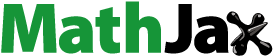
Abstract
Basalt fiber-reinforced polymer composites are receiving considerable attention as they represent a low-cost green source of raw materials. In most cases, fiber-reinforced polymer composites face harsh environments, such as chloride ions in coastal marine environments or cold regions with salt deicing. The resistance of fiber-reinforced polymers subjected to the above environments is critical for the safe design and application of such composites. This research aims to develop a framework to investigate the durability properties of the lightweight expanded clay basalt fiber polymer reinforced concrete exposed to the NaCl environment. The specified quantity of concrete structural elements was cured in its specified curing solution for 28-day curing period before testing. The main effect of micro silica is to enhance concrete strength and durability. Dispersed basalt fibers with 20 mm length and 1.6 percent volume were added to the concrete mixtures. The concrete beams were reinforced with 2∅10 mm rebars as reinforcement while the concrete cylindrical columns were reinforced with dispersed chopped basalt fiber. The results show that addition of dispersed chopped basalt fiber in the concrete caused an increase in the flexural and compressive strength of the concrete structural elements. Micro silica enhanced the concrete strength even when immersed in NaCl solution. Basalt fiber and micro silica fume utilization enhanced the mechanical properties of the concrete.
PUBLIC INTEREST STATEMENT
The materials used in concrete construction place important role in the durability and effectiveness of the concrete structure. Due to environmental factors, it has become a necessity concrete to be reinforced with the proper materials that will withstand harsh environments. Based on these, this paper investigated the use of basalt fiber reinforcements like rebar and chopped fiber, the checked the effect of micro silica in salty water and in non-salty water. This is believed to answer the difficult questions with affordability in civil/structural engineering in concrete structural location.
1. Introduction
Lightweight concrete has been widely consumed in several parts of the construction industry such as masonry concrete block and wall panel productions. The main attributes of lightweight concrete may include many advantages like cost-effectiveness, low density, thermal conductivity, and high strength to density ratio (Zaetang et al., Citation2013). However, some disadvantageous properties are including brittleness, reduce mechanical strength compare to regular concrete, and crack forming at early ages (Hamad, Citation2014). The low density of lightweight concrete highly reduces the structural loads which decreases the cost of the production of the concrete structural elements. Therefore, it is widely preferred for the structures constructed in seismic zones (Kockal & Ozturan, Citation2011; Posi et al., Citation2013). Lightweight concrete is traditionally produced with Portland cement, lightweight aggregates (diatomite, perlite, pumice from natural sources, expanded clay), and recycled clay bricks (recycled sources) (Aliabdo et al., Citation2014; Kramar & Bindiganavile, Citation2013; The Pennsylvania State University, Citation2014). The construction industry requires new developments due to the high demand for big structures which increase the need for better performance concrete. Hence, many types of materials were added to enhance concrete performance like fibers (Barabanshchikov & Gutskalov, Citation2016; Citation2017), and nanomaterials (Nizina et al., Citation2017).
However, its potential is not fully realized, due to the small specific surface of the steel fiber, the low adhesion of cement stone to it, and the insufficient strength of the concrete itself, which leads to the “pulling” of the fibers upon its destruction. In this regard, basalt fiber (Babkov et al., Citation2007; Komokhov, Citation2001), which is considered new and rarely used in cement concrete in some countries because of its non-popularity, attracts attention. In strength, it surpasses steel and, due to the small diameter of the fibers (9–20 microns), has a much larger specific adhesion surface to cement stone than steel, having a chemical affinity with it. Moreover, the elongation at break of the basalt fiber is two times lower than steel, which allows it to prevent the formation of microcracks more effectively in concrete during loading.
The studies on the use of basalt fiber in fine-grained concrete (Borovskikh & Khozin, Citation2008) showed the effectiveness of its use. So, with the introduction of 4% basalt fiber, the flexural strength of concrete increased by 2.2 times, and the compressive strength 1.5 times. Attention should be paid to hardening under “pure” tension (splitting of CK samples), which is 4.5 times higher than hardening under compression. However, it is worth noting that the possibility of using basalt fiber in cement systems largely depends on the resistance of the fibers to the action of cement hydration products.
It is reported by many researchers that fiber addition, especially steel, carbon, and polypropylene fibers inclusion to the lightweight concrete mixes improved the material properties such as modulus of elasticity, abrasion resistance, freeze-thaw resistance, drying shrinkage, and porosity (Saha, Citation2018; Shariq et al., Citation2013). Among all fiber types, carbon fiber is commonly utilized due to its superior contribution to the composite materials such as high strength, good stability, and good bonding behavior with the cement; however, its high cost limits the application of carbon fiber reinforced concrete (Frazão et al., Citation2018). Basalt fiber is also used as an eco-friendly and high-performance fiber in the research of fiber reinforced concrete materials. Basalt fibers can enhance both compressive and flexural strengths and toughness together with the fracture energy in the cement matrix composite applications (Ralegaonkar et al., Citation2018). Basalt fibers are also having a better binding performance with the cement due to their similar chemical properties with cement, and it is cheaper compared to the other widely used fibers in civil engineering research (Libre et al., Citation2011). Basalt fibers have many times greater tensile strength than steel fibers, and it has been widely used as small pieces during the mix design of concrete composites in civil engineering applications (Arslan, Citation2016). Steel and polypropylene fibers addition to the lightweight concrete mixes has been widely researched, and different results are reported due to the variety of the examined parameters. In most cases, positive influences have been reported for the strength improvement of the cementitious composites (Balendran et al., Citation2002; Grabois et al., Citation2016; Kim et al., Citation2010). Basalt fiber inclusion has also been shown to increase the toughness of the concrete; however, it is difficult to assess the relative benefits since different test methods were applied (Elgabbas et al., Citation2016).
The impacting behavior and damage evolution of basalt fiber reinforced concrete (BFRC) with different fiber mass fractions ranging from 0%~0.60% were tested by (Zhao et al., Citation2010) using three-point bending impact equipment and ultrasonic testing technology. The continued damage detection based on ultrasonic testing was used to reveal the damage evolution process, and the stereomicroscope was applied to observe the surface cracks. The results show that the basalt fiber (BF) has little effect on the compressive strength of BFRC, but it can significantly improve the impact toughness of BFRC.
