Abstract
Amphibians are believed to be sensitive to hypoxia, and in terms of this ability, they are between mammals with poor toleranсе to hypoxia and some turtles and fish with good tolerance to variable oxygen availability. However, we found that the Pallas’ spadefoot, Pelobates vespertinus (Pallas, 1771), a Eurasian anuran burrowing amphibian species, is capable of surviving in harsh hypoxia during wintering. This species can tolerate over long-term vital oxygen concentration of almost 10 times lower than the normal atmospheric air concentration (about 2%). The lethal oxygen concentration was about 1% (from 0.6 to 1.8% for different individuals), i.e. 20 times lower than the atmospheric concentration. The Pallas’ spadefoots overwinters in soils for more than half a year, having small sizes of storage organs (fat bodies and liver) and low content of lipids and glycogen in them, which are consumed at a low rate in normoxia at 3°С. Under hypoxic conditions, lipid consumption stops, and glycogen consumption increases, indicating a shift from aerobic to anaerobic metabolism. The cumulative effect of the reduced metabolic rate due to low wintering temperatures and the activation of glycolysis allows the Pallas’s spadefoot to be considered as one of the most hypoxia-resistant burrowing amphibian species studied.
Introduction
Most terrestrial wintering anuran amphibian species use only the first few centimeters of litter and soil, cavities or burrows of other animals for hibernation or aestivation. However, there are species of frogs and toads that regularly burrow tens of centimeters or even 2 meters on their own to escape cold winters, hot summers, or long dry seasons (Wells Citation2007), and are likely to be exposed at a depth to others extreme conditions. Due to their cryptic behavior, the environmental conditions at the hibernation/aestivation depth of these species (temperature, humidity, gas composition) and their adaptation to possible extreme factor values are poorly understood.
For deep-burrowing species, the depth of aestivation/hibernation may predetermine the lack of oxygen in the soil (hypoxia) and the excess of carbon dioxide (hypercapnia). Probably for this reason, as well as because of the texture of soils that ensure ease of burrowing, many species prefer light soil types – sandy and sandy clay, or broken soil (Seymour Citation1973a, Citation1973b; Belik Citation2010; Debelo & Chibilev Citation2013; Yermokhin et al. Citation2013). In such soils, gas diffusion is usually intense, and soil air, even in deep horizons, can be close in composition to atmospheric air (Seymour Citation1973a, Citation1973b; Withers Citation1978; Smagin Citation2005; Carvalho et al. Citation2010). However, the diffusion of gases can be hindered by layers of denser materials, such as loam, or freezing of soils from above, reaching a depth of more than 1.5 m in cold regions (Withers Citation1978; Ultsch & Anderson Citation1986; Berman et al. Citation2020; Bulakhova et al. Citation2020). Another factor that can lead to the occurrence of oxygen deficiency at the depth of hibernation is surface flooding, in which water penetrating into the soil and ground displaces soil air ahead of the wetting front (Lavelle & Spain Citation2001).
It was believed that amphibians are sensitive to hypoxia and, and in terms of this ability, they are between mammals with poor tolerance to hypoxia and some turtles and fish with good tolerance to variable oxygen availability; while the attitude to oxygen deficiency was studied mainly for amphibian species hibernating in water (Bickler & Buck Citation2007; Tattersall & Ultsch Citation2008). Recently was described the phenomenon of the Siberian frog, which overwinters for several months in water bodies with almost complete anoxia due to the transition to anaerobic metabolism (Berman et al. Citation2017, Citation2019; Shekhovtsov et al. Citation2020). In deep-burrowing amphibians, respiration, as well as the gas composition in soils during wintering/aestivation, is poorly understood (Seymour Citation1973a, Citation1973b; Wang et al. Citation1994; Glass et al. Citation1998; Pörtner et al. Citation1991; McAneney et al. Citation2006 and others). This is partly due to the fact that instrumental studies of the gas composition deep in the soil in nature are difficult (unless amphibians overwinter in cavities or burrows). Therefore, the one way of studying the reaction of amphibians to hypoxia is via laboratory studies of the physiological capabilities of animals. The results obtained in the laboratory – the ability to endure hypoxia for a short time (several minutes or hours) by reducing the metabolic rate to save limited energy resources, or using the transition to anaerobic metabolism, as well as the special choice of certain species of hibernacula with a reduced oxygen content to reduce the metabolic rate during hibernation or aestivation – are very important for understanding animal adaptations to extreme environmental conditions (Armentrout & Rose Citation1971; Pinder et al. Citation1992; Bickler & Buck Citation2007; Rossi et al. Citation2020).
Indirect evidence of the environmental conditions that amphibians may encounter during wintering may be the dynamics of their storage organs and reserve substances. Wintering and estivation can last several months, and animals must be provided with reserve substances to maintain life during this time. Before wintering and estivation, a greater conversion of food to glycogen and lipid reserves causes a large increase in the sizes of the liver and fat bodies, and during the dormancy, reserves are reduced (Pinder et al. Citation1992). Species vary in the patterns of lipid and glycogen storage. In burrowing amphibians, the lipids are considered to be the main metabolic fuel during aestivation/hibernation for maintaining a wholly aerobic metabolism, which is confirmed by seasonal changes in their reserves in the body and measurements of respiratory coefficients (see Feder & Burggren Citation1992). Lipids are stored in various tissues (liver, muscles, subcutaneous deposits, etc.), but their main depots in burrowing amphibians are abdominal fat bodies, which reach a significant size (more 3% of body mass) compared to those in amphibian species wintering in water (less 1% of body mass) (Smith Citation1950; Bush, Citation1963; Pasanen & Koskela Citation1974; Pinder et al. Citation1992). In contrast, glycogen in liver and muscle is a key resource for winter fuel requirements in water or terrestrial hibernating amphibians (Kato Citation1910; Bleibtreu Citation1911; Smith Citation1950; Mizell Citation1965; Pasanen & Koskela Citation1974; Schlaghecke & Blom Citation1978; Berman et al. Citation1984; Storey & Storey Citation1984; Irwin & Lee Citation2003; Costanzo et al. Citation2015; Bulakhova & Shishikina Citation2022). Glycogen also serves as the main reserve of amphibians in case of hypoxic conditions, because lipids cannot be used efficiently without available oxygen (Donohoe & Boutilier, Citation1998; Bickler & Buck Citation2007; Tattersall & Ultsch Citation2008; Shekhovtsov et al. Citation2020).
Burrowing amphibians of temperate latitudes, wintering for many months deep in the ground due to low negative air temperatures and freezing of the soil, are particularly promising for studying the attitude to hypoxia. In northern Eurasia, such animals include some species of toads (Bufo and Strauchbufo) and spadefoots (Pelobates), including Pelobates vespertinus (Pallas, 1771). The winter ecology of this species has been very little studied so far (Yermokhin et al. Citation2013; Berman et al. Citation2019a; Bulakhova et al. Citation2020). Meanwhile, it inhabits cold areas where deep freezing of the ground forces it to winter at a depth of more than 1.5 m (Berman et al. Citation2020; Bulakhova et al. Citation2020).
