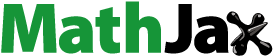
Abstract
A compilation of magnetocaloric properties of metallic nanostructures with Curie temperature (TC) between 260 and 340 K has been tabulated. The tabulated data show that nanostructure plays an important role in enhancing the magnetocaloric properties of a material, namely by reducing the peak of magnetic entropy, but broadening of the magnetocaloric effect curve with an average of 10 K sliding window for Curie temperature. A second table lists all bulk metallic and intermetallic materials, in which there is no nanostructural data, with an entropy change of at least 20 J/kg K and a Curie temperature between 260 and 340 K. We propose that further experiments should be made on the nanostructured form of these materials.
Public Interest Statement
In contrast with conventional refrigerator systems that work based on compression and evaporation of gas, magnetic refrigeration systems work based on magnetizing and demagnetizing a magnetic material. Magnetic refrigeration is an energy-efficient and environmentally friendly refrigeration technology with the following desirable characteristics: (1) it does not use ozone-depleting and hazardous chemicals or greenhouse gases; (2) the cooling efficiency of magnetic refrigeration is significantly higher than conventional cooling technology, compressor-based techniques; and (3) the magnetic refrigerator can be built more compactly and generates less noise. Magnetic refrigeration exploits a property of magnetic materials called the magnetocaloric effect (MCE). A through literature survey has been done in this article and it has been concluded that metallic nanostructures are the best materials to be used in a magnetic refrigeration system compared to their bulk counterparts. Moreover, this paper suggests some new materials to be synthesized as nanostructures and their MCE properties studied.
1. Introduction
Magnetic refrigeration technology, as a promising alternative to conventional gas compression cooling, has attracted extensive attention due to its good energy efficiency, and environmentally friendly nature. Materials with Curie temperature near room temperature with good magnetocaloric effect (MCE) properties and negligible hysteresis are of special interest.
Particle size, shape, morphology, chemical composition, structure, and interaction of the particle with the surrounding matrix and neighboring particles all have a profound effect on the magnetic behavior of the material. Any material with discrete functional parts, with any internal, external, or surface structure dimension of the order of 100 nm or less is classified as nanostructured material. Nanostructures can be synthesized in different shapes such as nanowires, nanotubes, etc. Differentiation between the numbers of dimensions on the nanoscale describes a nanostructured material. It has been shown that nanostructures are the best choice for magnetic refrigeration systems (Bennett et al., Citation1995; Della Torre, Bennett, & Jin, Citation2012; Shir, Yanik, Bennett, Della Torre, & Shull, Citation2003; Shull, Swartzendruber, & Bennett, Citation1991). Nanostructures have a higher MCE over a temperature distribution so they exhibit more cooling efficiency as compared to bulk materials. The entropy change ΔS, determines the cooling efficiency and it is largest at lower fields. The magnitude of entropy change varies for different materials based on the number of particles per unit volume, magnetic moment of the particles, and the order parameter (Shir et al., Citation2003). An example of this can be seen in Figures and for gadolinium. Magnetocaloric properties of a nanostructure can be tuned and optimized by controlling its properties such as size, shape, morphology, etc. Therefore, the MCE peak can be displaced to other temperatures, broadened, or made sharper. This point has been amplified, by gathering all nanostructures with Curie temperature in the range of 260–340 K in Table and comparing their MCE properties to their bulk counterparts for materials available in the literature. Furthermore, all bulk materials reported in the literature with magnetic entropy of at least 20 J/kg K and a Curie temperature between 260 and 340 K have been arranged in Table . This list is of importance for future research since there are no data available for the nanostructurized form of these bulk materials.
Figure 1. Calculated change in entropy, |∆Sm|, of nanocomposite clusters of Gd and Gd alloys between 0 and 2T vs. temperature.
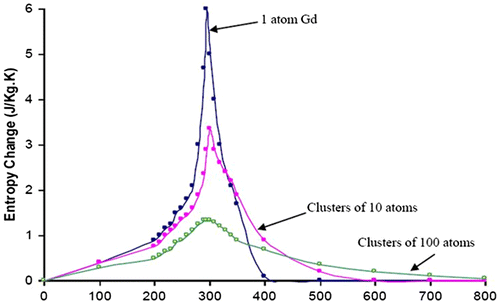
Figure 2. MCE (∆T) vs. temperature for the operating lines: ideal Gd bulk material and a calculated Gd nanocomposite (Shir et al., Citation2003).
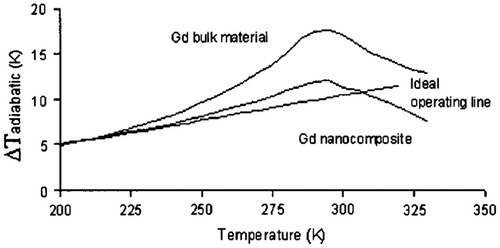
Table 1. Studied nanostructured materials and their bulk counterparts
Table 2. Metallic and intermetallic bulk materials with magnetic entropy of at least 20 J/kg K
Though much research has been done on employing oxide nanostructures, oxide nanostructures have less magnetization and will not be able to compete with metallic nanostructures. Therefore, metallic and intermetallic nanostructures seem to be the best choice for magnetic refrigeration systems.