Considering BFRC tensile strength and crack resistance, the effect of different lengths of BF (Budkonstruktsiya, L. L. C., Technobasalt-Invest, Citation2013; Ma et al., Citation2010), inclusion dosage (Arslan, Citation2016; D. Wang et al., Citation2019; Zheng et al., Citation2019), and different types (bundle dispersion fibers and minibars) (Branston et al., Citation2016) were investigated. Results of these studies indicated that the increase in the length and fiber dosage of basalt fibers causes a rise in the tensile strength, both types of basalt fibers increase pre-cracking strength, but only minibars enhance the post cracking behavior.
Fiber-reinforced polymers (FRPs) are increasingly being used for the repair and rehabilitation of existing structures (Li et al., Citation2017; Pan et al., Citation2015). The growing use of FRPs for strengthening and retrofitting can be explained by their specific strength and specific stiffness, good fatigue properties, ease of handling,
and primary resistance to corrosion (Nishizaki et al., Citation2015). Chloride ions are a major cause of corrosion of steel in concrete, which governs the effective life of the structures and their maintenance costs (Chen et al., Citation2007). The corrosion-resistant characteristic makes FRPs ideal for applications subjected to the chloride ion environment (Dong et al., Citation2016; Mishra & Chakraborty, Citation2016). Due to the versatile applications of concrete structures, FRP-reinforced concrete members may often be exposed to wetting and drying cycles, deicing salts in cold climates, sea salts in hot–humid climates, and many other severe environments (Ceroni et al., Citation2006). In those applications, the long-term durability of the FRP composites is often one of the major advantages over the conventional materials (Lu et al., Citation2015; Xian & Karbhari, Citation2007; Z.K. Wang et al., Citation2017); durability is a significant consideration, especially because of the requirements of long service life without frequent inspection and maintenance (Nishizaki et al., Citation2015).
Unfortunately, the durability of FRP composites is more complex than the corrosion of steel reinforcement because degradation of the material could depend both on the resin matrix and fibers and their interface bond behavior (Al-Sabagh et al., Citation2017; Ceroni et al., Citation2006; Lu et al., Citation2015; Sadik & Gokhan, Citation2019). Many previous studies have been conducted on the performance degradation of FRP-reinforced structures in a chloride-containing environment (Chen et al., Citation2007; Dong et al., Citation2016; Mishra & Chakraborty, Citation2016; Z.K. Wang et al., Citation2017).
The study (Ashkan et al., Citation2020) investigated the effect of the BF on the lightweight concrete fabricated with silica fume and fly ash, it was noticed that the presence of BF caused a reduction in the slump and compressive strength by 37% and 13%, respectively but, addition of BF enhanced the tensile, flexural strength, and shrinkage of the concrete. Furthermore, it was identified that all mixtures had a “good” level of water absorption quality and thus were placed in the category of “structural concrete”.
The study (Karaburc et al., Citation2020) investigated the mechanical and durability properties of basalt fiber reinforced pumice lightweight concrete (BPLC) containing nano ground calcium carbonate (GCC). In the research, GCC was utilized as ordinary Portland cement replacement material at the percentages of 5%, 10%, 15%, 20% and 25%, and BF with the 6 mm length were added in two contents of 0.5% and 1% by volume. It was obtained that GCC added mixes had lower mechanical strength results at the early ages; however, comparable strengths with the reference mixes at later ages also resulted in decreased water absorption, sorptivity and increased magnesium sulphate resistance compared to the reference lightweight concrete. BF utilization improved the mechanical properties of the BPLC, but fiber inclusion lessened the fresh concrete properties.
This paper (Elango et al., Citation2020) dealt with the flexural behavior study on reinforced concrete beams with steel and basalt rebar using Portland Pozzolana Cement. From the investigation, basalt reinforced beam (BRB) showed significant greater deflection when compared to steel reinforced beam due to lower elastic modulus, whereas flexural load carrying capacity of BRB increases due to enriched tensile strength property in basalt rebar, significant increase of tensile strength in BRB was also noticed. The paper concluded that basalt rebar can be effectively used in construction as a replacement of conventional steel reinforcement for sustainable development.
It is necessary to check the durability of structural materials that are bound to necessary services. Structural reinforced concrete is attacked by external materials that expose them to cracks and failures. When the reinforcing materials in concrete start rusting, the concretes start cracking and degradation because of loss in the bond between the concrete and the reinforcing materials. Because of these challenges, this research paper has the task to suggest, analyze, and show the durability of the ECC reinforced with BF, the effects of BF in ECC reinforcement when exposed to a salty environment.
2. Experimental setup
Despite the great care in making specimens in laboratory studies, it cannot be claimed that all specimens were the same. In this study, an equal making process of specimens has been done in all tests to minimize differences. The experimental study of concrete is carried out following the CIS Interstate Standard GOST 10,180–2012 (GOST 1Citation0180–2012, 2013).
2.1. Materials
The materials for the lightweight concrete mix and the production of the concrete for this study are listed below for better illustration.
Lightweight expanded clay aggregate of 5–8 mm fraction = 200 kg/m3 as coarse aggregate.
An expanded clay aggregate is a lightweight aggregate from clay. The clay is dried, heated, and burned in rotary kilns at 1100–1300 °C inhabiting essential properties like lightweight, insulating, strong, non-combustible, and fire-resistant, extremely stable, and durable, natural material for sustainable construction, versatility, and high drainage capacity (Md et al., Citation2016; Sepehr et al., Citation2014; Shafigh et al., Citation2011; Slater et al., Citation2012; Zendehzaban et al., Citation2013). The chemical composition of lightweight expanded clay aggregate according to the analysis of different authors is illustrated in .
Quartz sand of 0.6–1.2 mm fraction as fine aggregate with fineness modulus of 2.7 = 585 kg/m3 as the fine aggregate.
Table 1. Chemical composition of LECA
A feature of the proposed quartz is the presence of coarse-grained sand, with a large modulus of fineness up to M3,5. Quartz sand has a rounded part with a low content of clay inclusions and inclusions of soft rocks. The resulting quartz sand undergoes additional enrichment and drying. The moisture content is up to 0.2% (CitationFractionated quartz sand, xxxx). The chemical composition of quartz sand is shown in .
Mineral filler Silverbond Quartz flour of 50 µm = 100 kg/m3 as the mineral filler.