The first objective of this study was to determine, via a laboratory experiment, the minimal vital concentration of oxygen in the air at which P. vespertinus can survive over short (during a day) and long term (at least for a month) exposure. That is, to find out the range of possibilities of P. vespertinus in relation to hypoxia – from lethal threshold to the extreme but safe hypoxia. The second goal is to study the type (lipids or glycogen) and stock of energy resources as indirect evidence of preferred conditions during hibernation and their consumption with different oxygen content in the air.
Material and methods
Animals care
Pelobates vespertinus (n = 32, body mass, P = 11.1 ± 0.5 g, and body length, SVL = 41.6 ± 1.3 mm) were collected in mid-September 2020 near the village of Uritskoye (Saratov region, 51°25`N, 44°56`E) in the Medveditsa river valley. The animals were transported in a thermostated container with soil to the Institute for experiments. In the laboratory, the animals in several ventilated 3-liter containers with soil with a moisture content of 35–40% were placed in thermostats (ТСО-1/80, Smolensk SKTB SPU, Russia). The thermostats were additionally thermally insulated from the outside to reduce gradients, as a result of which the maximum deviation of the average temperature in the thermostat was reduced from the set one (±1.5°С according to the passport) to ±0.5°C (measurements were controlled by a calibrated temperature loggers, iButton DS1922L, Scientific and Technical Laboratory “ElIn”, Moscow, Russia). Pre-winter acclimation was carried out: temperature was lowered stepwise – two days at 15, at 10 and at 8°C, two weeks at 5°C and then - at 3°C (). At a temperature of 3°C, which is typical for wintering of the Pallas’ spadefoot in nature (Yermokhin et al. Citation2013), the animals were kept in the experiments and in the control. Experimental protocols were approved by the Bioethics Committee of the Institute of Biological Problems of the North (Permission No. 001/021).
Figure 1. Scheme of acclimation, stay in the control and in the main experiments of the spadefoots Pelobates vespertinus. The red line – oxygen content, the blue line – temperature. Exp-I and Exp-II - average duration of stay of the experimental groups under hypoxic conditions; the black circles - sampling of animals for biochemical analyses.
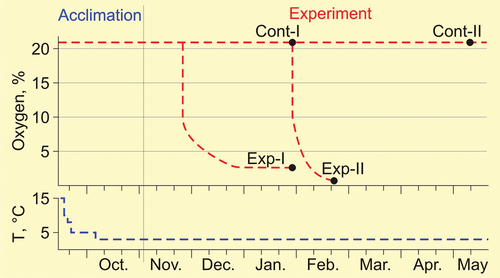
Preliminary experiments and equipment
After acclimation, to develop experimental protocols, two compact preliminary experiments were carried out on 6 individuals, which were not later used in the main experiments. The first experiment was carried out to test the possibility of reducing the duration of the experiment - a rough estimate of the reduced oxygen concentration at which the behavior of the spadefoots would not be inhibited; the second - to determine near-lethal concentration and reversibility of coma in the spadefoots. In all preliminary and main experiments, one spadefoot was placed into 800 ml glass vessel chamber (Duran Group, DWK Life Science, Germany). Hermetic lids of vessels have four gas passages, which were plugged with soft silicone stoppers and closed with plastic screw caps. Moist filter paper was placed in the chamber to maintain high air humidity, and a carbon dioxide sorbent formed during the respiration of animals (soda lime - Sofnolime 2550, Molecular Products Limited, UK) was placed to prevent hypercapnia.
All individuals (n = 6) from containers with normal oxygen content were placed in vessel chambers with an oxygen concentration of 10%, i.e. two times lower than the atmospheric norm (the required oxygen content was achieved by slowly purging experimental vessels-chambers with spadefoots with gaseous nitrogen). The choice of this concentration was based on data from the experiments of Rossi et al. (Citation2020) on striped burrowing frog (Cyclorana alboguttata, Hylidae), which did not show signs of depression state under 10% oxygen concentration.
The content of oxygen and carbon dioxide in the vessels was measured with a MultiGas OxyPro O2/CO2 gas analyzer (HTK Hamburg GmbH, https://www.htk-hamburg.com/) once every two days. Air was sampled with a gas analyzer needle through a silicone plug puncture. The volume of a single sample is 20 ml; the measurement accuracy of the calibrated instrument is ±0.5%. Before each measurement session, the O2 sensor was calibrated for atmospheric air and pure N2 (GOST 99.9%); CO2 sensor – for pure CO2 (GOST 99.8%) and N2 (GOST 99.9%). The content of gases in the chamber air was calculated as the average of three repeated measurements. To compensate for the pressure in the chamber (as a result of the removal of 60 ml of air), prepared air with the required proportion of oxygen, which was obtained by mixing nitrogen and atmospheric air in the required proportion, was pumped into it through a silicone stopper with a syringe.
The state of the spadefoots in the all experiments was assessed once a day by the adequacy of their motor responses to lighting, camera tilt, or to the touch of a thin wire inserted through a silicone plug.
The spadefoots stayed at 10% oxygen for two weeks and showed no anxiety, were in normal postures, and responded adequately to stimuli. Then the four animals were transferred to a concentration of 5% oxygen for two weeks; their behavior also did not differ from normal. The other two spadefoots were left under conditions of gradually decreasing oxygen concentration due to respiration until coma was reached (muscles were relaxed, pupils did not react to light, buccopharyngeal movements were absent). Coma occurred on days 15 and 16 at oxygen concentrations of 1.9 and 0.7%. After 18–20 h of coma, the spadefoots were placed to a normal oxygen concentration and then they came to life. All of these six individuals lived in the laboratory for several weeks after the experiments were completed.
The results of the preliminary experiments made it possible to model the scheme of the main experiments. They allowed to start the main experiments not with atmospheric oxygen concentration, but with 10% and to consider a minimum vital oxygen concentration of approximately 2–3% (the average between a well-tolerated concentration of 5% and an average near lethal one).
Main experiments
The ability of spadefoots (n = 7) to stay for a long time (at least for a month) in conditions of extremely low (about 10 times lower than normal) oxygen content (2–3%) was determined in the first experiment (Experiment-I). From the time of capture they were in normoxia, then they were transferred to chamber vessels with an oxygen content of 10%, which decreased due to the respiration of animals to 2–3% and was maintained (by adding a gas mixture) on this level until the end of January (), after which six animals (sample “exp-I”) were pitted for biochemical analyzes ().
Table I. Body length (SVL) and body mass (P) of Pelobates vespertinus used for biochemical analyzes.