2. Methodology
Nanostructures have several advantages as a refrigerant for 100–300-K applications compared to the other common methods of assembling a magnetic refrigeration bed, such as a layered thermal bed (Shir et al., Citation2003) or mixing of different magnetic materials. In order to predict and evaluate the MCE of a nanostructure, refrigerant capacity can be considered as the basis for calculating the temperature behavior of the material. The relative cooling power (RCP) is defined as(1)
(1)
where ∆SM (T, H) is the refrigerant’s magnetic entropy change as a function of temperature and magnetic field and δTFWHM is the full width at half maximum (FWHM) of the peak of magnetic entropy. This parameter measures how much heat can be transferred between the cold and hot heat exchangers in an ideal refrigeration cycle (Gschneidner, Pecharsky, Pecharsky, & Zimm, Citation1999; McMichael, Shull, Swartzendruber, Bennett, & Watson, Citation1992). Increasing the RCP not only increases the amount of refrigeration obtainable from the particular refrigerant and field excursion, but also tends to increase the thermodynamic efficiency of the cycle, thus measuring how well a particular volume or quantity of refrigerant is utilized. Improvement in RCP mainly relies on broadening the magnetic entropy change by either coupling two phases of magnetic materials with desirable properties or nanostructure synthesis with the main motivation rooted in their inherent tendency to have distributed exchange coupling, which will broaden the magnetic entropy curve (Ucar, Ipus, France, Mchenry, & Laughlin, Citation2012).
The sum rule (McMichael et al., Citation1992) implies that for materials with the same saturation magnetic moments, Mo, those with high entropy change at a given temperature will have low entropy changes at other temperatures, and materials which do not have a large entropy change at any particular temperature can undergo a moderate entropy change over a broader temperature range. Magnetization of suitable nanostructures can be switched by an applied magnetic field of the order 1 T (10 kOe) or less, which is easily realized without using superconducting magnet (Skomski, Binek, Mukherjee, Sahoo, & Sellmyer, Citation2008).
The need for obtaining materials with a desired entropy change leads to the need for accurate modeling and simulation of entropy change for materials. Work by Bennett, McMichael, Tang, and Watson (Citation1994) and Bennett, McMichael, Swartzendruber, and Shull (Citation1992) shows how Monte Carlo simulations can be used to predict entropy change for a nanostructure with ferromagnetically interacting clusters. Results in McMichael et al. (Citation1992) and Bennett et al. (Citation1992) show that magnetic nanostructures can possess enhanced magnetocaloric properties at high temperatures and low fields. Materials that rely on nanostructures include paramagnetic salts used for attaining low temperatures and superparamagnetic particles for intermediate temperatures, while on ferromagnetic to paramagnetic or magnetostructural phase transformation at room temperature (Ucar et al., Citation2012).
Work by Shir et al. (Citation2003) emphasizes that magnetic nanostructures with interacting clusters are a means by which to readjust magnetic entropy (∆S) to make it more uniform with the temperature and to provide enhanced magnetic entropy (∆S) values at temperatures above their effective interaction temperature. In addition, they revealed that by appropriate selection of nanostructure materials, MCE vs. T curve can be fine-tuned to a specific curve as shown in Figures and . Magnetic entropy (ΔS) constancy can be fulfilled and in the reversible case, the cycle will have an efficiency equivalent to the Carnot cycle.
Work by Shull (Citation1993) shows experimental and theoretical verifications of the enhancement of magnetocaloric properties of a superparamagnetic nanostructure material Gd3Ga5−xFexO12 (GGIG) at high temperatures and low fields. In addition, the MCEs were found to be 3–4 times larger than a low temperature paramagnetic refrigerant (GGG).
Nanostructuring improves the entropy change in low fields and high temperatures, but yields some reduction in the maximum entropy change per atom. An important feature of magnetic nanoparticles is that the entropy exhibits a strong dependence on the magnetic anisotropy which can be exploited for magnetic cooling (Skomski et al., Citation2008). In principle, anisotropy of nanostructures can be tailored almost at will, being possible to control not only its magnitude but also the distribution of easy axes orientation, leading to MCE response that is qualitatively different to that of the bulk material (Franco et al., Citation2008). Inter-particle and intra-particle interactions modify the magnetic responses for an arrangement of nanoparticles such as anisotropy energy (Skomski et al., Citation2008), Zeeman energy, and dipolar interaction energy. The total energy is given by(2)
(2)
in which, the anisotropy and Zeeman energy terms are and
, respectively. The magnetic dipole energy between two particles i and j, separated by a distance rij, with magnetic moments μi and μj, and the volume corresponding to the ith particle Vi, is
.