Table 2. Chemical composition of quartz sand
Quartz flour is produced by grinding chemically pure, natural quartz sand to a finely divided state. The technology used guarantees the stability of the chemical composition during grinding and allows you to get a constant particle size distribution of quartz flour. The crushed quartz flour is represented by rounded particles with uneven, broken edges. Quartz differs from other mineral fillers in hardness, abrasion and chemical resistance, anti-corrosion, and low coefficient of thermal expansion and the properties are illustrated in . Quartz is a chemically stable mineral; it is soluble only in hydrofluoric acid. With a low oil absorption and a small surface area of the particles, the use of quartz flour will allow you to get a system with a high degree of filling.
Binder Holcim Portland cement M500 D20 CEM II 42.5 N.
Table 3. Compositions and properties of quartz flour of 50 µm
The characteristics of Holcim Portland cement M500 D20 CEM II 42.5 N. M—brand, 500 is a figure showing the average compressive strength for 28 days in kg/cm2, D—additives, 20—allowable number of additives in % (up to 20%), CEM II—cement containing additives, and the content of additives is 6–20%, I-type additives, limestone, 42.5-class compressive strength for 28 days, must be at least this value, and B-quick hardening. shows the properties of this type of Portland cement.
Organic mineral-based additives: Silica fume (Micro silica) = 62.5 kg/m3 and fly ash = 62.5 kg/m3.
Table 4. Physical and chemical properties of Holcim Portland cement
states the chemical compositions of silica fume (micro-silica) and fly ash in percentage (%) which a better insight of the mineral-based additives is explained below.
Table 5. Chemical compositions of silica fume (micro-silica) and fly ash in percentage (%)
Silica fume is a product of ferroalloy production and is formed during the smelting of ferrosilicon and its alloys.
By granulometric composition, the average particle size of silica fume is approximately 100 times smaller than the average grain size of cement. When using silica fume for the manufacture of especially strong concrete, thousands of spherical microparticles surround each cement grain, compacting the cement mortar, filling the voids with strong hydration products, and improving adhesion to aggregates, much more effectively than other mineral additives, such as zeolite tuff, blast furnace, and boiler slag.
It is used as a highly active additive to concrete. It is intended for the preparation of special high-grade concrete for strength and waterproofness, foam concrete, dry building mixes, rubber, ceramics, tiles, tiles, and refractory masses (CitationSilica Fume MK-85, xxxx).
The addition of silica fume helps reduce cement consumption (up to 200–450 kg/m3).
As a result of physical and chemical effects, a favorable change in the microstructure of the test occurs, associated with a significant decrease in porosity in the zone of capillary pores. A change in the structure of pores in concrete is considered by many researchers as the main factor in the influence of silica fume on the mechanical properties and strength of concrete. These changes are reflected in the decrease in concrete permeability. Reduced water permeability enhances the resistance of concrete to aggressive environments.
High properties of silica fume improve concrete characteristics such as compressive strength, adhesion and wear resistance, frost resistance, chemical resistance, and significantly reduce permeability. Having dispersed, the smallest particles of silica fume compact and stabilize the mixture and significantly reduce the protrusion of water and separation.
Silica fume can provide a compressive strength far exceeding that of conventional concrete, and here only the aggregate strength is a limiting factor. When using natural aggregates, strengths exceeding 150 kg/cm2 are achieved, and when using special high-strength aggregates, strengths of 300 kg/cm2 can be achieved. The addition of silica fume provides increased crack resistance of concrete. Silica fume reduces the PH of water in the pores of a cement gel.
The effect of pore-filling created by pozzolanic spherical microparticles contributes to a significant reduction in capillary porosity and permeability of concrete. Impermeable concrete can be obtained with moderate content of silica fume and relatively low content of ordinary Portland cement. Since silica fume has a greater effect on permeability than strength, concrete with silica fume content will always be much less permeable than the concrete of equivalent strength on ordinary Portland cement. Low permeability to water and gases (W12—W16).
Reduced alkalinity of concrete with silica fume should weaken its resistance to carbonization and chlorides. Low permeability and increased density of cement stone provide excellent frost resistance of concrete with silica fume (F200—F600, up to F1000 with special additives).
Low permeability and low free lime content increase the resistance of concrete to aggressive chemicals. Long-term tests have shown that in terms of its potential resistance to sulfates, it is equal to sulfate-resistant Portland cement.
Fly ash: The use of fly ash can lead to significant retardation of the setting time, which means that finishing operations may have to be delayed. At normal temperatures, the rate of the pozzolanic reaction is slower than the rate of cement hydration, and fly ash concrete needs to be properly cured if the full benefits of its incorporation are to be realized. When high levels of fly ash are used it is generally recommended that the concrete is moist cured for a minimum period of 7 days. It has been recommended that the duration of curing is extended further (for example, to 14 days) where possible, or that a curing membrane is placed after 7 days of moist curing. If adequate curing cannot be provided in practice, the amount of fly ash used in the concrete should be limited. The finishing and curing requirements for high-volume fly ash concrete exposed to cyclic freezing and thawing in the presence of deicing salts are discussed in the section Effect of Fly Ash on the Durability of Concrete (Thomas, Citation2007).
Super plasticizing and water-reducing additive Sika Plast concrete in liquid form = 8 l/m3.
Super plasticizing increases the plasticity of concrete and reduces the water added to the concrete mixture. It has the property of improving the strength of concrete, reduces lumps in the concrete mixture.
Tap water at room temperature. Generally, water that is suitable for drinking is satisfactory for use in concrete = 255 l/m3 for mixing.
For concrete reinforcement, the following materials are needed.
Chopped basalt fiber BF with diameter (d) of 15 µm and length (l) of 20 mm. 1.6% BF which was derived from the research work (Galishnikova et al., Citation2021).
Basalt rebar of diameter 10 mm for concrete reinforcement.
states the properties of the reinforcement materials for this research.
Table 6. Chemical composition of basalt fibers chopped strands
Table 7. Properties of a basalt rebar
2.2. Specimen making and research method
2.2.1. ECC beams
The flexural tests were done on 48 expanded clay concrete beams:
12 ECC beam reinforced with basalt rods of 2∅10 mm without BF with micro silica.
12 ECC beam reinforced with basalt rods of 2∅10 mm without BF and micro silica.
12 ECC beam with BF and micro silica-reinforced with basalt rods 2∅10 mm.
12 ECC beam with BF reinforced with 2 (two) basalt rods 2∅10 mm without micro silica.
From the four sets of lightweight concrete beams of 12 beams each, 6 beam specimens from each of the ECC beams were cured in a water bath containing sodium chloride (NaCl), and the other 6 beam specimens from each of the ECC beams were cured in a water bath without sodium chloride. All durability and mechanical tests were conducted on day 28.
