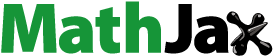
Abstract
Ribavirin is widely used in several types of combination chemotherapy for the treatment of chronic hepatitis C. In order to deliver ribavirin to the liver and reduce its side effects, we previously prepared ribavirin monophosphate (RMP)-loaded nanoparticles consisting of a mixture of poly(D,L-lactic acid), arabinogalactan-poly(L-lysine) conjugate, and iron (III) ions. In this study, the nanoparticles were developed in the absence of poly(L-lysine) to avoid cytotoxicity. The nanoparticles were successfully prepared from a mixture of poly(D,L-lactic acid) with terminal amino group(s) and arabinogalactan-poly(L-glutamic acid) conjugate. RMP was stably loaded in the nanoparticles and was gradually released from the nanoparticles under physiological conditions. Furthermore, high accumulation of the nanoparticles in hepatic cells was observed in vitro and in vivo. The nanoparticles also exhibited significantly lower cytotoxicity than that exhibited by those containing poly(L-lysine). Thus, the nanoparticles are expected to be useful in liver-specific ribavirin delivery with low cytotoxicity for the treatment of chronic hepatitis C.
Public Interest Statement
Chronic hepatitis mainly results from infection of hepatocytes with the hepatitis virus and is the major risk factor for the development of cirrhosis and hepatocellular carcinoma. Ribavirin exhibits anti-viral activity in hepatocytes infected with the hepatitis C virus and hepatitis E virus. Although ribavirin is essential to many of the beneficial effects of hepatitis treatments, clinical application of ribavirin is restricted because of ribavirin-induced hemolytic anemia. In order to avoid the side effects of ribavirin and improve its therapeutic effects, we have developed biodegradable nanoparticles as ribavirin carriers to accumulate ribavirin in the hepatocytes. The nanoparticles in our previous report showed high accumulation and long retention times of ribavirin in the livers of mice. However, there was concern that the nanoparticles caused tissue or cell injury because the nanoparticles were formed from cytotoxic polymers. In this study, we successfully developed novel liver-specific ribavirin-loaded nanoparticles without these cytotoxic polymers.
Competing interests
The authors declare no competing interest.
1. Introduction
Recently, various combination chemotherapies were approved for clinical treatment of chronic hepatitis C (Burstow et al., Citation2017; Nakamoto, Kanda, Shirasawa, & Yokosuka, Citation2015). Ribavirin, an anti-viral ribonucleoside analog, is one of the essential drugs in some combination chemotherapies, and it exhibits anti-viral activity in hepatocytes infected with the hepatitis C virus. Furthermore, it was reported that ribavirin as a monotherapy was effective in the treatment of chronic hepatitis E virus infection (Kamar et al., Citation2014). However, repeated administration of ribavirin frequently induces severe hemolytic anemia (Brochot et al., Citation2010) because it extensively accumulates in erythrocytes (Russmann, Grattagliano, Portincasa, Palmieri, & Palasciano, Citation2006).
While many researchers have been trying to synthesize novel ribavirin analogs to reduce hemolysis, substantial efforts have also been directed toward the modulation of the pharmacokinetics of ribavirin (Guo, Sun, Yang, Tang, & Wang, Citation2015). Ribavirin was covalently conjugated with carrier molecules such as poly(L-lysine) (Stefano et al., Citation1997), hemoglobin (Levy et al., Citation2006), bile acids (Dong, Li, Guo, & Polli, Citation2015), poly(vinyl pyrrolidone), and poly(acrylic acid) (Kryger, Smith, Wohl, & Zelikin, Citation2014). Some conjugates exhibited enhanced accumulation in the hepatic cells and reduced accumulation in erythrocytes. However, cleavage of the covalent bond between ribavirin and its carrier molecules in vivo is a determinant for exhibiting effective biological activity. Craparo, Teresi, Licciardi, Bondí, and Cavallaro (Citation2013) developed nanoparticles (micelles) which physically encapsulated a ribavirin derivative. In this system, chemically-modified ribavirin (ribavirin tripalmitate) was used as a hydrophobic prodrug because, in this form, the molecules could be easily encapsulated in the loci of solid particles through hydrophobic interactions. However, ribavirin tripalmitate would also need to be converted to ribavirin in vivo to exhibit its activity.
In our works, we focused on ribavirin monophosphate (RMP) as a drug. Ribavirin is intracellularly phosphorylated by cellular kinases to form a monophosphate, a diphosphate, and a triphosphate, which all exhibit anti-viral activities (Mori et al., Citation2013). Thus, it is expected that RMP directly functions as an active drug without chemical changes in vivo. Furthermore, the accumulation of RMP in erythrocytes may be suppressed because it is generally difficult for phosphorylated nucleosides to penetrate cell membranes (Hecker & Erion, Citation2008).