Inter-particle interactions’ strength exhibits three magnetic stages: (1) Superparamagnetism (SPM) for no interaction, (2) Superspin glass (SSG) for intermediate interaction strengths, and (3) Superferromagnetism for highly interacting superspins (Bedanta & Kleemann, Citation2009). Detailed studies on the effect of different parameters e.g. particle size, inter-particle distance, magnetic field, and temperature on the above states are discussed in Chen, Sahoo, Kleemann, Cardoso, and Freitas (Citation2004) and Petracic et al. (Citation2006).
3. Sample preparation
Properties of materials, in general, and nanoparticles, in particular, are influenced by shape, size, and other parameters controlled by sample preparation. Hence, a special interest in the field of sample preparation. Broadly techniques can be classified into top-down or bottom-up approaches as shown in Figure .
Many current strategies combine both synthesis and assembly into a single process (Gubin, Koksharov, Khomutov, & Yurkov, Citation2005; Saravanan, Gopalan, & Chandrasekaran, Citation2008). Some of these techniques are
• | High-temperature Thermal Decomposition which is suitable for preparing films of nanoparticles (Saravanan et al., Citation2008). | ||||
• | Gas–liquid Interface for the synthesis of metallic nanoparticles in the absence of solid substrates or matrices by oxidation–reduction (redox) reactions at an interface between two phases, one containing a metal compound and the other, the reducing agent (Saravanan et al., Citation2008). | ||||
• | Electrolytic erosion and electrochemical generation are examples of Mechanochemical dispersion, which is an attractive way to produce disperse systems with the size of the particles depending upon the density of the electric current (Saravanan et al., Citation2008). | ||||
• | Liquid–liquid Interface Reaction not only synthesizes nanoparticles but also casts them into a film in one process (Gubin et al., Citation2005). | ||||
• | Vaporization (laser, thermal, plasma, arch discharge, and solar energy-induced) of supersaturated metal allows to study physiochemical characteristics and properties at the gas and solidified phases (Gubin et al., Citation2005). | ||||
• | Thermolysis or Thermal decomposition of metal-containing compounds is carried out in a liquid medium in the presence of surfactants or polymers resulting in nanoparticles with diameters up to 10 nm (Gubin et al., Citation2005). | ||||
• | Decomposition of metal-containing compounds by ultrasonic treatment is carried out in metal carbonyls and their derivatives being used as metal-containing compounds (Gubin et al., Citation2005). | ||||
• | Using strong reducing agents e.g. alkali metal dispersions in ethers or hydrocarbons, alkali metal complexes with organic electro acceptors or high-boiling alcohols, and magnetic metallic nanoparticles can be prepared by the Reduction method (Gubin et al., Citation2005). | ||||
• | Sol–gel method, though mostly used to obtain metal oxides, is being used for the synthesis of nanosized metals (Gubin et al., Citation2005). | ||||
• | High-energy ball milling is a widely used technique for synthesizing new metastable alloys out from the thermodynamic equilibrium such as amorphous metallic glasses or disordered and supersaturated solid solutions with achieving a size of below 10 nm (Michalski et al., Citation2012; Ucar, Ipus, Laughlin, & McHenry, Citation2013). |
Due to sensitivity of nanostructures to milling intensity, temperature and other factors have far-reaching effects on their properties (Saravanan et al., Citation2008).
4. Metallic nanostructure survey
Table demonstrates that nanostructures are a better choice compared to their bulk counterpart to be utilized in the magnetic refrigeration system. Even though the bulk material has a higher magnetic entropy change, the RCP of the nanostructure is higher than the bulk counterpart. This is very important in the magnetic refrigeration system since increasing the RCP not only increases the amount of refrigeration obtainable from the particular refrigerant and field excursion, but also tends to increase the thermodynamic efficiency of the cycle.
The reduction in magnetization for bulk Pr2Fe17 goes down sharply with temperatures above 280 K, while for the nanostructure Pr2Fe17, it decreases slowly. At 150 K, the magnetization is 50% greater in the bulk sample but under higher magnetic field, the situation is different, as seen in Figure (Gorria et al., Citation2008).
Figure 4. Temperature dependence of the magnetization for both bulk and BM-10h Pr2Fe17 alloys under an applied magnetic field of μ0H = 100 mT.
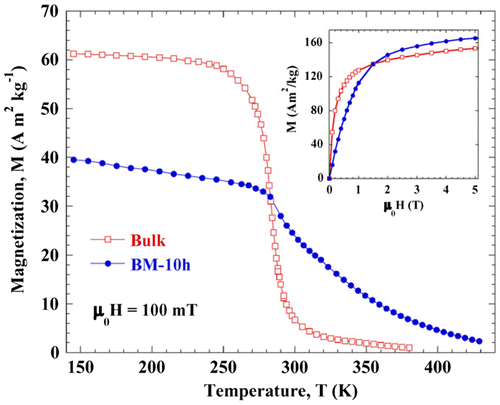
Figure shows the improvement in the RCP of a nanostructure compared to its bulk counterpart. Even though the magnetic entropy is higher in the bulk material, the nanostructure Pr2Fe17 has 60% higher δTFWHM compared to the bulk (Gorria et al., Citation2008).