In the second experiment (Experiment-II) exact near-lethal oxygen concentrations were clarified with a short stay in which spadefoots (n = 6) went into coma. The animals were in normoxic conditions until the end of January (almost 4.5 months after capture), and then they were transferred to vessels with 10% oxygen. The oxygen content decreased, due to the respiration of animals, to near lethal concentrations – until the onset of coma. Three of these six animals were pitted for biochemical studies (sample “exp-II”) (, ).
Biochemical assays
The consumption of reserve substances was studied in individuals in two experimental (“exp-I”, n = 6 and “exp-II”, n = 3) and two control samples consisting only of adult females, since it is known that in many amphibian species there are age and sex differences in the absolute and relative sizes of storage organs and the content of reserve substances (Pasanen & Koskela Citation1974; Morton Citation1981; Yermokhin et al. Citation2014; Bulakhova & Shishikina Citation2022).
Control animals hibernated in a thermostat in soil at 3°C and with normal oxygen content from late September to late January (n = 9, first control group, “cont-I”, pitted for biochemical studies simultaneously with animals “exp-I’) and until mid-May (n = 4, second control group, “cont-II”) (, ). At autopsy, it turned out that one of the females in the first control group, despite its large size, was immature (its ovaries consisted of small brownish oocytes, the mass of the ovaries was three times less, and the mass of the entire reproductive system was four times less than in adults in this group). This individual was excluded from the control sample, but studied like other animals, and the results of the study are used in the Discussion.
The study of water content in tissues, the mass of storage organs (liver, fat bodies) and reserve substances (lipids, glycogen) was carried for 21 animals () out according to the methods described earlier (Pasanen & Koskela Citation1974; Bulakhova & Shishikina Citation2022). Hepatosomatic index (HIS) and fat body index (FBSI) were calculated as the ratio of weights of the liver and fat bodies to body weight, expressed in %. The water content (%) was determined in samples (200–500 mg) of liver tissue and gastrocnemius muscles. A fresh tissue sample was weighed, dried for 2 days at 105°C and then weighed again (according to Pasanen & Koskela Citation1974). Determination of glycogen and lipid concentrations was carried out in 20–50 mg weighed samples of liver and muscle tissues. The glycogen contents were analyzed using o-toluidine according to the methods described previously (Tarnoky & Nagy Citation1963; Severin & Solovyeva Citation1989). An Ecoview-UV-3100 spectrophotometer (Shanghai Mapada Instruments Co., Ltd.) was used for determination of optical density. The content of glycogen in samples was calculated by a number of standard glucose solutions. The lipids in liver and in muscles were extracted with diethyl ether: ethanol (1:3) mixture for 48 h and estimated using the method by Canal et al. (Citation1972).
Statistical analysis
The concentrations of glycogen and lipids, the water content in all tissues and the relative mass of the liver in the control and the experimental groups were compared using the Mann–Whitney U-test. Analyses were performed using Statistica 10; significance was judged at p < 0.05. All values are presented as mean ± SEM unless stated otherwise.
Results
Spadefoots in hypoxia
In the first experiment, at an oxygen concentration in the range of 10–3%, the spadefoots spent 24–34 days, at 2–3% - more than 1 month, only one individual died earlier, on the 38th day of the experiment (). At the end of January, the animals were pitted for biochemical analyses ().
Table II. Duration of stay (days) of spadefoots Pelobates vespertinus in conditions of reduced oxygen content.
In the second experiment (determination of the near-lethal oxygen content values), coma in animals occurred at 0.6–1.8% oxygen, i.e. at a concentration 12–21 times lower than the normal atmospheric one (). The coma state was reversible within a few hours: in the preliminary experiment, two animals transferred to a normal oxygen concentration after 20 h of coma revived and then lived in the laboratory for several months. If the animals remained at the achieved concentrations during the day, they did not survive.
Table III. Duration of stay (days) of the spadefoots Pelobates vespertinus in the experiment to determine the near-lethal oxygen concentration.
The content of carbon dioxide during all experiments did not exceed 0.03%.
At a temperature of 15°C, spadefoots actively moved in containers, responded adequately to manipulations with them, and quickly (in a few minutes) completely buried themselves in the soil.
However, when temperatures dropped to 5 and 3°C, the activity of spadefoots significantly decreased, but the behavior did not differ both in normoxia and in hypoxia at 10, 5, and 2–3% oxygen: they did not show signs of anxiety even when the cameras were tilted or the lighting was changed, they were in normal postures (sitting or lying with their limbs tucked under them), were sluggishly active, moved slowly; some animals “dug” moist filter paper at the bottom of the chambers, turned it into a lump, under which they slowly hid. As the temperature increased, the activity increased again.
At concentrations close to lethal (less than 2%), the behavior of the spadefoots changed dramatically and indicated discomfort: they stopped moving, periodically extended one or both hind limbs, remaining in this position from several minutes to half an hour.
Water content in tissues
The water content of the animals did not differ in the liver and muscles in any of the four groups (U-test, p > 0.05). Also, the water content in the tissues did not differ either between the two control or between the control and the experimental animals, despite the fact that they were kept in environments with different humidity (control - in soil with a moisture content of about 35–40%, experimental - in air with humidity of about 100% and condensate on the walls of the vessel) (). This lack of differences made it possible to compare the content of reserve substances in groups of animals without standardization of body weight and conversion to dry matter.
Table IV. Water content (%) in the liver and muscles of the Pelobates vespertinus in the control and experimental groups.
Mass of storage organs
Liver
The mass of the liver was small, the maximum value among all individuals reached only 560 mg, the minimum was 180 mg. The relative mass of the liver in the first control and the second experimental groups was 2.9% (). Although the liver index was lower in the other two groups (2.5–2.6%), there were no significant differences between the four groups (U-test, p > 0.05).
Fat bodies
Not all animals had fat bodies. In the control groups, at the end of January, only 50% of animals had fat bodies weighing 21 ± 6 mg (5–31 mg), and by mid-May, their proportion decreased to 25% (1 individual with a fat body weight of 22 mg). In the immature female, who was in normoxia until the end of January, the mass of fat bodies was many times higher than in all other animals studied – 190 mg.
Among the experimental animals that were in 2–3% oxygen, the proportion of individuals with fat bodies was higher – 67%, and the mass of fat bodies was higher – 37 ± 9 mg (19–58 mg) – than in control animals at the same time. In the second experimental group, none of the animals had fat bodies; however, this may be due to the smallest sample size.
The relative mass of fat bodies in adults was low – FBSI on average was 0.05–0.1% in the control groups and 0.24% in the first experimental group, while in the immature female in the control group at the end of January it was 1.4%.
The content of lipids and glycogen in tissues
The content of lipids in the liver of animals in both control groups did not differ and amounted to only 2.6 mg/g of wet tissue. In the experimental groups, it was not significantly higher, 3.2–3.5 mg/g. The total lipid content in the liver, taking into account the mass of the liver, in all groups was only 0.8–0.9 mg. In the muscles in all groups, the lipid content was higher than in the liver (1.4–2.5 times) and reached an average of 3.7–6.2 mg/g in the control groups and was slightly higher (7.9–8.5 mg/g) in the experimental ones.