As the carrier for RMP, we focused on biodegradable solid nanoparticles. Solid particles are promising carriers for the stable retention of drugs, and particles consisting of biodegradable hydrophobic polymers can extend the therapeutic effect of drugs, because of their slow release as the polymers degrade (Ishihara & Mizushima, Citation2010). However, it is difficult to encapsulate hydrophilic molecules such as RMP in solid nanoparticles. In our previous report, RMP-loaded nanoparticles were prepared from a mixture of poly(D,L-lactic acid) homopolymer (PLA) and arabinogalactan-poly(L-lysine) conjugate (AG-PLL), in order to improve the therapeutic effect and safety of ribavirin (Ishihara, Kaneko, Ishihara, & Mizushima, Citation2014). RMP was physically and stably encapsulated in the nanoparticles by our unique technique involving the use of metal ions (Ishihara & Mizushima, Citation2010). In addition, RMP was gradually released with degradation of PLA, suggesting a long-term therapeutic effect. Arabinogalactan (AG), a polysaccharide with a galactan core and side chains of galactose and arabinose, is used as a ligand for liver-specific drug delivery (Elgart, Farber, Domb, Polacheck, & Hoffman, Citation2010; Kaneo et al., Citation2000; Park, Ishihara, Kano, Akaike, & Maruyama, Citation1999; Shah, Pathak, Jain, Barhate, & Nagarsenker, Citation2013), because it is well-known that proteins and polymers bearing galactose moieties selectively bind to the asialoglycoprotein receptor on the plasma membrane of hepatocytes and are internalized via receptor-mediated endocytosis (Pathak, Vyas, & Gupta, Citation2008; Poelstra, Prakash, & Beljaars, Citation2012). The PLL chain of the AG-PLL conjugate plays a role in anchoring AG chains on the surface of the nanoparticles through the ionic interaction between the amino groups of PLL and the terminal carboxyl groups of PLA (Figure (a)). However, polycationic materials (polycations) such as PLL, generally induce severe cytotoxicity in vitro and in vivo (Fischer, Li, Ahlemeyer, Krieglstein, & Kissel, Citation2003; Monnery et al., Citation2017; Parhamifar et al., Citation2014).
Figure 1. Ionic interactions of nanoparticle components. (a) A nanoparticle developed previously consisting of RMP, AG-PLL, and PLA with terminal carboxyl groups (PLA-C). (b) A nanoparticle developed in this study consisting of RMP, AG-PLG, and PLA with terminal amino groups (PLA-N1 or PLA-N3). The components in dotted line squares were cationic, and those in solid line squares were anionic. Allows mean ionic interactions. (c) Chemical structures of PLA, RMP, AG-PLL and AG-PLG.
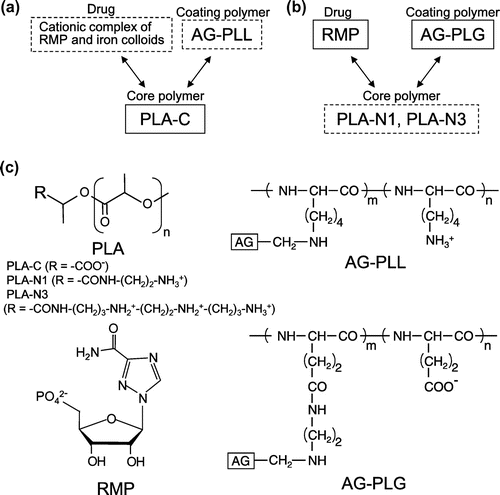
In this study, RMP-loaded nanoparticles modified with AG on their surfaces were prepared in the absence of polycations. PLA with terminal amino groups, and a conjugate between AG and poly(L-glutamic acid) (AG-PLG) were synthesized and used for the preparation of nanoparticles. We examined the cytotoxicity of the nanoparticles, their internalization into cultured cells, and their accumulation in mouse livers.
2. Materials and methods
2.1. Materials and animals
AG from larch wood, ethylenediamine anhydrous and N,N′-bis(3-aminopropyl)ethylenediamine were purchased from Tokyo Chemical Industry Co., Ltd. (Tokyo, Japan). Poly(l-lysine) (PLL) hydrobromide and sodium cyanoborohydride were purchased from Sigma-Aldrich (St. Louis, MO). Poly(l-glutamic acid) (PLG) sodium salt and 1-ethyl-3-(3-dimethylaminopropyl)carbodiimide monohydrochloride (EDC) were purchased from Peptide Institute, Inc. (Osaka, Japan). 1-(β-d-Ribofuranosyl)-1H-1,2,4-triazole-3-carboxamide (ribavirin) and 9-anthryldiazomethane (ADAM) were purchased from Funakoshi Co., Ltd. (Tokyo, Japan). Ribavirin 5′-monophosphate (RMP) was synthesized from ribavirin following a reported method (Allen et al., Citation1978). 2,4,6-Trinitrobenzenesulfonic acid sodium salt dehydrate (TNBS) was purchased from Wako Pure Chemicals Industries, Ltd. (Osaka, Japan).
Female ICR mice (6-week-olds) were obtained from CLEA Japan, Inc. (Tokyo, Japan). The mice were allowed free access to water and chow, and were housed under controlled environmental conditions (constant temperature, humidity, and a 12-h dark-light cycle). The experiments and procedures described here were carried out in accordance with the Guide for the Care and Use of Laboratory Animals as adopted and promulgated by the National Institutes of Health, and were approved by the Animal Care Committee of Nihon University on 23 August 2014 (AP14EN001).