Figure 5. Temperature dependence of calculated Δ|SM| and RCP values under an applied magnetic field, μ0H = 5 T, for pure Gd, bulk Pr2Fe17, and BM-10h Pr2Fe17.
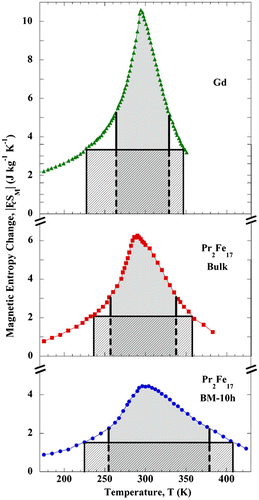
Three samples of Nd2Fe17 at different particle sizes practically show same MCE properties, but δTFWHM becomes broader with the increase in the milling time and hence reduction in the size. For nanostructure Nd2Fe17 sample, δTFWHM is 72, while for the bulk it is 50 (Alvarez, Gorria, et al., Citation2010; Alvarez, Sánchez-Marcos, et al., Citation2010) as shown in Figure . A slight improvement of about 5% (Alvarez, Gorria, et al., Citation2010) is observed in RCP, but in the case of Pr2Fe17, as seen in Table , the improvement in MCE is not that great in the applied magnetic field of 15 kOe.
Figure 6. Temperature dependence of Δ|SM| under H = 15 kOe for bulk and milled Nd2Fe17 samples.
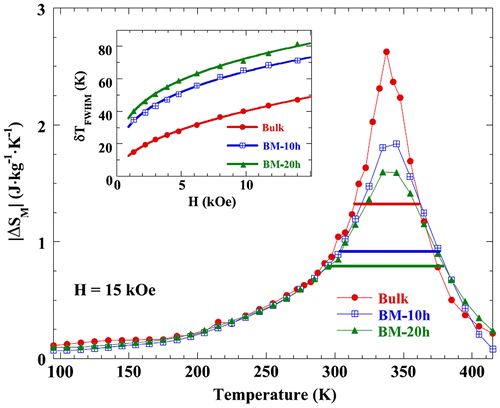
Curie temperature of Ni nanostructure decreases with the reduction in grain size with a dramatic drop below 10 nm, but its Curie temperature is still above room temperature. The Fe–Ni system, on the other hand, is a good economical and efficient alternative to rare earth-based alternatives. Fe–Ni-based alloys suffer from low magnetic entropy change, thus resulting in a low adiabatic temperature change. However, Fe–Ni-based alloys have low hysteresis and tunable RCP by alloying and breadth of the magnetic transition controlled by impurity- and disorder-derived distributed exchange interactions (Ucar, Craven, Laughlin, & Mchenry, Citation2014; Ucar et al., Citation2013).
As seen in Table (Ucar et al., Citation2013), even though the compositions of Fe72Ni28 and Fe70Ni30 are different, the same Curie temperature has been obtained by varying the milling time in preparing the material (50 and 10 h, respectively). Although TC of Fe70Ni30 when milled for 50 h is above room temperature, addition of Mo content can decrease it without decreasing TC due to broadening of MCE curve because of decrease in magnetic moment and spin-up electron density (Ucar et al., Citation2014). Alternatively nanoclusters, e.g. Co with size of 2 nm embedded in Ni–Cu matrix, are chosen because of the tenability of their Curie temperature by varying the copper concentration (Michalski et al., Citation2012). Work by Mukherjee, Sahoo, Skomski, Sellmyer, and Binek (Citation2009) shows compelling evidence of the benefits of Co/Cr superlattices through modeling and experimental data.
Work by Rong, Li, and Ping Liu (Citation2007) on FexPt100−x shows the strong effect of controlled composition on its Curie temperature. Moreover, ordered phases with different structures and their formation in different compositional regions are discussed. Ramasamy, Mazumdar, Bennett and Gupta (Citation2012) synthesized CuCr2Te4 by reducing tellurium with sodium borohydride in boiling trioctylamine yielding cube-shaped spinel nanocrystals that have room temperature TC. Work by Sun et al. (Citation2012) shows a reduction of 80% thermal and 60% magnetic hysteresis in MnAs0.97P0.03 because of 0.68% microstrain presence.
5. Suggestions for new nanostructures
Nanotechnology with its inherent flexibility of tailoring microscopic parameters unlike their bulk counterparts has its advantages (Mukherjee et al., Citation2009). Table lists metallic and intermetallic bulk materials with magnetic entropy of at least 20 J/kg K and Curie temperature in the range of 260–340 K. As observed in case of Table materials, it is anticipated that synthesizing these materials as nanostructures can achieve more than 40% improvements depending upon the composition of the materials.