The maximum content of lipids in tissues among all the studied animals was in the immature female: 4.8 mg/g of liver and 13.1 mg/g of muscles.
The content of glycogen in the tissues of control animals that spent about 4 months in the laboratory under normoxic conditions was not high (liver – 35.4 ± 5.1 mg/g of wet tissue; muscles – 4.1 ± 1.0 mg/g of wet tissue). By the end of the 7.5-month laboratory wintering, it decreased significant (U-test, p = 0.008) in the control group to 2.1 and 0.2 mg/g, respectively ().
Figure 3. Glycogen content (mg/g of wet tissue) in liver (a) and muscles (b) in four groups of spadefoots Pelobates vespertinus. Circles - individual data points.
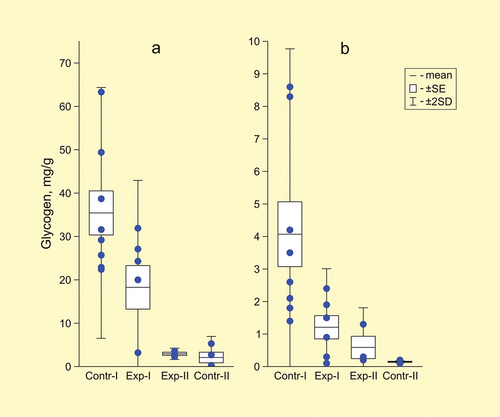
In the first experimental group, the glycogen content in the liver was two times lower than in the control animals at the same time (18.3 ± 5.0 mg/g of wet tissue) and 3.4 times lower in muscles (up to 1.2 ± 0.4 mg/g of wet tissue, U-test, p = 0.02) (). In animals of the second experimental group, it decreased to 3.1 ± 0.3 mg/g in the liver and 0.7 ± 0.3 mg in the muscles (compared to “cont-I” in both tissues U-test, p = 0.02).
The highest glycogen content among the studied animals was in the immature female at the end of January – 81.8 mg/g in the liver and 11.7 mg/g in the muscles.
Discussion
On the attitude of Pallas’ spadefoots to hypoxia
According to the results of this work, the Pallas’ spadefoot can be considered the high tolerant to hypoxia among amphibians burrowing into the ground. Even a long-term (more than a month) almost tenfold oxygen deficiency in the ground air was successfully tolerated by most spadefoots (86%). However, exposure to concentrations of 0.6–1.8% is fatal to them. Thus, the Pallas’ spadefoot can withstand extreme hypoxia for a long time, but cannot tolerate a complete lack of oxygen (anoxia), which is what the most oxygen-deficient amphibian, the Siberian frog, hibernating in water, is capable of (Berman et al. Citation2019). The accuracy of the oximeter used (±0.5%) does not allow one to accurately determine the lower threshold of oxygen concentration at which spadefoots can survive for a long time, but the data obtained reliably indicate that it lies below 2%.
Survival in such an extreme (both in terms of concentration and duration) oxygen deficiency in the air is still unique for amphibians. In much milder hypoxia, at oxygen concentrations of about half of normal (10%), the hylid frog Cyclorana alboguttata can live for a long time (Rossi et al. Citation2020). Some species can endure more severe hypoxia only for a short time. For example, toads Bufo marinus survive in the laboratory at oxygen concentrations of 7–10% for a day, and at 5% for 1 hour (Wood & Malvin Citation1991; Gamperl et al. Citation1999); B. paracnemis at 5% oxygen – for a few minutes (Kruhøffer et al. Citation1987). Under anoxic conditions, Bufo valliceps and B. woodhousei survived an average of 6–8 min (Guttman Citation1974), salamander Proteus anguinus – 12 h, and newt Calotriton asper – only 1.5 h (Issartel et al. Citation2009). However, most of the listed species are inhabitants of warm regions, and they do not encounter such low temperatures during hibernation/aestivation as the Pallas’ spadefoot does, and the above oxygen concentrations and duration of stay in them were obtained in laboratory experiments at temperatures from 12 to 32°C. It is likely that with a decrease in temperature, their resistance to hypoxia will increase, due to a suppression of metabolism and a decrease in oxygen demand. For example, the leopard frog Rana pipiens survives under anoxic conditions for about 3 h at 25°С, but 30 h at 5°С (Hermes-Lima & Storey Citation1996; Knickerbocker & Lutz Citation2001).
In different areas, the Pallas’ spadefoot winters deep in the ground, in some areas – up to 1.5–2 m (Iskakova Citation1959; Garanin Citation1983; Debelo & Chibilev Citation2013). For many species of burrowing anurans living in hot or xeric conditions, such a large depth of aestivation is due to the avoidance of high temperatures and the response to soil dryness (Seymour Citation1973b; Carvalho et al. Citation2010; Szekely et al. Citation2018, etc.). For the Pallas’ spadefoot, burying is a strategy due to intolerance to negative winter temperatures. This species is among the most cold-sensitive among boreal amphibians: in the experiments, only one individual out of five withstood exposure of −1°C for 3 days (Berman et al. Citation2019a, Citation2020).
Most aestivating anurans live in places with sandy soils or in drying mud, and these substrates may impose different limitations for these species regarding oxygen. Sandy microhabitats are not expected to be oxygen-limited; conversely, drying mud microhabitats as well as cocoons of aestivating anurans are likely to be less permeable to oxygen, but have been little studied in this context (Seymour Citation1973b; Carvalho et al. Citation2010; Rossi et al. Citation2020).
The Pallas’ spadefoot also prefers sandy, sandy clay or broken soil, but is common in areas with seasonally frozen soils in winter - the 0°С isotherm in most of the species range is at a depth of 80 cm, and in the east of the range it is even deeper, at a depth of 120–160 cm (Berman et al. Citation2020; Bulakhova et al. Citation2020). Such deep freezing of soils not only forces a spadefoots to burrow deep (below the freezing level), but can also impede air diffusion in soils and cause a change in the composition of soil air. Flooding of sites, in which water penetrating the soil displaces air ahead of the wetting front (Lavelle & Spain Citation2001), probably also affects the gas composition in soils. This impact can be significant and prolonged (due to snow melting in spring or thaws in early winter). However, the places of wintering of the spadefoot in relation to the supply of oxygen have not been studied at all, as well as for many aestivating species.