2.2. Synthesis of arabinogalactan-poly(l-glutamic acid) conjugate (AG-PLG)
AG was purified with dialysis using a Spectra/Por7 dialysis membrane (MWCO: 1000, Spectrum Laboratories, Inc., Rancho Dominguez, CA) for 3 d, and recovered using lyophilization prior to use. AG with an amino group at the reducing terminal (AG-NH2) was synthesized with reductive amination of AG and ethylenediamine in the presence of sodium cyanoborohydride. Purified AG (3,000 mg), ethylenediamine (400 mg), and sodium cyanoborohydride (473 mg) were dissolved in 7.5 mL of water and the pH of the solution was adjusted to 3.7 by adding 6 M HCl. After incubation for 18 h at 40°C, the resulting products were precipitated seven times in an excess volume of ethanol with centrifugation at 20,000 × g for 10 min at 4°C. The products were further purified with dialysis against water using a Spectra/Por7 dialysis membrane (MWCO: 1000) for 4 d. Finally, AG-NH2 was obtained using lyophilization.
A conjugate of AG-NH2 and PLG (AG-PLG) was synthesized with condensation in the presence of EDC. Poly(l-glutamic acid) sodium salt (25 mg), AG-NH2 (200 mg), and EDC (80 mg) were dissolved in 5 mL of 0.1 M sodium phosphate buffer solution (pH 5.5). After incubation for 18 h at room temperature, the resulting conjugates were precipitated five times in an excess volume of ethanol with centrifugation at 20,000 × g for 10 min. The products were further purified using a Centriprep Centrifugal Filter Unit (YM-50, MWCO: 50,000, EMD Millipore Corp., Billerica, MA). Finally, AG-PLG was obtained via lyophilization. The molecular weights of AG-PLG, PLG, and AG-NH2 were determined using size-exclusion chromatography (SEC) on TSKgel α-4,000 and TSKgel G3000PWXL columns (Tosoh Co., Tokyo, Japan) coupled with a refractive index (RI) detector using an aqueous solution of 0.1 M sodium sulfate as the mobile phase. The instrument was calibrated with monodisperse pullulan standards (Showa Denko K.K., Tokyo, Japan).
2.3. Synthesis of poly(D,L-lactic acid) with terminal amino group(s)
Poly(D,L-lactic acid) with a carboxyl group on its terminal (PLA-C, Mw 7100, Mn 5500) was supplied from Taki Chemical Co., Ltd. (Kakogawa, Japan). Ethylenediamine or bis(aminopropyl)ethylenediamine was introduced into the PLA-C chain with condensation. PLA-C (750 mg), ethylenediamine (37.5 mg), and EDC (375 mg) were dissolved in 7.5 mL of DMSO. Separately, PLA (750 mg), bis(aminopropyl)ethylenediamine (109 mg), and EDC (750 mg) were dissolved in 7.5 mL of DMSO. The solutions were allowed to react for 5 h at 30°C with stirring. The resulting PLA-ethylenediamine (PLA-N1) or PLA-bis(aminopropyl)ethylenediamine (PLA-N3) was purified with precipitation in an excess volume of cold water and with lyophilization. The polymers were characterized using SEC using TSKgel G3000H column (Tosoh) with tetrahydrofuran (THF) as the mobile phase.
2.4. Preparation of nanoparticles
Nanoparticles were prepared using the oil-in-water solvent diffusion method as previously reported, with minor revisions (Ishihara et al., Citation2014). PLA (PLA-N1 or PLA-N3, 12.5 mg) was dissolved in 290 μL of DMSO. Then, 9.0 μL of an aqueous solution of 1.8 mg AG-PLG, 12.5 μL of an aqueous solution of 2.5 mg RMP, and 3 μL of 3 M HCl aqueous solution were sequentially added. The resulting mixture was allowed to stand for 10 min at room temperature. The mixture was added drop-wise to 3 mL of 0.2 mg/mL polysorbate 80 aqueous solution with continuous stirring at 700 rpm. Subsequently, 200 μL of sodium citrate aqueous solution (0.5 M, pH 7.2) was immediately added. The resulting nanoparticles were purified using a Minimate™ TFF capsule with a 300-kDa Omega membrane (Pall Co., Port Washington, NY) and condensed using a Centriprep Centrifugal Filter Unit (YM-50). Nanoparticles formed from PLA homopolymer alone without AG-PLG and AG-PLL were prepared similarly. Furthermore, rhodamine B-loaded nanoparticles were also prepared by adding a conjugate of PLA and rhodamine B, following a method previously reported (Ishihara et al., Citation2008).
2.5. Evaluation of nanoparticle characteristics
The particle size was determined using dynamic light scattering (Nano Partica SZ-100, HORIBA, Ltd., Kyoto, Japan). The zeta potential of the particles was also determined in 10 mM sodium phosphate buffer solution (pH 7.0) under a constant applied voltage (10 V).