Below in , the dimensions of the ECC beam are illustrated, showing locations of the basalt fiber rebars.
The 1.6% basalt Fiber used for this experiment were selected from a series of experiment in research work (Galishnikova et al., Citation2021). In the research work, the flexural and compressive strength of lightweight expanded clay concrete cubes and rectangular prisms with series of dispersed chopped BF in the following percentages: 0.45%, 0.9%, 1.2%, 1.6%, and control specimens without dispersed chopped BF were analyzed. From the experiments and analysis, it was discovered that 1.6% basalt fiber addition to the lightweight expanded clay concrete showed higher flexural and compressive strength. Based on this, the author is using 1.6% basalt fiber as dispersed fiber for this experiment (Galishnikova et al., Citation2021).
The concrete beams are molded in a metal beam according to CIS Interstate Standard GOST 10,180–2012 (GOST 1Citation0180–2012, 2013) as shown in . The concrete beams are of dimensions Length 1500 mm x Width 50 mm x Depth 120 mm, as shown in .
After molding the concrete beams in the molds, they were covered with polyethylene and kept at room temperature (20 ± 5) °C and relative air humidity (95 ± 5) %. On the 73rd hour, the concrete beams were removed from the molds and kept in the curing bath till the 28th day. The beams were tested for deflection on MATEX hydraulic press as shown in .
The effect of BF addition and replacing cement with micro silica was investigated by mechanical and durability laboratory tests. Spread diameters of the blends were evaluated by the slump test. The slump test was repeated three times with 20 min (the first test time includes mixing duration) intervals to determine slump losses. Setting times of the specimens were determined according to the requirements of BS EN 196–3 (BS EN 196–3, Citation2016).
Flexural strength tests were performed on Length 1500 mm x Width 50 mm x Depth 120 mm specimens as per the requirements of EN 14,651 (EN 14651, Citation2005) as shown in .
2.2.2. ECC cylindrical columns
The compressive tests were done on 24 expanded clay concrete beams:
6 ECC cylindrical columns without BF and with micro silica.
6 ECC cylindrical columns without BF and micro silica.
6 ECC cylindrical columns reinforced with dispersed chopped BF and micro silica.
6 ECC cylindrical columns reinforced with dispersed chopped BF without micro silica.
From the four sets of lightweight cylindrical concrete columns of 6 cylindrical columns each, 3 column specimens from each of the ECC cylindrical columns were cured in a water bath containing sodium chloride (NaCl), and the other 3 column specimens from each of the ECC cylindrical columns were cured in a water bath without sodium chloride. All durability and mechanical tests were conducted on day 28.
The 1.6% basalt Fiber used for this experiment were selected from a series of experiment in research work (Galishnikova et al., Citation2021) the same as for the ECC beam earlier discussed.
The ECC cylindrical columns are molded in a metallic Euro cylinder beam according to EN 1992-1-1 (English): Eurocode 2 (EN Citation1992-1-1 (English), 2004). The ECC cylindrical columns are of dimensions Height 300 mm x Diameter 150 mm ().
After molding the ECC cylindrical columns in the molds, they were covered with polyethylene and kept at room temperature (20 ± 5) °C and relative air humidity (95 ± 5) %. On the 72nd hour, the concrete columns were removed from the molds and kept in the curing bath till the 28th day. The cylindrical columns were tested for compression on Hydraulic press PG-100 as shown in .
The effect of BF addition and replacing cement with micro silica was investigated by mechanical and durability laboratory tests. Spread diameters of the blends were evaluated by the slump test. The slump test was repeated three times with 20 min (the first test time includes mixing duration) intervals to determine slump losses. Setting times of the specimens were determined according to the requirements of BS EN 196–3 (BS EN 196–3, Citation2016).
The porosity index (γ) is defined by the volume fraction of permeable pores contained in a specimen of hardened concrete was determined according to ABNT (Associação Brasileira de Normas Técnicas—ABNT, Citation1987), by means (1):
Where: is the water-saturated specimen weight,
the weight of dry specimen and
the weight of water-saturated specimen immersed in liquid.
Some ECC cylindrical columns after the day 28 curing period and dried up were immersed in a liquid bath for 72 hours to check the weight of the cylinder on the 72nd hour.
Compressive strength tests were performed on Height 300 mm x Diameter 150 mm specimens (see ) as per the requirements of EN 14,651 (EN Citation1991-1-1 (English), 2002).
3. Results and discussions
The laboratory experimental results of the ECC beams and ECC cylindrical columns are illustrated in this section. These test results were derived on the day 28 curing period and analysed.
3.1. ECC beams
Regular concrete Beam: The test was performed to evaluate the effect of curing concrete in saltwater. shows the flexure of expanded clay basalt rod-reinforced concrete beam cured in both tap water and saltwater. The beam cured in tab water (FBW), initially the first noticeable cracks in the beam appeared when the load reached 2.2 KN (1.97 mm deformation) after that the cracking in the stretched zone appeared when the load reached 4.2 KN (2.72 mm deformation). The destruction of the beam occurred when the load reached 4.7 KN (3.19 mm deformation). The beam cured in saltwater (FBSW) shows the first noticeable crack when the load reached 2.2 KN (1.68 mm deformation) and the failure accrued when the load reached 2.4 KN (2.4 mm deformation). The saltwater decreases the maximum load of the beam by 49% and the maximum deformation by 30.8%. Moreover, saltwater changes the behavior of the beam under loads from linear to nonlinear as shown in .