6. Summary
MCE data have been gathered for all the metallic nanostructures with Curie temperature between 260 and 340 K in Table . When available, nanostructures were compared to their bulk counterparts to understand the effect of nanostructure on MCE properties. In all the cases observed, nanostructures have a profound positive effect on the MCE properties. Metallic nanostructures are important to be considered and further studied for use in magnetic refrigeration technology. In this regard, all metallic bulk materials with magnetic entropy of at least 20 J/kg K have been gathered in Table . As observed in the case of Table materials, when bulk materials are synthesized as nanostructures, an improvement of greater that 40% has been reported in their MCE properties. We propose Table materials be synthesized as nanostructures and their MCE properties studied.
Additional information
Funding
Notes on contributors
Amir Aslani
Authors are focusing their cutting-edge research at the Institute for Magnetics Research (IMR) of the George Washington University on modeling, experimental measurements, and the use of magnetic materials. The materials most commonly studied are magnetic recording media, magneto-optical media, magnetocaloric material, and magnetostrictive material. Some of the applications for such materials include computer hard drives, memories, microwave devices, magnetostrictive transducers, and magnetic refrigeration. Modeling methods employed include Preisach-based models, micromagnetics, and nonlinear finite element models. IMR has several well-equipped laboratories such as Magnetic Refrigeration Research Laboratory, Magnetic Material Testing Laboratory, and Magneto-Optics Laboratory. Past and present organizations that have cooperated with the Institute in conducting research include NIST, NSF, DOE, ONR, GE Global Research, BEP, DARPA, IBM, Fuji, and ANSYS Software.
References
- Alvarez, P., Gorria, P., Franco, V., Marcos, J. S., Perez, M. J., Sanchez Llamazares, J. L., … Blanco, J. A. (2010). Nanocrystalline Nd2Fe17 synthesized by high-energy ball milling: Crystal structure, microstructure and magnetic properties. Journal of Physics-Condensed Matter, 22, 216005, 8 pp.
- Alvarez, P., Sánchez-Marcos, J., Sanchez Llamazares, J. L., Franco, V., Reiffers, M., Blancoand, J. A., & Gorria, P. (2010, July 6–9). 14th Czech and Slovak Conference on Magnetism. Košice, Slovakia.
- Balli, M., Fruchart, D., Gignoux, D., Dupuis, C., Kedous-Lebouc, A., & Zach, R. (2008). Giant magnetocaloric effect in Mn1−x(Ti0.5]V0.5)xAs: Experiments and calculations. Journal of Applied Physics, 103, 103908.10.1063/1.2917323
- Balli, M., Fruchart, D., Gignoux, D., & Zach, R. (2009). The “colossal” magnetocaloric effect in Mn1−xFexAs: What are we really measuring? Applied Physics Letters, 95, 072509.10.1063/1.3194144
- Bedanta, S., & Kleemann, W. (2009). Supermagnetism. Journal of Physics D-Applied Physics, 42, 013001.10.1088/0022-3727/42/1/013001
- Bennett, L. H., McMichael, R. D., Swartzendruber, L. J., Hua, S., Lashmore, D. S., Shapiro, A. J., … Nikitenko, V. I. (1995). Magneto-optical indicator film observation of domain structure in magnetic multilayers. Applied Physical Letters, 66, 888–890.
- Bennett, L. H., McMichael, R. D., Swartzendruber, L. J., & Shull, R. D. (1992). Monte Carlo and mean field calculations of the magnetocaloric effect of ferromagnetically interacting clusters. Journal of Magnetism and Magnetic Materials, 104–107, 1094–1095.10.1016/0304-8853(92)90504-H
- Bennett, L. H., McMichael, R. D., Tang, H. C., & Watson, R. E. (1994). Monte Carlo simulations of the magnetocaloric effect in superferromagnetic clusters having uniaxial magnetic anisotropy. Journal of Applied Physics, 75, 5493–5495.10.1063/1.355667
- Bratko, M., Morrison, K., de Campos, A., Gama, S., Cohen, L. F., & Sandeman, K. G. (2012). History dependence of directly observed magnetocaloric effects in (Mn, Fe)As. Applied Physics Letters, 100, 252409.10.1063/1.4729893
- Canepa, F., Cirafici, S., Napoletano, M., & Merlo, F. (2002). Magnetocaloric properties of Gd7Pd3 and related intermetallic compounds. IEEE Transactions on Magnetics, 38, 3249–3251.
- Caron, L., Trung, N. T., & Brück, E. (2011). Pressure-tuned magnetocaloric effect in Mn. Physical Review B, 84, 020414(R).10.1103/PhysRevB.84.020414
- Chen, X., Sahoo, S., Kleemann, W., Cardoso, S., & Freitas, P. P. (2004). Universal and scaled relaxation of interacting magnetic nanoparticles. Physical Review B, 70, 172411.10.1103/PhysRevB.70.172411
- Dagula, W., Tegus, O., Fuquan, B., Zhang, L., Si, P. Z., Zhang, M., … Buschow, K. H. J. (2005). Magnetic-entropy change in Mn1.1Fe0.9P1-xGex compounds. IEEE Transactions on Magnetics, 41, 2778–2780.