The AG content in the nanoparticles was quantified using ethyl-4-aminobenzoate (Wako Pure Chemicals Industries) following a reported method (Yasuno, Murata, Kokubo, Yamaguchi, & Kamei, Citation1997). The RMP content and the PLA content in the nanoparticles were determined using HPLC as reported previously (Ishihara et al., Citation2014). In this study, nanoparticle weight was defined as the total PLA content in the nanoparticles. The loading efficiency of RMP in the nanoparticles was calculated as follows:
2.6. Release of ribavirin from nanoparticles
The release behavior of RMP from the nanoparticles was evaluated as reported previously (Ishihara et al., Citation2014). Briefly, the RMP-loaded nanoparticles were dispersed in phosphate-buffered saline (PBS, pH 7.4) at 37°C. After incubation for a defined period of time, the suspension was filtered with centrifugation (8,000 rpm, 30 min) using a Nanosep® Centrifugal Device equipped with an Omega Membrane (MWCO: 30 kDa, Pall Co.). The contents of ribavirin and RMP in the filtrate were determined using HPLC. The total amount of ribavirin and RMP detected in the filtrate was assumed to be the amount of RMP released from the nanoparticles, because the released RMP is converted to ribavirin by dephosphorylation during incubation in PBS. The total RMP content in the nanoparticles was determined as follows. An aliquot (100 μL) of the nanoparticle suspension was mixed with 300 μL of tetrahydrofuran (THF) to dissolve the PLA matrix. Afterward, 600 μL of sodium citrate aqueous solution (20 mM, pH 7.2) was added to solubilize the RMP. After centrifugation at 20,000 × g for 10 min, the RMP content in the supernatant was determined by HPLC. The mean amounts measured from three individual nanoparticle suspensions were normalized as the total RMP amount (100%) in the nanoparticles.
2.7. Uptake of nanoparticles in cultured cells
The interaction of the nanoparticles with the cells was evaluated as reported previously (Ishihara et al., Citation2014). Briefly, HepG2 cells (a human hepatocellular carcinoma cell line) were added to an 8-well Nunc Lab-Tek II Chambered Coverglass (Thermo Fisher Scientific, Inc. Waltham, MA) at 5 × 104 cells per well and incubated overnight at 37°C in Dulbecco’s Modified Eagle Medium (DMEM) containing 10% fetal bovine serum (FBS). The cells were incubated in 500 μL of Opti-MEM I Reduced Serum Medium (Opti-MEM, Life Technologies Corp., Carlsbad, CA) with rhodamine-loaded nanoparticles at a concentration of 0.1 mg/mL (rhodamine concentration: 0.4 μg/mL) for 3 h at 37°C. The cells were washed six times with PBS and were fixed with 2% paraformaldehyde solution. After nuclear staining with 5 μg/mL Hoechst 33,258 (Dojindo Laboratories, Kumamoto, Japan), the cells were observed using a fluorescence microscope equipped with a DS-Fi1c digital camera and a Nikon DS-L2 controller (Nikon Corp., Tokyo, Japan).
2.8. Cytotoxicity assay
HepG2 cells were added to a 96-well plate at 1.2 × 104 cells per well and incubated overnight at 37°C in DMEM containing 10% FBS. The cells were incubated with AG-PLG, AG-PLL, or various nanoparticles at 37°C in 100 μL of Opti-MEM without FBS or DMEM with 10% FBS. After incubation for 24 h, the cells were washed twice with PBS, and then cell viability was determined using a Cell Counting Kit-8 (Dojindo Laboratories). The mean number of cells in three individual untreated wells were normalized as the 100% viability value. The cells treated with nanoparticles at 2.5 mg/mL for 24 h were also observed using a microscope.
2.9. Animal experiments
The distribution of the nanoparticles in the mouse livers was examined. A suspension of rhodamine-loaded nanoparticles (PLG/N3-NP shown in Table ) or saline-dissolved rhodamine was intravenously administered to mice via the tail vein at a rhodamine dose of 4 μg/mouse. The liver, extracted 3 h after administration, was frozen in Tissue-Tek® O.C.T. Compound (Funakoshi). Unstained frozen sections (5-μm thick) were prepared at the Health Sciences Research Institute, Inc. (Yokohama, Japan) and observed using a fluorescence microscope.
Table 1. Molecular weights of polymers
3. Results and discussion
AG with an amino group at the reducing terminal (AG-NH2) was synthesized with reductive amination of AG and excess ethylenediamine. TNBS reacts with primary amino groups, resulting in trinitrophenylated products with a higher molar extinction coefficient at 340–350 nm (Satake, Okuyama, Ohashi, & Shinoda, Citation1960). The reaction of AG-NH2 or AG with TNBS was evaluated using SEC (Supplemental Figure S1). AG-NH2 showed a 350 nm absorbance peak at its retention time while AG did not, indicating AG-NH2 had an amino group. Furthermore, spectrophotometric determination of amino groups using TNBS showed that the modification ratio ([amino group] / [AG]) was 0.47. A conjugate of AG-NH2 and PLG was obtained with a coupling reaction in the presence of EDC. AG-NH2, PLG, and AG-PLG were analyzed using SEC (Supplemental Figure S2) and the average molecular weights of the polymers were calculated as shown in Table . The results also indicated that the conjugate was composed of 3.3 AG molecules and 1 PLG molecule.
