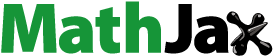
Abstract
This study investigated whether MgO nanoparticles (NPs) produced via the sol–gel process can simultaneously remove the pharmaceutical diclofenac sodium (DCF) and cadmium ions (Cd2+) from water. The UV spectroscopy, Fourier transform infrared spectroscopy (FTIR), X-ray diffraction (XRD), scanning electron microscopy (SEM), transmission electron microscopy (TEM), and energy-dispersive X-ray spectroscopy (EDX) were used to confirm the composition, morphology and particle size of the MgO NPs. The adsorption performance of MgO NPs was compared to that of bentonite-nano zero valent iron (B-nZVI) and activated carbon (AC). The impact of contact time, adsorbate concentration, pH, and temperature on the removal efficacy of DCF and Cd2+ via MgO NPs in aqueous solutions were investigated, and the removal data was best described by the kinetic model of pseudo-second-order and the Langmuir isotherm model (R2 > 0.996). The maximum removal capacity of MgO for DCF and Cd2+ at pH 7 was 66.2 mg.g−1 (%R = 90) and 50.8 mg.g−1 (%R = 80), respectively. The average adsorption enthalpy for DCF and Cd2+ (ΔH° 50 kJ. mol−1) demonstrates the occurrence of physical adsorption process, while the negative value of ΔG° reveals the spontaneous nature of DCF and Cd2+ adsorption on the surface of MgO NPs. The DCF and Cd2+ adsorbed MgO surfaces were reused against various gram-positive bacteria. Finally, it was shown that MgO and sand column were more effective for removal of persistent substances and heavy metal residues than a column packed by a mixture of B-nZVI and sand.
1. Introduction
Recent studies have shown that typical approaches for purifying water from recalcitrant organic contaminants were inefficient. The prevailing environmental pollutants detected in water sources of late encompass pharmaceutical compounds, microorganisms, as well as heavy metal ions Ni2+, Cu2+, Pb2+, and Cd2+. These substances could potentially pose a carcinogenic threat to human health (Sulaiman et al., Citation2016; Sulaimana et al., Citation2014; Sulaiman & Shahwan, Citation2017). Also, the contaminated water by microorganism is commonly caused the transmission of waterborne disease (Al-Jabari, Imtiaz, Sulaiman, Alawi, & Shilo, Citation2018; Al-Jabari et al., Citation2019; Sulaiman et al., Citation2015).
The proliferation of water pollution coincides with the rise in population and urban industrialization, along with the exacerbation of water resource scarcity. Which pose significant threats to both human well-being and the environment (Guangyu & Thiruvenkatachari, Citation2003; Shannon et al., Citation2008; Venieri et al., Citation2014).
Consequently, the need for an effective approach to treating polluted water made necessary the development of alternatives to current conventional methods. Numerous techniques for water remediation have already been employed. Among them, adsorption appears as a remarkably efficient, adaptable, and cost-effective method utilized in water purification. The integration of nanotechnology using adsorbent nanomaterials in water remediation significantly increased the adsorption capacity towards water treatment. This increase can be ascribed to high large surface areas of nanomaterials, their low-cost operation, and the plenty of active sites available. In addition to this, various techniques for water remediation have been employed (Chen, He, Wang, & Wei, Citation2014; Choe et al., Citation2015; Fominykh et al., Citation2014; Huang, Lu, & Li, Citation2012; Jin et al., Citation2016; Kang, Park, Choi, Lee, & Yi, Citation2004; Liang, Zhang, Wang, Zhang, & Liu, Citation2015; Mbamba, Batstone, Flores-Alsina, & Tait, Citation2015; Moussavi & Mahmoudi, Citation2009; Wu et al., Citation2016; Zhang, Gao, Yao, Xue, & Inyang, Citation2012).