Figure 7. Flexure of expanded clay basalt rod-reinforced concrete beam without micro silica additive cured in both tap water and saltwater
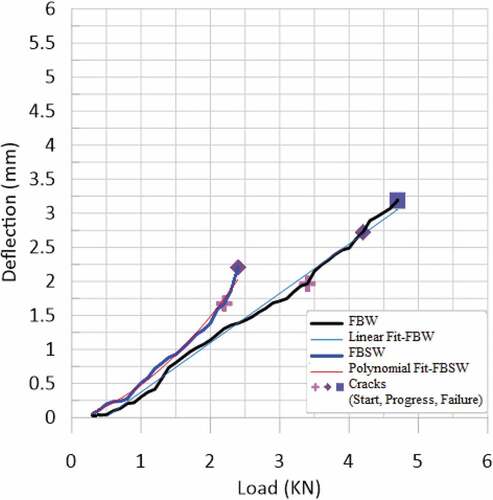
Micro Silica (MS) concrete Beam: Firstly, the beam with micro silica cured in tab water showed the first visible crack when the load reached 3.8 KN (2.6 mm deformation). The cracks in the stretched zone appeared at a load equal to 4.7 KN (3.26 mm deformation) and the failure of the beam accrued at the load equal to 5 KN (3.6 mm deformation). Secondly, the beam with micro silica cured in saltwater showed the first visible cracks at load 2.2 KN (1.75 mm deformation). The cracks increased rapidly to reach the stretched zone at a load equal to 2.6 KN (3.23 mm deformation) and reach the failure point at a load equal to 2.9 KN (3.7 mm deformation). Micro silica increased the maximum load of the concrete beam by 6.4% and the maximum deformation by 12.9% compared to the regular beam cured in tap water. However, the main impact of using the micro silica is boosting the maximum deformation of the concrete beam by 67.3% and the maximum load by 20.8% by when the beam is cured in saltwater compare to a regular concrete beam cured in saltwater. Also, the decrease in the maximum load of the beam due to the curing in saltwater was decreased from 49% to 42%. The maximum deformation is comparable in both cases. The nonlinear performance is shown in for beams cured in saltwater and linear performance for beams cured in tap water. Adding the micro-silica even delayed the start of cracks by 4.6%.
Figure 8. Flexure of expanded clay basalt rod-reinforced concrete beam with micro silica additive cured in both tap water and saltwater
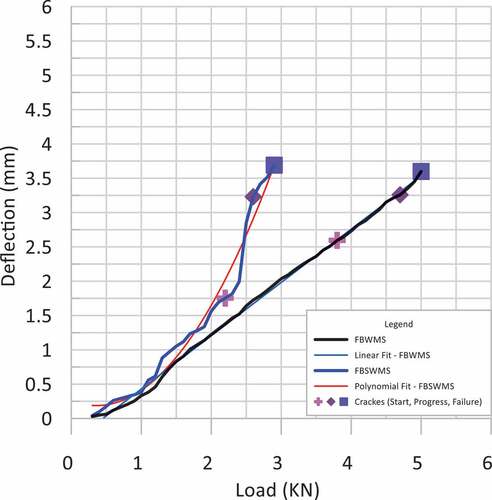
BF concrete beam: The first visible cracks appeared in the beam with BF cured in tab water when the load reached 3.3 KN (3.68 mm deformation). after that, the cracks in the stretched zone appeared at a load equal to 5.1 KN (5.5 mm deformation) and the failure of the beam accrued at the load equal to 6.5 KN (6.49 mm deformation).
Secondly, the beam with BF cured in saltwater showed the first visible cracks at load 4.4 KN (2.63 mm deformation). The cracks expanded to reach the stretched zone at a load equal to 5.0 KN (3.16 mm deformation) and the beam reached the failure point at a load equal to 5.5 KN (3.84 mm deformation). shows the effect of curing basalt fiber concrete beams in both saltwater and tab water. BF shows an important enhancement for the concrete beams by increasing the maximum deformation by 103% and 74% for both tab water and saltwater, respectively. Moreover, the maximum loads also increase by 38% and 129% for both tab water and saltwater, respectively. However, the decrease in the max deformation because of saltwater was equal to 40.85% while the decrease in maximum load is equal to 15.4%.
Figure 9. Flexure of expanded clay basalt rod-reinforced concrete beam with BF cured in both tap water and saltwater
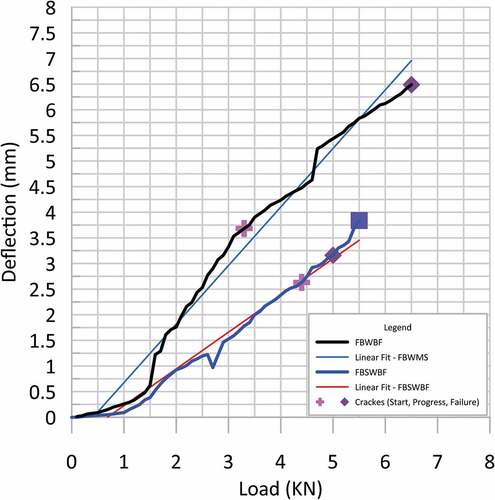
BF and micro-silica concrete beam: show the effect of curing micro silica basalt fiber concrete beams in both tab water and saltwater. The first beam was cured in tab water and the second one was cured in saltwater. The first beam showed the first cracks when the load reached 4 KN (2.57 mm), then the crack propagation appears at the load equal to 4.9 KN (3.07 mm) at the end the failure of the beam happened when the load reached 7.1 KN (4.28 mm). the first cracks appeared in the second beam when the load reached 4.9 KN (3.07 mm), then the crack propagation appears at the load equal to 5.3 KN (3.39 mm) at the end the failure of the beam happened when the load reached 5.9 KN (4.07 mm). The concrete beam cured in tap water has higher deformation and load compared to the regular beam by 34% and 51%, respectively. Moreover, the maximum loads and deformation for the beam cured in saltwater also increased by 146% and 85%, respectively. Nonetheless, the decrease in the max deformation because of saltwater was equal to 4.8% while the decrease in maximum load is equal to 17%. shows all the maximum loads of all types of samples used in this research.
3.2. ECC cylindrical columns
3.2.1. Porosity evaluation
The ECC cylindrical columns used in the porosity evaluation () are the cylindrical columns containing micro silica and cured in normal water. Reason being that they gave the best compressive strength as shown in table 9 and they have more weight than the ECC cylinders without micro silica. Notwithstanding, some of the cylinders were immersed in NaCl water to check the porosity evaluation.
Table 8. Porosity of ECC cylindrical columns
EquationEquation (1)(1)
(1) verified for ECC cylindrical columns immersed in normal water that the incorporation of basalt fibers into the concrete mixture results in a porosity index of 5.37 as compared to 4.98 in plain ECC and incorporation of basalt fibers into the concrete mixture results in a porosity index of 5 as compared to 3.7 in plain ECC for ECC cylindrical columns immersed in NaCl water.