The role of lipids in the metabolism of spadefoots
In burrowing amphibians, lipids are considered to be the main metabolic fuel during aestivation/hibernation (see Feder & Burggren Citation1992). Lipids are stored in various tissues (liver, muscles, subcutaneous deposits, etc.), but their main depots in burrowing amphibians are abdominal fat bodies, which reach a significant size: in burrowing toads Anaxyrus fowleri – on average 3% (Bush Citation1963), in Scaphiopus – 3–5% and in Cyclorana platycephala – 10–24% (Seymour Citation1973a; van Beurden Citation1980). The lipid reserve in fat bodies of this size is sufficient to meet all the energy requirements of 7–10 months or even two years of aestivation, and in C. platycephala – of up to 5–6 years (Bush Citation1963; McClanachan Citation1967; Seymour Citation1973a).
In the spadefoots in our study, after 4 months of wintering in normoxia (at the end of January), only half of the animals had fat bodies, the mass of which was small (on average, about 0.1% of the total body weight); by the end of wintering (mid-May), the proportion of animals with fat bodies decreased to a quarter. This successive reduction in the proportion of animals with fat bodies confirms that, at normal oxygen levels, fat bodies play a role in aerobic metabolism (Pinder et al. Citation1992) and are consumed even at low temperatures (about 3°C). A significant mass of fat bodies in the middle of wintering in the immature female (FBSI 1.4%), as well as a higher content of lipids and glycogen in other tissues (see below) compared to adults, indicates differences in patterns of storage and consumption of reserve substances in age and/or sex groups, which is typical for many amphibian species (Pasanen & Koskela Citation1974; Bulakhova & Shishikina Citation2022). The average FBSI values of the toadlets of P. vespertinus in different populations during the exit from ponds in the second half of summer ranged from 2 to 10% (Yermokhin et al. Citation2014).
In the liver of spadefoots, the lipid content in the middle of wintering was low (2.6 mg/g of tissue) and did not differ from that, for example, in the frost-resistant moor frog Rana arvalis (Bulakhova & Shishikina Citation2022), but it was significantly lower than in the non-cold-resistant R. temporaraia (Passanen & Koskela Citation1974) or Rana esculenta (Iela et al. Citation1979). It is likely that the liver of the spadefoot, like that of other amphibian species (Fitzpatrick Citation1976), is not a lipid depot, but only carries out synthesis and transport of substances from one organ to another (for example, from fat bodies to developing gonads in species in which gametogenesis continues in winter).
In muscles, the content of lipids in all groups of spadefoots was higher than in the liver. It is known that in the spadefoot Scaphiopus and the hylid frog Cyclorana platycephala wintering in the soil, lipids also accumulate under the skin (McClanachan Citation1967; Seymour Citation1973a; van Beurden Citation1980). We did not investigate the presence of subcutaneous fat deposits in the Pallas’ spadefoot (but they were not visible during standard autopsy procedures and muscle tissue sampling), and other authors have not studied the content of lipids in muscles in burrowing species. However, for species of Eurasian frogs (Rana temporaria, R. dybowski, R. amurensis, R. arvalis) wintering in water or on land, the lipid content in the liver during wintering was always higher than in the muscles (our data). It is likely that the muscles of Pallas’ spadefoots may have some significance as a lipid depot, which requires further research.
The small size of fat bodies and the high proportion of animals without them already in the middle of wintering, the low content of lipids in the liver and muscles indicate either a low metabolic rate even at a normal oxygen content, or the use of other energy resources to ensure successful wintering. Obtaining energy from lipids is possible only in the presence of oxygen (Pinder et al. Citation1992), but stops with hypoxia. Pallas’ spadefoots, which had been in normoxia for 4 months, had almost half the mass of fat bodies than those who spent part of this time (38–70 days) at a reduced oxygen content. The proportion of animals that completely catabolized fat bodies in these two groups also differed: 50% in the control and only 33% in the experiment. Obviously, hypoxia for almost half the duration of the experiment affected the involvement of lipids in metabolism – low oxygen levels did not allow lipids to be oxidized, and the animals used non-lipid metabolism.
The role of glycogen in the Pallas’ spadefoot metabolism
Respiratory coefficients in Scaphiopus spadefoots indicate that during wintering, carbohydrates and proteins are used along with lipids (McClanachan Citation1967). In a normoxic Pallas’ spadefoot, along with a reduction in the size of fat bodies, the content of glycogen in tissues decreases: in the second half of wintering (from late January to mid-May) for 3.5 months, it decreased by an average of 33 mg/g in the liver and almost 4 mg/g in the muscles. Although one cannot rule out a higher rate of resource consumption in laboratory conditions compared to the natural situation, as shown for other amphibian species (Koskela & Pasanen Citation1975), glycogen consumption in the Pallas’ spadefoot is, nevertheless, low.
The content of glycogen in the liver of the Pallas’ spadefoot, which was in normoxia in the state of torpor in the middle of wintering (35.4 mg/g), was significantly lower than that of amphibians wintering in water at the same temperatures (3–4°C). Indeed in adult females of R. temporaria in the middle of wintering, the glycogen content in the liver averaged about 90 mg/g of wet tissue (Kato Citation1910; Smith Citation1950; Pasanen & Koskela Citation1974), in R. esculenta, 130 mg/g (Kato Citation1910), in R. chensinensis and R. dybowski, about 70 mg/g (Hong et al. Citation1968; our unpublished data). The content of glycogen in the muscles of the spadefoots was extremely low (about 4 mg/g of tissue) and several times lower than in the above frog species (Pasanen & Koskela Citation1974; our unpublished data). With a low relative mass of the liver in the spadefoots compared to the indicated species of frogs (less than 3% versus 3–5%) and the average mass of muscle tissue in an individual of less than 2.5 g, the total glycogen reserve in both tissues in the middle of wintering in normoxia turned out to be several times less than in other studied amphibian species (Kato Citation1910; Hong et al. Citation1968; Pasanen & Koskela Citation1974; our data).
Thus, the Pallas’ spadefoot (at least adults) may be the species with the lowest pre-wintering tissue glycogen content compared to other amphibians. Probably, the lack of cold and frost resistance in this species is associated with a low glycogen reserve (Berman et al. Citation2019a), since the survival and minimal tolerable temperatures during freezing in amphibians are determined by the synthesis of cryoprotectants, the amount of which depends on the amount of glycogen in the liver (Costanzo & Lee Citation1993).
Pallas’ spadefoots that spent 2 months in low oxygen conditions used up twice as much glycogen as controls, but retained large fat bodies. It is obvious that this is caused by the conditions of the experiment – oxygen deficiency. Although we did not determine the level of glucose and lactate in the tissues, it is difficult to suggest another variant as a shift of metabolism from aerobic lipid or carbohydrate during normoxia to anaerobic metabolism due to glycogen consumption during hypoxia. Similarly, the common frog Rana temporaria and Cyclorana platycephala efficiently use both lipids and carbohydrates in normoxia, and there is only modest use of glycogen reserves (van Beurden Citation1980; Donohoe et al. Citation1998). With progressive hypoxia, dependence on carbohydrate metabolism increases and the glycogen concentrations in the tissues of hypoxic frogs are more rapidly depleted than in their normoxic counterparts (Donohoe & Boutilier Citation1998).