- Dagula, W., Tegus, O., Li, X. W., Song, L., Brück, E., Cam Thanh, D. T., … Buschow, K. H. J. (2006). Magnetic properties and magnetic-entropy change of MnFeP0.5As0.5−xSix(x=0-0.3) compounds. Journal of Applied Physics, 99, 08Q105–08Q105-3.
- Della Torre, E., Bennett, L. H., & Jin, Y. (2012). An effect of particle size on the behavior of ferromagnetic materials. Journal of Magnetism and Magnetic Materials, 324, 2189–2192.10.1016/j.jmmm.2012.02.010
- Dung, N. H., Zhang, L., Ou, Z. Q., Brück, E., & Zhang, J. X. (2011). From first-order magneto-elastic to magneto-structural transition in (Mn,Fe)1.95P0.50Si0.50 compounds. Applied Physics Letters, 99, 092511.10.1063/1.3634016
- Franco, V., Pirota, K. R., Prida, V. M., Neto, A. Conde, A., Knobel, M., … Vazquez, M. (2008). Tailoring of magnetocaloric response in nanostructured materials: Role of anisotropy. Physical Review B, 77, 104434.10.1103/PhysRevB.77.104434
- Fujieda, S., Fujita, A., & Fukamichi, K. (2007). Enhancement of magnetocaloric effects in La1−zPrz(Fe0.88Si0.12)13 and their hydrides. Journal of Applied Physics, 102, 023907.10.1063/1.2753590
- Fujieda, S., Fujita, A., Fukamichi, K., & Suzuki, S. (2011). Influence of homogenization of microstructural composition on hydrogen absorption into La(FexSi1−x)13 magnetic refrigerants. IEEE Transactions on Magnetics, 47, 2459–2462.
- Fujita, A., Fujieda, S., Hasegawa, Y., & Fukamichi, K. (2003). Itinerant-electron metamagnetic transition and large magnetocaloric effects in La.FexSi1àx.13 compounds and their hydrides. Physical Review B, 67, 104416.10.1103/PhysRevB.67.104416
- Gama, S., Coelho, A. A., de Campos, A., Carvalho, A. M. G., Gandra, F. C. G., von Ranke, P. J., & de Oliveira, N. A. (2004). Pressure-induced colossal magnetocaloric effect in MnAs. Physical Review Letters, 93, 237202.10.1103/PhysRevLett.93.237202
- Gorria, P., Sánchez Llamazares, J., Álvarez, P. A., Pérez, M. J., Sánchez Marcos, J. S., & Blanco, J. A. (2008). Relative cooling power enhancement in magneto-caloric nanostructured Pr2Fe17. Journal of Physics D: Applied Physics, 41, 192003, 5 pp.10.1088/0022-3727/41/19/192003
- Gribanov, I. F., Golovchan, A. V., Varyukhin, D. V., Val’kov, V. I., Kamenev, V. I., Sivachenko, A. P., … Mityuk, V. I. (2009). Magnetic and magnetocaloric properties of the alloys Mn2−xFexP0.5As0.5 (0≤x≤0.5). Low Temperature Physics, 35, 786–791.10.1063/1.3253401
- Gschneidner, Jr., K. A., Pecharsky, V. K., Pecharsky, A. O., Zimm, C. B. (1999). Recent developments in magnetic refrigeration. Materials Science Forum, 315–317, 69–76.10.4028/www.scientific.net/MSF.315-317
- Gubin, S. P., Koksharov, Y. A, Khomutov, G. B., & Yurkov, Y. G. (2005). Magnetic nanoparticles: Preparation, structure and properties. Russian Chemical Reviews, 74, 489–520.10.1070/RC2005v074n06ABEH000897
- Hu, F-x., Shen, B-g., Sun, J-r., Wang, G-j., & Cheng, Z-h. (2002). Very large magnetic entropy change near room temperature in LaFe11.2Co0.7Si1.1. Journal of Applied Physics, 80, 826–828.
- Hu, F. X., Gao, J., Qian, X. L., Ilyn, M., Tishin, A. M., Sun, J. R., & Shen, B. G. (2005). Magnetocaloric effect in itinerant electron metamagnetic systems La(Fe1−x Cox)11.9 Si1.1. Journal of Applied physics, 97, 10M303.
- Kim, Y. K., Wada, H., & Itoh, S. (2007). Shock compaction of MnAs1−xSbx powder using underwater shock wave. AIP Conference Proceedings, 955, 1105–1108.
