. J., Wenger, K., & Claes, L. (1998). Testing criteria for spinal implants: Recommendations for the standardization of in vitro stability testing of spinal implants. European Spine Journal, 7, 148–154. doi:10.1007/s005860050045
- Wilke, H. J., Wolf, S., Claes, L. E., Arand, M., & Wiesend, A. (1995). Stability increase of the lumbar spine with different muscle groups. A biomechanical in vitro study. Spine, 20, 192–198. doi:10.1097/00007632-199501150-00011
- Wilke, H.-J., Geppert, J., & Kienle, A. (2011). Biomechanical in vitro evaluation of the complete porcine spine in comparison with data of the human spine. European Spine Journal, 20, 1859–1868. doi:10.1007/s00586-011-1822-6
- Yang, S.-H., Lin, C.-C., Hu, M.-H., Shih, T. T.-F., Sun, Y.-H., & Lin, F.-H. (2010). Influence of age-related degeneration on regenerative potential of human nucleus pulposus cells. Journal of Orthopaedic Research: Official Publication of the Orthopaedic Research Society, 28, 379–383. doi:10.1002/jor.20988
- Yingling, V. R., Callaghan, J. P., & McGill, S. M. (1999). The porcine cervical spine as a model of the human lumbar spine: An anatomical, geometric, and functional comparison. Journal of Spinal Disorders, 12, 415–423.10.1097/00002517-199912050-00012