PLAs with one or three amino group(s) at their terminal (PLA-N1 or PLA-N3) were synthesized via a reaction of ethylenediamine or bis(aminopropyl)ethylenediamine with a terminal carboxyl group in PLA-C, respectively. The carboxyl group content of each polymer was determined using 9-anthryldiazomethane (ADAM), which reacts with carboxyl groups without a catalyst (Nimura & Kinoshita, Citation1980). The synthesized polymers (PLA-N1 and PLA-N3) and PLA-C were reacted with 0.2 mg/ml of ADAM in acetonitrile for 3 h at room temperature, and the reactions were analyzed by SEC. The 366 nm absorbance peak derivatized with ADAM was detected at the retention time of PLA-C, indicating that ADAM was bound to a terminal carboxyl group of PLA-C. On the other hand, the peak areas of PLA-N1 and PLA-N3 were smaller than that of PLA-C as shown in Supplemental Figure S3. The results strongly suggested that the terminal carboxyl groups of PLA-N1 and PLA-N3 were modified with amino compounds. From the relative peak areas, the modification ratios of PLA-N1 ([ethylenediamine]/[PLA]) and PLA-N3 ([bisaminopropylethylenediamine]/[PLA]) were calculated as 0.77 and 0.60, respectively.
Nanoparticles were prepared with the oil-in-water solvent diffusion method using the PLAs (PLA-C, PLA-N1, or PLA-N3) as core polymers, the conjugates (AG-PLL or AG-PLG) as coating polymers, and RMP (Figure ). In our previous work, a nanoparticle was successfully developed consisting of RMP, AG-PLL, and PLA with terminal carboxyl groups (PLA-C) (Ishihara et al., Citation2014). PLA-C shows ionic interactions with both AG-PLL and the cationic complex between RMP and iron (III) colloids, resulting in the encapsulation of RMP in the nanoparticles and the coating of AG-PLL on the nanoparticles’ surfaces (Figure (a)). In this study, we attempted to prepare nanoparticles without AG-PLL, to avoid the high cytotoxicity of PLL. The nanoparticles were prepared from RMP, PLA-N1 (or PLA-N3), and AG-PLG. In the nanoparticles, the terminal amino group(s) of PLA-N1 (or PLA-N3) showed ionic interactions with the PLG chain of AG-PLG and RMP (Figure (b)). This method of loading RMP into the nanoparticles is simpler, because iron (III) colloids were not required.
As shown in Table , the zeta potential of the nanoparticles prepared from PLA-C alone without any coating polymers showed a large negative value (−43.4 mV), due to the carboxyl groups of PLA-C chains on the nanoparticles’ surfaces. On the other hand, the zeta potentials of None/N1-NP and None/N3-NP were neutral (−0.1 mV) and moderately positive (+5.1 mV), respectively, probably due to the amino groups on PLA. The zeta potentials of PLG/N1-NP and PLG/N3-NP were lower than those of the nanoparticles without coating polymers, indicating that AG-PLG was coated on the nanoparticle surface. Determination of saccharide showed that the AG content in PLG/N1-NP and PLG/N3-NP was approximately 10% (w/w). As shown in supplemental Figure S4, the nanoparticles were spherical and their sizes almost matched those measured by dynamic light scattering. The loading efficiency of RMP in PLG/N3-NP was 0.6% (w/w), while that in PLG/N1-NP was 0.1% (w/w), probably due to the larger number of amino groups in the PLG/N3-NP. On the other hand, the loading efficiency of RMP in the PLL/C-NP was slightly higher than that in the PLG/N3-NP. RMP was not encapsulated in the nanoparticles prepared from AG-PLG and PLA-C, indicating that a determining factor for RMP loading is the ionic interactions of RMP with PLA-N1 and PLA-N3.
Table 2. Nanoparticle characteristics
Solid particles formed from biodegradable polymers can result in long-term therapeutic effects through the slow release of the drugs as the polymers degrade. The release behavior of RMP from PLG/N3-NP dispersed in PBS at 37°C was evaluated, and showed a gradual release of RMP without a large initial burst (Figure ). However, PLG/N3-NP released RMP faster than the PLL/C-NP containing iron ions reported in our previous article (Ishihara et al., Citation2014). We have previously prepared many types of nanoparticles containing acidic drugs in the presence of metal ions such as zinc and iron (Ishihara et al., Citation2008, Citation2014; Ishihara & Mizushima, Citation2010). Nanoparticles with metal ions tend to release drugs slower than those without metal ions, possibly because of the strong interactions of acidic drugs with excess metal ions in the nanoparticles. These acidic drugs in the nanoparticles were released synchronously with PLA degradation (Ishihara & Mizushima, Citation2010), suggesting that drugs, including RMP, are released by the surface erosion of the PLA matrix of the nanoparticle.
Figure 2. Release behavior of RMP from PLG/N3-NP.
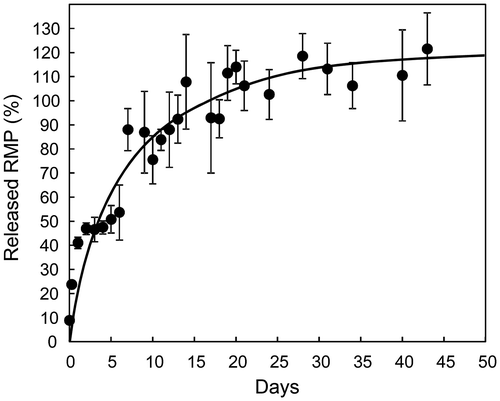
Although AG is a well-known ligand molecule for liver-specific drug delivery, the structure and composition of AG is different depending on its origin. Initially, we attempted to verify its interaction with hepatic cells in vitro. As shown in supplemental Figure S5, FITC-labeled AG was apparently internalized in the HepG2 cells while FITC-labeled bovine serum albumin was not. Thus, it was found that the AG molecule was a useful ligand to hepatic cells. Next, the interactions of rhodamine-loaded nanoparticles with hepatic cells were investigated. PLG/N1-NP and PLG/N3-NP exhibited higher internalization by cells than None/N1-NP did, as shown in Figure . The results also showed that the nanoparticles were mainly distributed in the cytoplasm. These results suggested that the nanoparticles were internalized via receptor-mediated endocytosis. On the other hand, None/N3-NP was internalized by the cells despite the absence of AG. This may be due to an ionic interaction between the cells and nanoparticles with surface positive charges.