3.2.2. Compressive strength
In general, all the samples cured in NaCl solution show less compressive strength than the samples cured in tap water. A noticeable decrease in performance occurs in in the plain ECC cylinders with micro silica with 24.5%. However, the ECC cylinders with BF shows the minimum decrease with 4.4% .
4. Conclusion
The degradation of the flexure of the expanded clay basalt rod-reinforced concrete beam due to corrosion of saltwater had been inspected. On the other hand, the enhancement of the concrete beam due to adding basalt fiber and micro-silica additive was also investigated. Four types of beams were investigated in both tap water and saltwater environment. The degradation of the maximum loads and deflection was compared. The major conclusions are as follows:
The concrete beam cured in saltwater drop both the maximum load by 49% and the maximum deformation by 30.8%.
Micro silica improved the maximum load of the concrete beam by 6.4% and the maximum deformation by 12.9% compared to the regular beam cured in tap water. Although, BF increased the maximum deformation by 103% and the maximum load by 38% and the concrete beam with both BF and Micro silica cured in tap water has higher deformation and load compared to the regular beam by 34% and 51%, respectively.
The micro-silica reduced the effect of curing in saltwater for the maximum load of the beam by 7%.
Micro silica improved the maximum deformation of the concrete beam by 67.3% and the maximum load by 20.8% for the beams cured in saltwater compare to regular concrete beam cured in saltwater.
The BF concrete beams cured in saltwater have higher maximum deformation and maximum load by 74% and 129%, respectively. As A Result, the reduction of the max deformation equal to 40.85% while the reduction in maximum load is equal to 15.4%.
The beams with MS and BF have the maximum loads and deformation for the beam cured in saltwater also increased by 146% and 85%, respectively. however, the reduction of the max deformation because of saltwater was equal to 4.8% and the decrease in maximum load is equal to 17%.
NaCl decrease the compressive strength of cylinder ECC by 10%. While the percentage increased to 24.5% after adding micro silica to the mix.
Micro silica increases the compressive strength of ECC cylinder by 25% while by adding BF and both BF and micro silica the compressive strength increased by 56% and 69.7%, respectively.
The NaCl decrease the compressive strength of ECC cylinder by 10%, 4.4%, 24.5%, and 8.85% for Plan ECC, BF ECC, Micro silica ECC, and BF micro silica ECC cylinders, respectively.
Acknowledgements
The publication has been prepared with the support of the “RUDN University Program 5-100”.
Disclosure statement
There is not conflict or competing of interest in preparing this paper.
Additional information
Funding
Notes on contributors

Paschal Chimeremeze Chiadighikaobi
Chiadighikaobi Paschal Chimeremeze is a Nigerian. He holds a PhD in Civil Engineering from People's Friendship University of Russia (RUDN University). His research interests are in: Structural materials, composite materials, structural design and analysis, computational civil engineering, steel frame stability analysis, computational mechanics of structural systems, concrete reinforcement, Nano-concrete, fiber dispersed concrete reinforcement, lightweight concrete, basalt fiber, finite element analysis, green city. Due to the challenges of affordable with high strength and durability, the author has been conducting research and experiments on the effects and uses of basalt fiber in concrete structures.
References
- Aliabdo, A. A., Abd-Elmoaty, A. E. M., & Hassan, H. H. (2014). Utilization of crushed clay brick in concrete industry. Alexandria Engineering Journal, 53(1), 151–21. https://doi.org/10.1016/j.aej.2013.12.003
- Al-Sabagh, A., Taha, E., Kandil, U., Awadallah, A., Nasr, G.-A. M., & Reda Taha, M. (2017). Monitoring moisture damage propagation in GFRP composites using carbon nanoparticles. Polymers, 9(3), 94. https://doi.org/10.3390/polym9030094
- Arslan, M. E. (2016). Effects of basalt and glass chopped fibers addition on fracture energy and mechanical properties of ordinary concrete: CMOD measurement. Construction and Building Materials, 114(1), 383–391. https://doi.org/10.1016/j.conbuildmat.2016.03.176
- Ashkan, S., Parisa, N., Ali, S. P., Mohammad, M. M., Hossein, M., & Elaheh, H. V. (2020). Prediction of mechanical properties of lightweight basalt fiber reinforced concrete containing silica fume and fly ash: Experimental and numerical assessment. Journal of Building Engineering, 32, 101732. https://doi.org/10.1016/j.jobe.2020.101732.
- Associação Brasileira de Normas Técnicas – ABNT. (1987). NBR 9779:1987: Argamassa e concreto endurecidos – Determinação da absorção da água por capilaridade - Método de ensaio. Rio De Janeiro. https://www.passeidireto.com/arquivo/6572074/nbr-9779-argamassa-e-concreto-endurecidos-determinacao-da-absorcao-de-agua-por-c
- Babkov, V. V., Bazhenov,, Yu, M., Bykova, A. A. (2007). Cements, concretes, mortars, and dry mixes (Vol. Part I, 804). NPO Professional.
- Balendran, R. V., Zhou, F. P., Nadeem, A., & Leung, A. Y. T. (2002). Influence of steel fibres on strength and ductility of normal and lightweight high strength concrete. Building and Environment, 37(12), 1361–1367. https://doi.org/10.1016/S0360-1323(01)00109-3
- Barabanshchikov, Y., & Gutskalov, I. 2016. Strength and deformability of fiber reinforced cement paste on the basis of basalt fiber. Advances in Civil Engineering, 5. ( Article ID 6562526). https://doi.org/10.1155/2016/6562526.
- Borovskikh, I. V., & Khozin, V. G. “Investigation of the influence of methods for introducing short-chopped basalt fiber on the strength characteristics of fine-grained concrete”, Construction complex of Russia: Science, education, practice: materials of the international scientific-practical conference. - Ulan-Ude: Publishing House of VSTU, pp. 27–31, 2008.
- Branston, J., Das, S., Kenno, S. Y., & Taylor, C. (2016). Mechanical behaviour of basalt fibre reinforced concrete. Construction and Building Materials, 124, 878–886. https://doi.org/10.1016/j.conbuildmat.2016.08.009
- BS EN 196–3. (2016). Methods of testing cement -– Part 3: Determination of setting times and soundness. British Standard. https://doi.org/10.1111/j.1748-720X.1990.tb01123.x.