Although the rate of glycogen consumption in the experimental groups of P. vespertinus was higher than in the control groups, it was significantly lower than that of the species intolerant to hypoxia. For example, in moderately resistant R. temporaria, the liver glycogen store becomes depleted within the first week of hypoxic exposure when the oxygen content in the water is about 40% of normal content (Boutilier Citation2001), while in hypoxia-intolerant Bufo cognatus, the concentration of hepatic glycogen halves after just 40 minutes in anoxia (Armentrout & Rose Citation1971). At the same time, the rate of glycogen consumption in the spadefoot under oxygen deficiency is higher than in the Siberian wood frog, the most adapted to anoxia anuran amphibian. Its glycogen content is halved only during almost two months of complete anoxia at the same temperatures (our unpublished data).
Metabolic depression is a common response to adverse environmental conditions in animals (including amphibians) and underlies hibernation, torpor, diapause, anaerobiosis and anhydrobiosis, and aestivation (Hochachka & Guppy Citation1987; Pinder et al. Citation1992). The long-term tolerance of Pallas’ spadefoot to hypoxia is obviously the result of the activation of glycolysis in combination with the suppression of metabolism. Lower temperature affects animal activity and metabolic rate, and physiological and metabolic plasticity provides energy savings; their cumulative effect is aimed at maintaining metabolism at a minimum level, so that resource reserves do not become limiting for survival (Boutilier et al. Citation1997). Based on the small size of energy “depots” (liver and fat bodies) and the low content of stored resources (lipids and glycogen), it can be assumed that the role of metabolic depression for survival during long wintering in P. vespertinus must be very big.
Conclusion
According to the results of this work, the Pallas’ spadefoot can now be considered as the high tolerant to hypoxia amphibian species, wintering in soils at positive temperatures. It is able to stay in hypoxic conditions (the oxygen content is almost 10 times lower than normal) for a long time, remaining in an active, albeit somewhat inhibited, state. In the class of amphibians, only one species has been known so far that endures extreme hypoxia (oxygen is less than 1.5% of the norm) for a long time (for months) while wintering in water (Siberian wood frog, Rana amurensis) (Berman et al. Citation2019). The level of minimum vital oxygen content for the P. vespertinus is quite high compared to R. amurensis, but significantly lower than that of other Eurasian and American frog species wintering in water: R. muscosa (the oxygen content threshold for adults is about 20% of the norm) (Bradford Citation1983), R. temporaria and R. dybowski (above 25%), R. macrocnemis (above 30%) (Berman & Bulakhova Citation2019; Bulakhova et al. Citation2020a), R. catesbeiana, R. pipiens (above 40%) (Tattersall & Ultsch Citation2008). The ability of amphibian species hibernating/aestivating in the soil to tolerate hypoxia has been little studied, since it is believed that at the depth of their burrowing, the oxygen content is close to normal, and a long-term survival in the soil at a considerable depth is ensured by normoxic metabolic depression (Seymour Citation1973a; Flanigan et al. Citation1990; Pinder et al. Citation1992; Carvalho et al. Citation2010; Moreira et al. Citation2020). Only Cyclorana alboguttata has been shown to be able to survive for a long time at half the normal oxygen content using anaerobic metabolic depression (Rossi et al. Citation2020).
Pallas’ spadefoot spends more than half a year in wintering, having small size of storage organs with low content of fats and glycogen in them, and low consumption rate of reserve substances even at normal oxygen content in the environment. Based on this, it can be assumed that the role of a decrease in the metabolic rate for survival during a long wintering in the spadefoot must be very big. It is likely that low temperatures in wintering areas play a leading role in initiating metabolic depression. The long-term tolerance of hypoxia in the Pallas’s spadefoot, against the background of a stoppage of lipid consumption and an increase in glycogen consumption, obviously indicates the activation of glycolysis. It is likely that the combined effect of reducing the metabolic rate and activating glycolysis makes the Pallas’s spadefoot one of the most hypoxia-tolerant amphibian species.
Acknowledgements
We thank V.G. Tabachishin for providing spadefoots for research, S.M. Golubtsov and E.A. Andriyanova for transporting animals to Magadan.
Disclosure statement
No potential conflict of interest was reported by the author(s).
Additional information
Funding
References
- Armentrout D, Rose FL. 1971. Some physiological responses to anoxia in the Great Plains toad, Bufo cognatus. Comparative Biochemistry and Physiology 39A:447–455. DOI:10.1016/0300-9629(71)90308-2.
- Belik VP. 2010. On amphibian fauna and ecology in the steppe part of the Don basin. Sovremennaya Gerpetologiya 10(3/4):89–100.
- Berman DI, Alfimov AV, Bulakhova NA. 2020. Maps to play, or why doesn’t Pallas’ spadefoot toad go East. Priroda 11:22–36. DOI: 10.7868/S0032874X20110034.
- Berman DI, Bulakhova NA. 2019. How winterkill suffocations stop the common frog spreading from Europe to Asia. Priroda 7:12–26. DOI: 10.7868/S0032874X19070020.
- Berman DI, Bulakhova NA, Balan IV. 2017. The most Siberian frog. Priroda 8:3–14.
- Berman DI, Bulakhova NA, Meshcheryakova EN. 2019. The Siberian wood frog survives for months underwater without oxygen. Scientific Reports 9:13594–13600. DOI: 10.1038/s41598-018-31974-6.
- Berman DI, Bulakhova NA, Mesheryakova EN, Yermokhin MV, Tabachishin VG. 2019a. Cold-hardiness of the common spadefoot Pelobates fuscus (Pelobatidae, Anura, Amphibia). Cryo Letters 40(5):284–290.
- Berman DI, Leirikh AN, Mikhailova EI. 1984. Wintering of the Siberian salamander Hynobius keyserlingi in the Upper Kolyma. Journal of Evolutionary Biochemistry and Physiology 20(3):323–327.
- Bickler PE, Buck LT. 2007. Hypoxia tolerance in reptiles, amphibians, and fishes: Life with variable oxygen availability. Annual Review of Physiology 69:145–170. DOI: 10.1146/annurev.physiol.69.031905.162529.
- Bleibtreu M. 1911. Weitere untersuchungen uber das verhalten des glykogens im eierstock der Rana fusca. Pflügers Archiv European Journal of Physiology 141(4–7):328–342. DOI:10.1007/BF01689701.
- Boutilier RG. 2001. Mechanisms of metabolic defense against hypoxia in hibernating frogs. Respiration Physiology 128:365–377. DOI: 10.1016/S0034-5687(01)00312-7.
- Boutilier RG, Donohoe PH, Tattersall GJ, West TG. 1997. Hypometabolic homeostasis in overwintering aquatic amphibians. Journal of Experimental Biology 200:387–400. DOI: 10.1242/jeb.200.2.387.