Magnesium oxide (MgO) nanoparticles, a type of inorganic metal oxide, offer an affordable, abundant, non-toxic, and environmentally friendly solution for effectively eliminating heavy metal ions (Kang et al., Citation2004), organic dyes (Moussavi & Mahmoudi, Citation2009), fluorides (Jin et al., Citation2016), and phosphates (Zhang et al., Citation2012) from aqueous solutions. Furthermore, these nanoparticles exhibit significant antibacterial properties, making them a potent antibacterial agent (Stoimenov, Klinger, Marchin, & Klabunde, Citation2002). In comparison to other techniques like activated sludge application, ion exchange, and photocatalysis, the utilization of nanoparticle materials exhibits a considerable potential for achieving these tasks. Primary research has mainly been focused on employing MgO nanoparticles for antibacterial attributes and adsorption capabilities. Numerous studies have illustrated the inhibitory impacts of MgO nanoparticles on a range of bacterial types, including both gram-positive and gram-negative strains (Jin & He, Citation2011; Krishnamoorthy, Manivannan, Kim, Jeyasubramanian, & Premanathan, Citation2012; Sawai et al., Citation2000; Stoimenov et al., Citation2002; Wetteland, Nguyen, & Liu, Citation2016). The potential of MgO nanoparticles as an antibacterial agent was investigated (Tang & Lv, Citation2014). It was suggested in previous research that the primary mechanism behind the antibacterial effects of MgO NPs might relate to the generation of reactive oxygen species (ROS) (Stoimenov et al., Citation2002). However, there is a lack of comprehensive research evaluating the combined benefit of MgO NPs, covering their antibacterial strength and ability to eliminate heavy metals and pharmaceutical compounds from water (Cai et al., Citation2017; Sawai et al., Citation2000).
Moussavi and Mahmoudi (Citation2009) investigated the removal of azo and anthraquinone reactive dyes from industrial wastewaters using magnesium oxide (MgO) nanoparticles. The study aimed to evaluate the adsorption performance of MgO nanoparticles compared to bentonite-nano zero-valent iron (B-nZVI) and activated carbon (AC) for the removal of reactive dyes. The impact of contact time, adsorbate concentration, pH, and temperature were studied.
Porous MgO nanoplates are highly effective in removing fluoride from water, with adsorption capacities exceeding 185.5 mg/g at neutral pH. This efficiency stems from the strong electrostatic attraction between the high isoelectric point of MgO and fluoride ions, along with the abundant availability of magnesium in nature. Unlike commercial MgO, which has limited adsorption capacity, porous MgO nanoplates outperform due to their distinctive porous structure. The adsorption mechanism involves hydroxyl exchange and the formation of surface carbonates from the reaction of MgO with CO2 in the air, which can also exchange with fluoride. Additionally, the dissolution ratio of Mg2+ is below 0.2%, ensuring that it is a safe and effective adsorbent for fluoride removal (Jin et al., Citation2016).
Sawai et al. (Citation2000) offered valuable insights into the antibacterial properties of MgO powder, showcasing its potential as a ceramic antibacterial agent. The research revealed that MgO powder possesses significant antibacterial activity against a range of bacteria, primarily due to the generation of ROS and the release of Mg2+ ions. Additionally, the study emphasized the critical role of particle size in influencing the antibacterial effectiveness of MgO powder.
The antibacterial function of MgO nanoparticles can be attributed to the lipid peroxidation caused by ROS generated through oxygen vacancies. These oxygen vacancies produce ROS, which can oxidize the lipids in bacterial cell membranes, leading to lipid peroxidation. This process causes damage to the lipids in the bacterial cell membrane, compromising its integrity and resulting in cell death. Therefore, the unique properties of MgO nanoparticles, especially their ability to generate oxygen vacancies, are essential for their effective antibacterial function (Krishnamoorthy et al., Citation2012).
Shahid et al. (Citation2022) found that MgO nanoparticles exhibited significant anti-inflammatory activity by inhibiting protein denaturation, with an IC50 value lower than that of the standard, indicating superior effectiveness. Additionally, the MgO nanoparticles demonstrated notable free radical scavenging activity, with IC50 values suggesting they are more effective at scavenging free radicals compared to the standard (ascorbic acid). Characterization of the MgO nanoparticles was performed using EDX, XRD, and SEM techniques, revealing a crystallite size of 19.57 nm. XRD analysis confirmed the crystallinity and purity of the MgO nanoparticles. These bio-inspired MgO nanoparticles hold potential for various applications in cosmetics, including scrubs, moisturizers, and microdermabrasion, as well as in the development of effective drugs for maintaining protein structure and reducing inflammation in the body (Shahid et al., Citation2022).