- Budkonstruktsiya, L. L. C., Technobasalt-Invest. “Test conclusions on tensile strength in bending of basalt fiber concrete”, Research and Development Enterprise Budkonstruktsiya LLC, Ukraine, 2013, http://www.technobasalt.com
- Ceroni, F., Cosenza, E., Gaetano, M., & Pecce, M. (2006). Durability issues of frp rebars in reinforced concrete members. Cement and Concrete Composite, 28(10), 857–868. https://doi.org/10.1016/j.cemconcomp.2006.07.004
- Chen, Y., Davalos, J. F., Ray, I., & Kim, H. Y. (2007). Accelerated aging tests for evaluations of durability performance of FRP reinforcing bars for concrete structures. Composite Structures, 78(1), 101–111. https://doi.org/10.1016/j.compstruct.2005.08.015
- Dong, Z., Wu, G., & Xu, Y. (2016). Experimental study on the bond durability between Steel-Frp Composite Bars (SFCBS) and sea sand concrete in ocean environment. Construction and Building Materials, 115, 277–284. https://doi.org/10.1016/j.conbuildmat.2016.04.052
- Elango, K. S., Gopi, R., Jayaguru, C., Vivek, D., Saravanakumar, R., & Rajeshkumar, V. (2020). Experimental investigation on concrete beams reinforced with basalt fiber reinforced polymer bars. Materials Today: Proceedings. https://doi.org/10.1016/j.matpr.2020.10.840
- Elgabbas, F., Vincent, P., Ahmed, E. A., & Benmokrane, B. (2016). Experimental testing of basalt-fiber-reinforced polymer bars in concrete beams. Composites Part B: Engineering, 91, 205–218. https://doi.org/10.1016/j.compositesb.2016.01.045
- EN 14651. (2005). Test method for metallic fibred concrete — Measuring the flexural tensile strength (limit of proportionality (LOP), residual). British Standards Institute.
- EN 1991-1-1 (English): Eurocode 1: Actions on structures - Part 1-1: General actions - Densities, self-weight, imposed loads for buildings [Authority: The European Union Per Regulation 305/2011, Directive 98/34/EC, Directive 2004/18/EC]. 2002. The European Union Per Regulation.
- EN 1992-1-1 (English): Eurocode 2: Design of concrete structures - Part 1-1: General rules and rules for buildings [Authority: The European Union Per Regulation 305/2011, Directive 98/34/EC, Directive 2004/18/EC]. 2004. The European Union Per Regulation
- Fractionated quartz sand. http://www.batolit.ru/93_p.shtml
- Frazão, C., Barros, J., Toledo Filho, R., Ferreira, S., & Gonçalves, D. (2018). Development of sandwich panels combining sisal fiber-cement composites and fiber-reinforced lightweight concrete. Cement and Concrete Composites, 86, 206–223. https://doi.org/10.1016/j.cemconcomp.2017.11.008
- Galishnikova, V. V., Kharun, M., Koroteev, D. D., & Chiadighikaobi, P. C. (2021). Basalt fiber reinforced expanded clay concrete for building structures. Magazine of Civil Engineering, 101(1), 1-12. Article No. 10107. https://doi.org/10.34910/MCE.101.7.
- GOST 10180–2012. (2013). Concretes. Methods for strength determination using reference specimens. Moscow, 30. https://files.stroyinf.ru/Data2/1/4293782/4293782275.htm
- Grabois, T. M., Cordeiro, G. C., & Toledo Filho, R. D. (2016). Fresh and hardened-state properties of self-compacting lightweight concrete reinforced with steel fibers. Construction and Building Materials, 104, 284–292. https://doi.org/10.1016/j.conbuildmat.2015.12.060
- Hamad, A. J. (2014). Materials, production, properties and application of aerated lightweight concrete: Review. International Journal of Materials Science and Engineering, 2(2), 152–157. https://doi.org/10.12720/ijmse.2.2.152-157
- Karaburc, S. N., Yildizel, S. A., & Calis, G. C. (2020). Evaluation of the basalt fiber reinforced pumice lightweight concrete. Magazine of Civil Engineering, 94(2), 81–92. https://doi.org/10.18720/MCE.94.7
- Kim, Y.-J., Hu, J., Lee, S.-J., & You, B.-H. (2010). Mechanical properties of fiber reinforced lightweight concrete containing surfactant. Advances in Civil Engineering, 2010, 1–8. https://doi.org/10.1155/2010/549642. Article ID 5496422010.
- Kockal, N. U., & Ozturan, T. (2011). Durability of lightweight concretes with lightweight fly ash aggregates. Construction and Building Materials, 25(3), 1430–1438. https://doi.org/10.1016/j.conbuildmat.2010.09.022
- Komokhov, P. G. (2001). On Concrete of the 21st Century. Bulletin of RAASN, 5, 9–12.
- Kramar, D., & Bindiganavile, V. (2013). Impact response of lightweight mortars containing expanded perlite. Cement and Concrete Composites, 37, 205–214. https://doi.org/10.1016/j.cemconcomp.2012.10.004
- Li, S., Hu, J., & Ren, H. (2017). The combined effects of environmental conditioning and sustained load on mechanical properties of wet lay-up fiber reinforced polymer. Polymers, 9(12), 244. https://doi.org/10.3390/polym9070244
- Libre, N. A., Shekarchi, M., Mahoutian, M., & Soroushian, P. (2011). Mechanical properties of hybrid fiber reinforced lightweight aggregate concrete made with natural pumice. Construction and Building Materials, 25(5), 2458–2464. http://dx.doi.org/10.1016/j.conbuildmat.2010.11.058
- Lu, Z., Xian, G., & Li, H. (2015). Effects of exposure to elevated temperatures and subsequent immersion in water or alkaline solution on the mechanical properties of pultruded BFRP plates. Composites, Part B, 77, 421–430. https://doi.org/10.1016/j.compositesb.2015.03.066
- Ma, J., Qiu, X., Cheng, L., & Wang, Y. “Experimental research on the fundamental mechanical properties of presoaked basalt fiber concrete”, in CICE 2010—The 5th International Conference on FRP composites in civil engineering, pp. 1–4, Beijing, China, September 2010.