- Bradford DF. 1983. Winterkill, oxygen relations, and energy metabolism of a submerged dormant amphibian, Rana muscosa. Ecology 64(5):1171–1183. DOI:10.2307/1937827/.
- Bulakhova N, Alfimov A, Berman I. 2020. The eastern boundary of the geographic range of the Pallas’ spadefoot Pelobates vespertinus (Anura, Amphibia) is limited by overwintering temperatures. Herpetozoa 33:171–175. DOI: 10.3897/herpetozoa.33.e58050.
- Bulakhova NA, Mazanaeva LF, Meshcheryakova EN, Berman DI. 2020a. Resistance of the Iranian long-legged wood frog (Rana macrocnemis Boulenger, 1885) (Amphibia, Anura) to negative temperatures on land and to hypoxia in water during overwintering. Herpetology Notes 13:1079–1086.
- Bulakhova N, Shishikina K. 2022. Pre-hibernation energy reserves and their consumption during freezing in the moor frog Rana arvalis in Siberia. European Zoological Journal 89(1):556–567. DOI:10.1080/24750263.2022.2060357.
- Bush FM. 1963. Effects of light and temperature on the gross composition of the toad, Bufo fowleri. Journal of Experimental Zoology 153(1):1–13. DOI:10.1002/jez.1401530102.
- Canal J, Delattre J, Girard ML. 1972. Acquisitions nouvelles dans le dosage des lipides totaux du serum: Description d’une methode nephelemetrique. Part 1. Technique manuelle. Annales de Biologie Clinique 30:325–332.
- Carvalho JE, Navas CA, Pereira IC. 2010. Energy and water in aestivating amphibians. Aestivation 141–169. DOI: 10.1007/978-3-642-02421-4_7.
- Costanzo JP, Lee R. 1993. Cryoprotectant production capacity of the freeze-tolerant wood frog, Rana sylvatica. CANadian Journal of ZOOLogy 71:71–75. DOI:10.1139/z93-011.
- Costanzo JP, Reynolds AM, Do Amaral MCF, Rosendale AJ, Lee RE. 2015. Cryoprotectants and extreme freeze tolerance in a subarctic population of the wood frog. PLoS One 10(2):e0117234. DOI:10.1371/journal.pone.0117234.
- Debelo PV, Chibilev AA. 2013. Amphibians and reptiles of the Ural-Caspian region. Series: Natural Diversity of the Ural-Caspian Region. Ekaterinburg: RIO UB RAS.
- Donohoe PH, Boutilier RG. 1998. The protective effects of metabolic rate depression in hypoxic cold submerged frogs. Respiration Physiology 111(3):325–336. DOI: 10.1016/S0034-5687(97)00125-4.
- Donohoe PH, West TG, Boutilier RG. 1998. Respiratory, metabolic and acid–base correlates of aerobic metabolic rate reduction in overwintering frogs. American Journal of Physiology-Regulatory, Integrative and Comparative Physiology 274:704–710. DOI: 10.1152/ajpregu.1998.274.3.R704.
- Feder ME, Burggren WW. 1992. Environmental physiology of the amphibians. Chicago: University of Chicago Press.
- Fitzpatrick LC. 1976. Life history patterns of storage and utilization of lipids for energy in amphibians. American Zoologist 16(4):725–732. DOI:10.1093/icb/16.4.725.
- Flanigan J, Withers P, Storey K, Guppy M. 1990. Changes in enzyme binding and activity during aestivation in the frog Neobatrachus pelobatoides. Comparative Biochemistry and Physiology Part B: Comparative Biochemistry 96(1):67–71.
- Gamperl AK, Milsom WK, Farrell AP, Wang T. 1999. Cardiorespiratory responses of the toad (Bufo marinus) to hypoxia at two different temperatures. Journal of Experimental Biology 202:3647–3658. DOI: 10.1242/jeb.202.24.3647.
- Garanin VI. 1983. Amphibians and reptiles of the Volga-Kama region. Moscow: Nauka Press.
- Glass ML, Fernandes MS, Soncini R, Glass H, Wasser JS. 1998. Effects of dry season dormancy on oxygen uptake, heart rate, and blood pressures in the toad, Bufo paracnemis. Journal of Experimental Zoology 279(4):330–336. DOI:10.1002/(SICI)1097-010X(19971101)279:4<330::AID-JEZ2>3.0.CO;2-P.
- Guttman S. 1974. Anoxia tolerance in two species of toads, Bufo valliceps and Bufo woodhousei. Comparative Biochemistry and Physiology Part A: Physiology 47(3):867–870. DOI:10.1016/0300-9629(74)90461-7.
- Hermes-Lima M, Storey KB. 1996. Relationship between anoxia exposure and antioxidant status in the frog Rana pipiens. American Journal of Physiology-Regulatory, Integrative and Comparative Physiology 271:991–996. DOI: 10.1152/ajpregu.1996.271.4.R918.
- Hochachka PW, Guppy M. 1987. Metabolic arrest and the control of biological time. Cambridge: Harvard Univ. Press.
- Hong SK, Park CS, Park YS, Kim IK. 1968. Seasonal changes of antidiuretic hormone action on sodium transport across frog skin. American Journal of Phvsiology 215:439–443. DOI: 10.1152/ajplegacy.1968.215.2.439.
- Iela L, Milone M, Filomena M, Rakesh C, Rastogi K, Chieffi G. 1979. Role of lipids in the physiology of the testis of Rana esculenta: Annual changes in the lipid and protein content of the liver, fat body, testis and plasma. Bollettino di Zoologia 46(1–2):11–16. DOI:10.1080/11250007909440272.
- Irwin JT, Lee JRE. 2003. Geographic variation in energy storage and physiological responses to freezing in the gray treefrogs Hyla versicolor and H. chrysoscelis. Journal of Experimental Biology 206(16):2859–2867. DOI:10.1242/jeb.00500.
- Iskakova K. 1959. Amphibians of Kazakhstan. Alma-Ata: Publishing Houses of the Academy of Sciences of the Kazakh SSR.
- Issartel J, Hervant F, de Fraipont M, Clobert J, Voituron Y. 2009. High anoxia tolerance in the subterranean salamander Proteus anguinus without oxidative stress nor activation of antioxidant defenses during reoxygenation. Journal of Comparative Physiology B 179:543–551. DOI: 10.1007/s00360-008-0338-9.
- Kato K. 1910. Uber das verhalten des glykogenes im eierstocke der frosche zu den verschiedenen jahreszeiten. Pflügers Archiv European Journal of Physiology 132:545–579. DOI: 10.1007/BF01683638.
- Knickerbocker DL, Lutz PL. 2001. Slow ATP loss and the defense of ion homeostasis in the anoxic frog brain. Journal of Experimental Biology 204:3547–3551. DOI: 10.1242/jeb.204.20.3547.