Figure 3. Uptake of rhodamine-loaded nanoparticles in HepG2 cells.
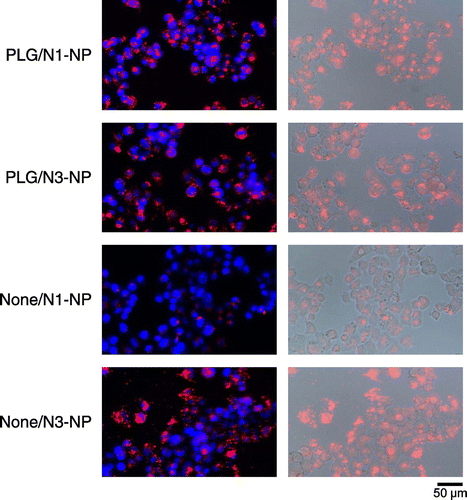
Next, the cytotoxicity of the conjugates against HepG2 cells was examined. AG-PLL caused high cytotoxicity even in culture medium with FBS, suggesting severe tissue/cell injury in vivo, while AG-PLG did not (Figure ). The mechanism of cytotoxicity of polycations is poorly understood, but it may be related to necrotic cell membrane damage (Fischer et al., Citation2003). In general, polycations with higher molecular weights show higher cytotoxicity (Monnery et al., Citation2017). Thus, it may be possible to reduce cytotoxicity using a PLL conjugate with a smaller molecular weight. However, unstable anchoring of the AG chain on the nanoparticle surface because of weaker interactions of the conjugate with PLA-C may be a concern.
Figure 4. Cytotoxicity of conjugates.
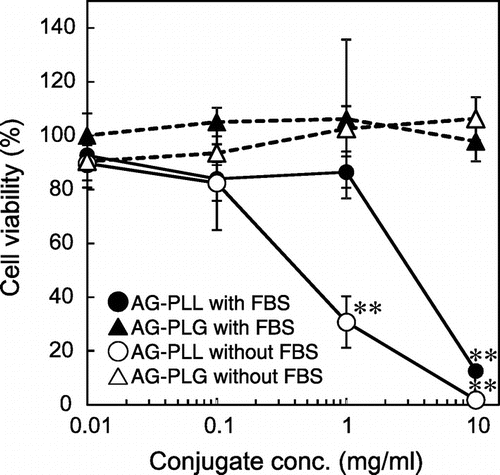
As shown in Figure (a), the nanoparticles containing AG-PLL (PLL/C-NP) also caused high cytotoxicity at high concentrations. The density of the cells incubated with PLL/C-NP was lower than that of cells incubated with PLG/N3-NP, and the plasma membrane of the cells appeared to be damaged (Figure (b)). The cytotoxicity may be caused by AG-PLL released from the nanoparticles and/or AG-PLL localized on the outer surfaces of the nanoparticles, because most of the AG-PLL was neutralized with the carboxyl groups of PLA-C in the nanoparticles. The high cytotoxicity of PLL/C-NP limits their clinical use for the treatment of hepatitis C. On the other hand, PLG/N1-NP and PLG-N3-NP did not exhibit any cytotoxicity, indicating the successful development of nanoparticles with low cytotoxicity.
Figure 5. Cytotoxicity of nanoparticles. After incubation of HepG2 cells with various nanoparticles in Opti-MEM for 24 h, (a) cell viability was determined, or (b) the cells were observed using a microscope. Each data point represents the mean ± SD of measurements from three individual wells.
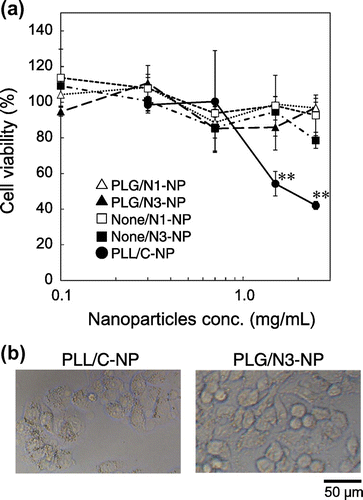
The distribution of the nanoparticles in the liver of mice was also examined. The rhodamine-loaded PLG/N3-NP were intravenously administered to mice and frozen liver sections were observed (Figure ). High accumulation and extensive distribution of the nanoparticles in the liver was observed. This result suggested the localization of the nanoparticles to hepatocytes.