- Li, J. Q., Sun, W. A., Jian, Y. X., Zhuang, Y. H., Huang, W. D., & Liang, J. K. (2006). The giant magnetocaloric effect of Gd5Si1.95Ge2.05 enhanced by Sn doping. Journal of Applied Physics, 100, 073904.10.1063/1.2355430
- Lima Sharma, A. L., Gama, S., Coelho, A. A., & de Campos, A. (2008). Irreversibility in cooling and heating processes in the magnetocaloric MnAs and alloys. Applied Physics Letters, 93, 261910.10.1063/1.3058712
- McMichael, R. D., Shull, R. D., Swartzendruber, L. J., Bennett, L. H., & Watson, R. E. (1992). Magnetocaloric effect in superparamagnets. Journal of Magnetism and Magnetic Materials, 111, 29–33.10.1016/0304-8853(92)91049-Y
- Mejía, C., Gomes, A. M., Reis, M. S., & Rocco, D. L. (2011). Fe/Cr substitution in MnAs compound: Increase in the relative cooling power. Applied Physics Letters, 98, 102515.10.1063/1.3560309
- Michalski, S., Skomski, R., Li, X-Zh, Le Roy, D., Mukherjee, T., Binek, Ch, & Sellmyer, D. J. (2012). Isothermal entropy changes in nanocomposite Co:Ni67Cu33. Journal of Applied Physics, 111, 07A930.
- Mitsiuk, V. I., Govor, G. A., & Budzyński, M. (2013). Phase transitions and magnetocaloric effect in MnAs, MnAs0.99P0.01, and MnAs0.98P0.02 single crystals. Inorganic Materials, 49, 14–17.10.1134/S002016851301007X
- Mosca, D. H., Vidal, F., & Etgens, V. H. (2008). Strain engineering of the magnetocaloric effect in MnAs epilayers. Physical Review Letters, 101, 125503.10.1103/PhysRevLett.101.125503
- Mukherjee, T., Sahoo, S., Skomski, R., Sellmyer, D. J., & Binek, C. (2009). Magnetocaloric properties of Co/Cr superlattices. Physical Review B, 79, 144406.10.1103/PhysRevB.79.144406
- Petracic, O., Chen, X., Bedanta, S., Kleemann, W., Sahoo, S., Cardoso, S., & Freitas, P. P. (2006). Collective states of interacting ferromagnetic nanoparticles. Journal of Magnetism and Magnetic Materials, 300, 192–197.10.1016/j.jmmm.2005.10.061
- Ramasamy, K., Mazumdar, D., Bennett, R. D., & Gupta, A. (2012). Syntheses and magnetic properties of Cr2Te3 and CuCr2Te4 nanocrystals. Chemical Communications, 48, 5656–5658.10.1039/c2cc32021e
- Recour, Q., Mazet, T., & Malaman, B. (2008). Magnetic and magnetocaloric properties of Mn3−xFexSn2 (0.1 ≤ x ≤ 0.9). Journal of Physics D: Applied Physics, 41, 185002, 5 pp.
- Rong, C-B., Li, Y., & Ping Liu, J. (2007). Curie temperatures of annealed FePt nanoparticle systems. Journal of Applied Physics, 101, 09K505.
- Rongm, C-B., & Ping Liu, J. (2007). Temperature- and magnetic-field-induced phase transitions in Fe-rich FePt alloys. Applied Physics Letters, 90, 222504.
- Samanta, T., Dubenko, I., Quetz, A., Stadler, S., & Ali, N. (2012). Giant magnetocaloric effects near room temperature in Mn1−xCuxCoGe. Applied Physics Letters, 101, 242405.10.1063/1.4770379
- Samanta, T., Dubenko, I., Quetz, A., Stadler, S., & Ali, N. (2013). Large magnetocaloric effects over a wide temperature range in MnCo1−xZnxGe. Journal of Applied Physics, 113, 17A922.
- Sánchez-Valdés, C. F., Ibarra-Gaytán, P. J., Llamazares, J. L. S., Ávalos-Borja, M., Álvarez-Alonso, P., & Blanco, J. A. (2014). Enhanced refrigerant capacity in two-phase nanocrystalline/amorphous NdPrFe17 melt-spun ribbons. Applied Physics Letters, 104, 212401.10.1063/1.4879544
- Saravanan, P., Gopalan, R., & Chandrasekaran, V. (2008). Synthesis and characterisation of nanomaterials. Defence Science Journal, 58, 504–516.10.14429/dsj
- Shir, F., Yanik, L., Bennett, L. H., Della Torre, E., & Shull, R. D. (2003). Room temperature active regenerative magnetic refrigeration: Magnetic nanocomposites. Journal of Applied Physics, 93, 8295–8297.10.1063/1.1556258
- Shull, R. D. (1993). Magnetocaloric effect of ferromagnetic particles. IEEE Transactions on Magnetics, 29, 2614–2615.10.1109/20.280849
- Shull, R. D., Swartzendruber, L. J., & Bennett, L. H. (1991). The magnetocaloric effect in nanocomposites. In Proceedings of the Sixth International Cryocoolers Conference (pp. 231–246). Annapolis, MD.
- Skomski, R., Binek, C., Mukherjee, T., Sahoo, S., & Sellmyer, D. J. (2008). Temperature- and field-induced entropy changes in nanomagnets. Journal of Applied Physics, 103, 07B329.