- Md, I., Sharmin, N. S., Md, M., & Akhtar, U. S. U. (2016). Effect of soda lime silica glass waste on the basic properties of clay aggregate. International Journal of Scientific and Engineering Research, 7(4), 149–154. https://www.ijser.org/researchpaper/Effect-of-Soda-lime-Silica-Glass-waste-on-the-basic-properties-of-Clay-Aggregate.pdf
- Mishra, A. K., & Chakraborty, S. (2016). Inverse detection of constituent level elastic parameters of FRP composite panels with elastic boundaries using finite element model updating. Ocean Engineering, 111, 358–368. https://doi.org/10.1016/j.oceaneng.2015.11.003
- Nishizaki, I., Sakuraba, H., & Tomiyama, T. (2015). Durability of pultruded gfrp through ten-year outdoor exposure test. Polymers, 7(12), 2494–2503. https://doi.org/10.3390/polym7121525
- Nizina, T. A., Balykov, A. S., Volodin, V. V., & Korovkin, D. I. (2017). Fiber fine-grained concretes with polyfunctional modifying additives. Magazine of Civil Engineering, 72(4), 73–83. https://doi.org/10.18720/MCE.72.9
- Nizina, T. A., Ponomarev, A. N., Balykov, A. S., & Pankin, N. A. (2017). Fine-grained fibre concretes modified by complexed nanoadditives. International Journal of Nanotechnology, 14(7–8), 665–667. https://doi.org/10.1504/IJNT.2017.083441
- Pan, Y., Xian, G., & Silva, M. A. G. (2015). Effects of water immersion on the bond behavior between CFRP plates and concrete substrate. Construction and Building Materials, 101(1), 326–337. https://doi.org/10.1016/j.conbuildmat.2015.10.129
- Posi, P., Teerachanwit, C., Tanutong, C., Limkamoltip, S., Lertnimoolchai, S., Sata, V., & Chindaprasirt, P. (2013). Lightweight geopolymer concrete containing aggregate from recycle lightweight block. Materials and Design, 52, 580–586. https://doi.org/10.1016/j.matdes.2013.06.001
- Ralegaonkar, R., Gavali, H., Aswath, P., & Abolmaali, S. (2018). Application of chopped basalt fibers in reinforced mortar: A review. Construction and Building Materials, 164, 589–602. https://doi.org/10.1016/j.conbuildmat.2017.12.245
- Sadik, A. Y., & Gokhan, C. (2019). Design and optimization of basalt fiber added lightweight pumice concrete using taguchi method. Revista Româna De Materiale/Romanian Journal of Materials, 49(4), 544–553. https://solacolu.chim.upb.ro/pg544-553.pdf
- Saha, A. K. (2018). Effect of class F fly ash on the durability properties of concrete. Sustainable Environment Research, 28(1), 25–31. https://doi.org/10.1016/j.serj.2017.09.001
- Sepehr, M. N., Kazemian, H., Ghahramani, E., Amrane, A. V., Sivasankar, V., & Zarrabi, M. (2014). Defluoridation of water via Light Weight Expanded Clay Aggregate (LECA): Adsorbent characterization, competing ions, chemical regeneration, equilibrium and kinetic modeling. Journal of the Taiwan Institute of Chemical Engineers, 45(4), 1821–1834. https://doi.org/10.1016/j.jtice.2014.02.009
- Shafigh, P., Hassanpour, M., Razavi, S. V., & Kobraei, M. (2011). An investigation of the flexural behaviour of reinforced lightweight concrete beams. International Journal of Physical Sciences, 6(10), 2414–2421. https://doi.org/10.5897/IJPS10.550
- Shariq, M., Prasad, J., & Abbas, H. (2013). Effect of GGBFS on age dependent static modulus of elasticity of concrete. Construction and Building Materials, 41, 411–418. https://doi.org/10.1016/j.conbuildmat.2012.12.035
- Silica Fume MK-85, Additive in concrete http://www.geogips.ru/catalog/cement_i_dobavki/plasticizer-accelerator/microsilica-mk-85_10kg/
- Slater, E., Moni, M., & Alam, M. S. (2012). Predicting the shear strength of steel fiber reinforced concrete beams. Construction and Building Materials, 26(1), 423–436. https://doi.org/10.1016/j.conbuildmat.2011.06.042
- The Pennsylvania State University. (2014). The effect of aggregate properties on concrete.
- Thomas, M. D. A. (2007). Optimizing the Use of Fly Ash in Concrete. Portland Cement Association, 24. https://www.academia.edu/7911232/Optimizing_the_Use_of_Fly_Ash_in_Concrete
- Wang, D., Ju, Y., Shen, H., & Xu, L. (2019). Mechanical properties of high-performance concrete reinforced with basalt fiber and polypropylene fiber. Construction and Building Materials, 197, 464–473. https://doi.org/10.1016/j.conbuildmat.2018.11.181
- Wang, Z. K., Zhao, X. L., Xian, G. J., Wu, G., Raman, R. K. S., Al-Saadi, S., & Haque, A. (2017). Long-term durability of Basalt- and Glass-Fibre Reinforced Polymer (BFRP/GFRP) bars in seawater and sea sand concrete environment. Construction and Building Materials, 139, 467–489. https://doi.org/10.1016/j.conbuildmat.2017.02.038
- Xian, G., & Karbhari, V. M. (2007). Segmental relaxation of water-aged ambient cured epoxy. Polymer Degradation and Stability, 92(9), 1650–1659. https://doi.org/10.1016/j.polymdegradstab.2007.06.015
- Zaetang, Y. Wongsa, A., Sata, V., Chindaprasirt, P., (2013). Use of lightweight aggregates in pervious concrete, Construction and Building Materials, 48, 585–591
- Zendehzaban, M., Sharifnia, S., & Hosseini, S. N. (2013). Photocatalytic degradation of ammonia by Light Expanded Clay Aggregate (LECA)-coating of TiO2 nanoparticles. Korean Journal of Chemical Engineers, 30(3), 574–579. https://doi.org/10.1007/s11814-012-0212-z
- Zhao, Q., Dong, J., Pan, H., & Hao, S. (2010). Impact behavior of basalt fiber reinforced concrete. Fuhe Cailiao Xuebao/Acta Materiae Compositae Sinica, 27(6), 120–125. https://www.researchgate.net/publication/288304151_Impact_behavior_of_basalt_fiber_reinforced_concrete
- Zheng, Y., Zhang, P., Cai, Y., Jin, Z., & Moshtagh, E. (2019). Cracking resistance and mechanical properties of basalt fibers reinforced cement-stabilized macadam. Composites Part B: Engineering, 165, 312–334. https://doi.org/10.1016/j.compositesb.2018.11.115