Diclofenac sodium (DCF) is a monosodium salt of 2-[(2, 6-dichlorophenyl) amino] benzene acetic acid, which is used as a common nonsteroidal anti-inflammatory drug (NSAID) () (Sulaiman & Al-Jabari, Citation2021). Furthermore, the traces of DCF have been found in treated effluents and water resources up to µg. L−1 (Sulaiman & Al-Jabari, Citation2021).
Ozonation and photocatalysis are advanced oxidation processes that can be used to remove DCF sodium from aqueous solutions. However, they are limited by the dimerization of carboxylic acids, which prevents complete mineralization (Wu et al., Citation2016). Consequently, the effective removal of DCF from polluted water presents a substantial challenge.
The novelty in the current work is to investigate whether MgO nanoparticles (NPs) produced via the sol-gel process can simultaneously remove the pharmaceutical diclofenac sodium (DCF), cadmium ions (Cd2+) and inactivate bacterial from aqueous solutions. Subsequently, various techniques were employed to characterize, analyze and to confirm the physical and chemical properties of the adsorbents. The study delved into comparing the potential for removing DCF and Cd2+ by utilizing the surface of MgO with that of B-nZVI and activated carbon (AC) adsorbents (Sulaiman & Al-Jabari, Citation2021). The investigation assessed factors like pH, adsorbent dosage, temperature, concentration of adsorbate, and contact duration influence the removal efficiency of DCF and Cd2+.In order to explore how DCF and Cd2+ adhere to the surface of MgO NPs and B-nZVI composite, diverse kinetic and thermodynamic models were applied and relevant parameters were determined. The removal efficiency of DCF and Cd2+ was determined by employing a packed column filtration system with a composite of sand and MgO, and the efficiency was compared to that of B-nZVI and sand composite. In the end, we evaluated the efficiency of the nanoparticles as an antimicrobial agent using the well-agar diffusion technique against Gram-positive bacteria.
2. Experimental part
2.1. Chemicals
The chemicals used in the present work were obtained from Sigma Chemical Company without any additional processing. Deionized water was employed for all experiments, and all chemicals were of analytical grade and used without additional purification including Mg(NO3)2·6H2Owith a purity of ≥97%, FeCl3·6H2O, CdCl2·2.5H2O, C6H8O7, commercial-grade sodium chloride (NaCl), and pure diclofenac sodium. Bentonite (B) was powdered into 200-micrometer size and dried for a whole night at 25 °C before use. In column adsorption experiments FAC (Fine Activated Carbon) was employed in a batch experiment.
2.1.1. Synthesis of bentonite-supported nZVI
nZVI nanoparticles and bentonite-supported nZVI (B-nZVI) were synthesized using the liquid-phase reduction method in which bentonite acted as a support material as previously reported (Sulaiman & Shahwan, Citation2017).
B-nZVI was prepared with an iron/bentonite mass ratio of 1:1. For this purpose, 4.84 g of ferric chloride hexahydrate (FeCl3·6H2O) were dissolved in 50 ml of a mixture of distilled water and absolute ethanol (prepared at a volume ratio of 4:1), and 1.00 g of bentonite was added. The mixture was stirred with a stirring rod for 15 min, and then 100 mL of 0.47 M NaBH4 solution was added dropwise into this mixture and vigorously stirred continuously under nitrogen atmosphere. The mixture’s color turned from red brown to light yellow, and then eventually to black.
After all of the NaBH4 solution had been added, the mixture was stirred under the nitrogen atmosphere continuously for another 20 min to completely deplete NaBH4 and FeCl3·6H2O. Vacuum filtration was employed to collect the B-nZVI particles, and these were quickly rinsed three times with absolute ethanol. This step is necessary to prevent the immediate oxidation of nZVI. Finally, the composite samples were dried at 333 K overnight (Sulaiman & Shahwan, Citation2017).
2.2. Synthesis of MgO NPs
The sol–gel calcination process was used for generating MgO NPs. Initially, 0.04 mol of citric acid (C6H8O7) and magnesium nitrate (Mg (NO3)2·6H2O) were weighted separately. These chemicals were dissolved individually in 40 ml of distilled water, and mixed under continuous stirring to obtain a uniform solution. This combined solution was heated to 80 °C with constant stirring, leading to the formation of a homogeneous solution, which will then start to form a gel. After that, the resulting wet gel will be dried for a duration of 2 h at 150 °C in an oven, yielding a white and fluffy precursor. To produce highly effective MgO nanoparticles, this white fluffy precursor was crushed and heated at 600 °C at a rate of 2 °C.min−1 for an additional 2 h again (Cai et al., Citation2017; Purwajanti et al., Citation2015; Sulaiman & Al-Jabari, Citation2021). The alternative adsorbent (B-nZVI) was prepared following the previously applied procedure (Sulaiman & Al-Jabari, Citation2021).