- Koskela P, Pasanen S. 1975. Effect of thermal acclimation on seasonal liver and muscle glycogen content in the common frog, Rana temporaria L. Comparative Biochemistry and Physiology Part A: Physiology 50(4):723–727. DOI:10.1016/0300-9629(75)90135-8.
- Kruhøffer M, Glass ML, Abe AS, Johansen K. 1987. Control of breathing in an amphibian, Bufo paracnemius: Effects of temperature and hypoxia. Respiration Physiology 69:267–275. DOI: 10.1016/0034-5687(87)90033-8.
- Lavelle P, Spain AV. 2001. Soil ecology. New York-Boston-Dordrecht-London- Moscow: Kluwer Academic Publishers.
- McAneney J, Gheshmy A, Uthayalingam S, Reid SG. 2006. Chronic hypoxia modulates NMDA-mediated regulation of the hypoxic ventilatory response in an amphibian, Bufo marinus. Respiratory Physiology & Neurobiology 153(1): 23–38. DOI:10.1016/j.resp.2005.09.001.
- McClanachan L. 1967. Adaptations of the spadefoot toad, Scaphiopus couchi, to desert environments. Comparative Biochemistry and Physiology 20:73–99. DOI:10.1016/0010-406X(67)90726-8.
- Mizell S. 1965. Seasonal changes in energy reserves in the common frog, Rana pipiens. Journal of Cellular and Comparative Physiology 66(2):251–258. DOI:10.1002/jcp.1030660212.
- Moreira DC, Carvajalino-Fernández JM, Silva WP, Kuzniewski F, Navas CA, de Carvalho JE, and Hermes-Lima M. 2020. Preparation for oxidative stress in Proceratophrys cristiceps (Anura, Odontophrynidae) naturally estivating in the Brazilian Caatinga. The Science of the Total Environment 723:137957. DOI:10.1016/j.scitotenv.2020.137957.
- Morton ML. 1981. Seasonal changes in total body lipid and liver weight in the Yosemite toad. Copeia 1981(1):234–238. DOI:10.2307/1444067.
- Pasanen S, Koskela P. 1974. Seasonal and age variation in the metabolism of the common frog, Rana temporaria L, in northern Finland. Comparative Biochemistry and Physiology A 47(2):635–654. DOI:10.1016/0300-9629(74)90027-9.
- Pinder AW, Storey KB, Ultsch GR. 1992. Estivation and hibernation. In: Feder ME, Burggren WW, editors. Environmental Physiology of the Amphibians. Chicago: Illinois, Univ. pp. 250–274.
- Pörtner HO, MacLatchy LM, Toews DP. 1991. Metabolic responses of the toad Bufo marinus to environmental hypoxia: An analysis of the critical Po2. Physiological Zoology 64(2):836–849. DOI:10.1086/physzool.64.3.30158210.
- Rossi GS, Cramp RL, Wright PA, Franklin CE. 2020. Frogs seek hypoxic microhabitats that accentuate metabolic depression during dormancy. Journal of Experimental Biology 223:jeb218743. DOI: 10.1242/jeb.218743.
- Schlaghecke R, Blom V. 1978. Seasonal variations in fat body metabolism of the green frog Rana esculenta (L.). Experientia 34:456–457. DOI: 10.1007/BF01915320.
- Severin SE, Solovyeva GA. 1989. Praktikum po biohimii. Moscow: MSU.
- Seymour RS. 1973a. Energy metabolism of dormant spadefoot toads (Scaphiopus). Copeia 1973(3):435–445. DOI:10.2307/1443107.
- Seymour RS. 1973b. Gas exchange in spadefoot toads beneath the ground. Copeia 1973(3):452–460. DOI:10.2307/1443109.
- Shekhovtsov SV, Bulakhova NA, Tsentalovich YP, Zelentsova EA, Yanshole LV, Meshcheryakova EN, Berman DI. 2020. Metabolic response of the Siberian wood frog Rana amurensis to extreme hypoxia. Scientific Reports 10:14604. DOI: 10.1038/s41598-020-71616-4.
- Smagin AV 2005. Gas phase of soil Moscow: MSU.
- Smith CL. 1950. Seasonal changes in blood sugar, fat bodies, liver glycogen and gonads in the common frog (Rana temporaria). Journal of Experimental Biology 26(4):412–429. DOI:10.1242/jeb.26.4.412.
- Storey KB, Storey JM. 1984. Biochemical adaptation for freezing tolerance in the wood frog, Rana sylvatica. Comparative Biochemistry and Physiology B 155:29–36. DOI: 10.1007/BF00688788.
- Székely D, Cogălniceanu D, Székely P, Denoël M. 2018. Dryness affects burrowing depth in a semi-fossorial amphibian. Journal of Arid Environments 155:79–81. DOI:10.1016/j.jaridenv.2018.02.003.
- Tarnoky K, Nagy S. 1963. Spectrophotometric determination of glycogen with o-toluidine. Clinica Chimica Acta 8:627–628. DOI: 10.1016/0009-8981(63)90116-5.
- Tattersall GJ, Ultsch GR. 2008. Physiological ecology of aquatic overwintering in ranid frogs. Biological Reviews 83:119–140. DOI: 10.1111/j.1469-185X.2008.00035.x.
- Ultsch GR, Anderson JF 1986. The respiratory microenvironment within the burrows of gopher tortoises (Gopherus polyphemus). Copeia 1986(3):787–795.
- van Beurden EK. 1980. Energy metabolism of dormant Australian water-holding frogs (Cyclorana platycephalus). Copeia 1980:787–799. DOI: 10.2307/1444458.
- Wang GL, Jiang BH, Rue EA, Semenza GL. 1994. Hypoxia-inducible factor 1 is a basic-helix-loop-helix-PAS heterodimer regulated by cellular O2 tension. Proceeding of the National Academy of Sciences USA 92(12):5510–5514. DOI:10.1073/pnas.92.12.55.
- Wells KD. 2007. The ecology and behavior of amphibians. Chicago: University of Chicago Press.
- Withers P. 1978. Models of diffusion-mediated gas exchange in animal burrows. American Naturalist 112(988):1101–1112. DOI:10.1086/283349.
- Wood S, Malvin G. 1991. Physiological significance of behavioral hypothermia in hypoxic toads (Bufo marinus). Journal of Experimental Biology 159(1):203–215. DOI:10.1242/jeb.159.1.203.
- Yermokhin MV, Tabachishin VG, Ivanov GA. 2014. Comparative analysis of body condition indexes efficiency of Pelobates fuscus toadlets. Sovremennaya Gerpetologiya 14(3–4):92–102.
- Yermokhin MV, Tabachishin VG, Ivanov GA, Bogoslovsky DS. 2013. Features of the location of Pelobates fuscus in the soil profile in the Medveditsa River valley at a beginning hibernation period. Sovremennaya Gerpetologiya 13(1–2):22–26.