4. Conclusions
In this study, nanoparticles coated with AG were developed for liver-specific ribavirin delivery with low cytotoxicity. The nanoparticles were prepared using AG-PLG, RMP, and PLA with the amino groups (PLA-N1 or PLA-N3). PLG/N3-NP showed higher loading efficiency of RMP than PLG/N1-NP, and released RMP gradually under physiological conditions. The in vitro cytotoxicity of the nanoparticles was low, and they accumulated in the liver of mice. Taken together, the nanoparticles are expected to show high biological activity with reduced side effects in the treatment of chronic hepatitis C. Furthermore, the strategy of using AG-coated nanoparticles for loading drugs may be applied to the treatment of various hepatic diseases.
Funding
This study was supported by a Grant-in-Aid for Scientific Research [grant number 22500410] from the Japan Society for the Promotion of Science.
Supplemental data
Supplemental data for this article can be accessed at https://doi.org/10.1080/2331205X.2017.1418133.
OAMD_1418133_Supplementary_Material.zip
Download Zip (1.9 MB)Acknowledgements
The authors thank Sayuri Ohuchi and Fumie Hashimoto for their technical assistance with the experiments.
Additional information
Notes on contributors
Kohei Kaneko
The research activities of our group are focused on delivery systems for drugs. We have directed substantial effort toward the development of carriers for low-molecular-mass drugs, peptides and poly(oligo)nucleotides (genes and siRNA), including polymeric solid particles, synthetic polymers, and lipid emulsions, to expand the utility of drugs for various clinical applications. As reported in this manuscript, we take a special interest in solid particles made from biodegradable polymers such as poly(lactic acid). Using our unique techniques of preparation, the particles, which have multiple functions such as stable drug retention, accumulation at the target lesion, sustained release of drugs at the target lesion, and further biological activity in vivo, have been developed. Our team has also clarified that the particles could be used in combination with various drugs such as steroids, prostaglandins, an immunosuppressive drug, anticancer drugs, and poly(oligo)nucleotides.
References
- Allen, L. B., Boswell, K. H., Khwaja, T. A., Meyer, Jr, R. B., Sidwell, R. W., Witkowski, J. T., … Robins, R. K. (1978). Synthesis and antiviral acticity of some phosphates of the broad-spectrum antiviral nucleoside, 1-beta-D-ribofuranosyl-1,2,4-triazole-3-carboxamide (ribavirin). Journal of Medicinal Chemistry, 21, 742–746.10.1021/jm00206a005
- Brochot, E., Castelain, S., Duverlie, G., Capron, D., Nguyen-Khac, E., & François, C. (2010). Ribavirin monitoring in chronic hepatitis C therapy: Anaemia versus efficacy. Antiviral Therapy, 15, 687–695.10.3851/IMP1609
- Burstow, N. J., Mohamed, Z., Gomaa I, A. I., Sonderup, M. W., Cook, N. A., Waked, I., … Taylor-Robinson, S. D. (2017). Hepatitis C treatment: Where are we now? International Journal of General Medicine, 10, 39–52.10.2147/IJGM
- Craparo, E. F., Teresi, G., Licciardi, M., Bondí, M. L., & Cavallaro, G. (2013). Novel composed galactosylated nanodevices containing a ribavirin prodrug as hepatic cell-targeted carriers for HCV treatment. Journal of Biomedical Nanotechnology, 9, 1107–1122.10.1166/jbn.2013.1608
- Dong, Z., Li, Q., Guo, D., Shu, Y., Polli, J. E. (2015). Synthesis and evaluation of bile acid-ribavirin conjugates as prodrugs to target the liver. Journal of Pharmaceutical Sciences, 104, 2864–2876.10.1002/jps.24375
- Elgart, A., Farber, S., Domb, A. J., Polacheck, I., & Hoffman, A. (2010). Polysaccharide pharmacokinetics: Amphotericin B arabinogalactan conjugate—A drug delivery system or a new pharmaceutical entity? Biomacromolecules, 11, 1972–1977.10.1021/bm100298r
- Fischer, D., Li, Y., Ahlemeyer, B., Krieglstein, J., & Kissel, T. (2003). In vitro cytotoxicity testing of polycations: Influence of polymer structure on cell viability and hemolysis. Biomaterials, 24, 1121–1131.10.1016/S0142-9612(02)00445-3
- Guo, H., Sun, S., Yang, Z., Tang, X., & Wang, Y. (2015). Strategies for ribavirin prodrugs and delivery systems for reducing the side-effect hemolysis and enhancing their therapeutic effect. Journal of Controlled Release, 209, 27–36.10.1016/j.jconrel.2015.04.016
- Hecker, S. J., & Erion, M. D. (2008). Prodrugs of phosphates and phosphonates. Journal of Medicinal Chemistry, 51, 2328–2345.10.1021/jm701260b
- Ishihara, T., Kaneko, K., Ishihara, T., & Mizushima, T. (2014). Development of biodegradable nanoparticles for liver-specific ribavirin delivery. Journal of Pharmaceutical Sciences, 103, 4005–4011.10.1002/jps.24219