- Sougrati, M. T., Hermann, R. P, Grandjean, F., Long, G. J, Bruck, E., Tegus, O., … Buschow, K. H. J. (2008). A structural, magnetic and Mössbauer spectral study of the magnetocaloric Mn1.1Fe0.9P1-xGex compounds. Journal of Physics-Condensed Matter, 20, 475206, 9 pp.
- Sun, N. K., Cui, W. B., Li, D., Geng, D. Y., Yang, F., & Zhang, Z. D. (2008). Giant room-temperature magnetocaloric effect in Mn1−xCrxAs. Applied Physics Letters, 92, 072504.10.1063/1.2884524
- Sun, N. K., Liu, F., Gao, Y. B., Cai, Z. Q., Du, B. S., Xu, S. N., & Si, P. Z. (2012). Effect of microstrain on the magnetism and magnetocaloric properties of MnAs0.97P0.03. Applied Physics Letters, 100, 112407.10.1063/1.3695039
- Tocado, L., Palacios, E., & Burriel, R. (2006). Adiabatic measurement of the giant magnetocaloric effect in MnAs. Journal of Thermal Analysis and Calorimetry, 84, 213–217.10.1007/s10973-005-7180-z
- Tocado, L., Palacios, E., & Burriel, R. (2009). Entropy determinations and magnetocaloric parameters in systems with first-order transitions: Study of MnAs. Journal of Applied Physics, 105, 093918.10.1063/1.3093880
- Trung, N. T., Ou, Z. Q., Gortenmulder, T. J., Tegus, O., Buschow, K. H. J., & Brück, E. (2009). Tunable thermal hysteresis in MnFe(P,Ge) compounds. Applied Physics Letters, 94, 102513.10.1063/1.3095597
- Ucar, H., Craven, M., Laughlin, D. E., & Mchenry, M. E. (2014). Effect of Mo addition on structure and magnetocaloric effect in γ-FeNi nanocrystals. Journal of Electronic Materials, 43, 137–141.
- Ucar, H., Ipus, J. J., France, V., Mchenry, M. E., & Laughlin, D. E. (2012). Overview of amorphous and nanocrystalline magnetocaloric materials operating near room temperature. JOM Journal of the Minerals, Metals and Materials Society, 64, 782–788.
- Ucar, H., Ipus, J. J., Laughlin, D. E., & McHenry, M. E. (2013). Tuning the Curie temperature in −FeNi nanoparticles for magnetocaloric applications by controlling the oxidation kinetics. Journal of Applied Physics, 113, 17A918-17A918-3.
- Wada, H., Matsuo, S., & Mitsuda, A. (2009). Pressure dependence of magnetic entropy change and magnetic transition in MnAs1−x Sbx. Physical Review B, 79, 092407.10.1103/PhysRevB.79.092407
- Yan, A., Müller, K-H., Schultz, L., & Gutfleisch, O. (2006). Magnetic entropy change in melt-spun MnFePGe. Journal of Applied Physics, 99, 08K903–08K903-4.
- Yue, M., Li, Z. Q., Xu, H., Huang, Q. Z., Liu, X. B., Liu, D. M., & Zhang, J. X. (2010). Effect of annealing on the structure and magnetic properties of Mn1.1Fe0.9P0.8Ge0.2 compound. Journal of Applied Physics, 107, 09A939.
- Yue, M., Liu, D., Huang, Q., Wang, T., Hu, F., Li, J., … Zhang, J. (2013). Structure evolution and entropy change of temperature and magnetic field induced magneto-structural transition in Mn1.1Fe0.9P0.76Ge0.24. Journal of Applied Physics, 113, 043925.10.1063/1.4788803
- Zeng, H., Kuang, C., Zhang, J., & Yue, M. (2012). Magnetocaloric effect in bulk nanocrystalline Gd metals by spark plasma sintering. Nanoscience Methods, 1, 16–24.10.1080/17458080.2010.515251
- Zhang, H., Long, Y., Niu, E., Shao, X. P., Shen, J., Hu, F. X., … Shen, B. G. (2013). Influence of particle size on the hydrogenation in La(Fe, Si)13 compounds. Journal of Applied Physics, 113, 17A911.
- Zhang, H., Shen, B. G., Xu, Z. Y., Zheng, X. Q., Shen, J., Hu, F. X., … Long, Y. (2012). Reduction of hysteresis loss and large magnetocaloric effect in the C- and H-doped La(Fe, Si)13 compounds around room temperature. Journal of Applied Physics, 111, 07A909.
- Zhao, J-l., Shen, J., Hu, F-x., Li, Y-x., Sun, J-r., & Shen, B-g. (2010). Reduction of magnetic hysteresis loss in La0.5Pr0.5Fe11.4Si1.6Hx hydrides with large magnetocaloric effects. Journal of Applied Physics, 107, 113911.10.1063/1.3374635