- Ishihara, T., & Mizushima, T. (2010). Techniques for efficient entrapment of pharmaceuticals in biodegradable solid micro/nanoparticles. Expert Opinion on Drug Delivery, 7, 565–575.10.1517/17425241003713486
- Ishihara, T., Takahashi, M., Higaki, M., Takenaga, M., Mizushima, T., & Mizushima, Y. (2008). Prolonging the in vivo residence time of prostaglandin E1 with biodegradable nanoparticles. Pharmaceutical Research, 25, 1686–1695.10.1007/s11095-008-9549-8
- Kamar, N., Izopet, J., Tripon, S., Bismuth, M., Hillaire, S., Dumortier, J., … Mallet, V. (2014). Ribavirin for chronic hepatitis E virus infection in transplant recipients. New England Journal of Medicine, 370, 1111–1120.10.1056/NEJMoa1215246
- Kaneo, Y., Ueno, T., Tanaka, T., Iwase, H., Yamaguchi, Y., & Uemura, T. (2000). Pharmacokinetics and biodisposition of fluorescein-labeled arabinogalactan in rats. International Journal of Pharmaceutics, 201, 59–69.10.1016/S0378-5173(00)00405-1
- Kryger, M. B., Smith, A. A., Wohl, B. M., & Zelikin, A. N. (2014). Macromolecular prodrugs for controlled delivery of ribavirin. Macromolecular Bioscience, 14, 173–185.10.1002/mabi.201300244
- Levy, G. A., Adamson, G., Phillips, M. J., Scrocchi, L. A., Fung, L., Biessels, P., … Bell, D. (2006). Targeted delivery of ribavirin improves outcome of murine viral fulminant hepatitis via enhanced anti-viral activity. Hepatology, 43, 581–591.10.1002/(ISSN)1527-3350
- Monnery, B. D., Wright, M., Cavill, R., Hoogenboom, R., Shaunak, S., Steinke, J. H., & Thanou, M. (2017). Cytotoxicity of polycations: Relationship of molecular weight and the hydrolytic theory of the mechanism of toxicity. International Journal of Pharmaceutics, 521, 249–258.10.1016/j.ijpharm.2017.02.048
- Mori, K., Hiraoka, O., Ikeda, M., Ariumi, Y., Hiramoto, A., Wataya, Y., & Kato, N. (2013). Adenosine kinase is a key determinant for the anti-HCV activity of ribavirin. Hepatology, 58, 1236–1244.10.1002/hep.v58.4
- Nakamoto, S., Kanda, T., Shirasawa, H., & Yokosuka, O. (2015). Antiviral therapies for chronic hepatitis C virus infection with cirrhosis. World Journal of Hepatology, 7, 1133–1141.10.4254/wjh.v7.i8.1133
- Nimura, N., & Kinoshita, T. (1980). Fluorescent labeling of fatty acids with 9-anthryldiazomethane (ADAM) for high performance liquid chromatography. Analytical Letters, 13, 191–202.10.1080/00032718008082552
- Parhamifar, L., Andersen, H., Wu, L., Hall, A., Hudzech, D., & Moghimi, S. M. (2014). Polycation-mediated integrated cell death processes. Advances in Genetics, 88, 353–398.
- Park, J. U., Ishihara, T., Kano, A., Akaike, T., & Maruyama, A. (1999). Preparation of dendritic graft copolymer consisting of poly-(L-lysine) and arabinogalactan as a hepatocyte specific DNA carrier. Preparative Biochemistry and Biotechnology, 29, 353–370.10.1080/10826069908544934
- Pathak, A., Vyas, S. P., & Gupta, K. C. (2008). Nano-vectors for efficient liver specific gene transfer. International Journal of Nanomedicine, 3, 31–49.
- Poelstra, K., Prakash, J., & Beljaars, L. (2012). Drug targeting to the diseased liver. Journal of Controlled Release, 161, 188–197.10.1016/j.jconrel.2012.02.011
- Russmann, S., Grattagliano, I., Portincasa, P., Palmieri, V. O., & Palasciano, G. (2006). Ribavirin-induced anemia: Mechanisms, risk factors and related targets for future research. Current Medicinal Chemistry, 13, 3351–3357.10.2174/092986706778773059
- Satake, K., Okuyama, T., Ohashi, M., & Shinoda, T. (1960). The spectrophotometric determination of amine, amino acid and peptide with 2, 4, 6-trinitrobenzene 1-sulfonic acid. The Journal of Biochemistry, 47, 654–660.10.1093/oxfordjournals.jbchem.a127107
- Shah, S. M., Pathak, P. O., Jain, A. S., Barhate, C. R., & Nagarsenker, M. S. (2013). Synthesis, characterization, and in vitro evaluation of palmitoylated arabinogalactan with potential for liver targeting. Carbohydrate Research, 367, 41–47.10.1016/j.carres.2012.11.025
- Stefano, G., Colonna, F. P., Bongini, A., Busi, C., Mattioli, A., & Fiume, L. (1997). Ribavirin conjugated with lactosaminated poly-L-lysine: Selective delivery to the liver and increased antiviral activity in mice with viral hepatitis. Biochemical Pharmacology, 54, 357–363.10.1016/S0006-2952(97)00223-2
- Yasuno, S., Murata, T., Kokubo, K., Yamaguchi, T., & Kamei, M. (1997). Two-mode analysis by high-performance liquid chromatography of ρ-aminobenzoic ethyl ester-derivatized monosaccharides. Bioscience, Biotechnology, and Biochemistry, 61, 1944–1946.10.1271/bbb.61.1944