2.2.1. Characterization
The vibrational spectra were recorded using a Fourier transform infrared spectrometer (FTIR, a Bruker TENSOR II Spectrometer) at room temperature in the range 200–4000 cm−1 (Sulaiman & Shahwan, Citation2017). The shapes and distribution of MgO, MgO-DCF, and MgO-Cd2+ were explored using a field emission scanning electron microscope (SEM-EDX, Jeol 6700LV), operating at 20 kV, and using a Transmission electron microscope (TEM, JEM-2100F) at an accelerating voltage of 200 kV. X-ray diffractions (XRD) patterns of the samples were acquired mini-XRD (Rigaku Ultima, Tokyo, Japan) with Cu-Kα radiation monochromatic source scanned at a rate of in the range from 5° to 80°.
The amounts of DCF and Cd2+ in water solutions were evaluated using a UV–vis Spectrophotometer (Hp 8453, Agilent, USA), The absorption of the solutions was measured across a wavelength spectrum ranging from 190 to 1100 nm. The pH solutions were adjusted by adding a proper amount of 0.1 M NaOH/HCl
2.3. Removal experiments
The efficiency of removing pollutants was investigated for three different adsorbents: MgO, B-nZVI, and FAC. All required solutions were formulated by dissolving the suitable quantities of DCF and Cd2+ substance in deionized water. To conduct the adsorption process, the batch method was applied to determine the efficiencies of adsorbents NPs removal. 0.1 g of each adsorbent was added to 100 ml solutions of 100 mg.L−1. Each solution was placed on a shaker operating at 125 rpm and 298K. Once equilibrium was reached, the remaining DCF and Cd2+ in the supernatant were separated using a syringe filter of 0.45 µm pore. The absorbance of contaminants was measured utilizing a UV–Vis spectrophotometer, the DCF was measured at λmax 276 nm and Cd2+ at 422 nm, then the concentrations of DCF and Cd2+ were calculated referring to their previously established calibration curves. In all experiments, the sample analysis was carried out in triplicate, and the average value was considered. Within the maximum error not exceeding 3.0%.
EquationEquation (1)(1)
(1) enables the calculation of the adsorption capacity of the adsorbent (qt, mg.g−1), considering that the initial concentration (C0, mg.L−1) of DCF and Cd2+ in the aqueous solution, the pollutant concentration in the aqueous solution at a specific time (Ct, mg.L−1), the mass of magnesium oxide (m, g), and the volume of the DCF and Cd2+ solution (V, L). Furthermore, EquationEq. (2)
(2)
(2) allows for the determination of the percentage removal efficiency (%R) of DCF and Cd2+.
(1)
(1)
(2)
(2)
To construct the calibration curve (Figure S1), a 1000 mg.L−1 standard solution was diluted to a series of solutions in the range of (5–100) mg.L−1.
2.3.1 pH effect
To see the influence of pH on the removal of contaminant, the pH of 100 ml solutions containing 25 mg. L−1 of DCF and 25 mg.L−1 Cd2+ was investigated. Adjustment of pH was achieved by introducing 0.1 M HCl and 0.1 M NaOH, which allowed the pH to maintain in the desired range of 2 to 9. Following the pH adjustment, the solutions were shaken subjected to shaking at of 125 rpm for 3 h at 298 K.
2.3.2. Effect of MgO concentration
The removal efficiency for MgO NP removal was investigated by conducting multiple batches with varying initial MgO amounts (100, 250, 500, and 1000 mg) in a solution containing 100 mg.L−1 of both DCF and Cd2+. The mixtures were Shaken at 125 rpm and 298 K. Subsequently, the samples were filtered through a 0.45 µm filter, and then the UV–Vis spectrophotometer was used to measure the absorbance of the solution before and after adsorption at wavelengths 276 and 422 nm, respectively.
2.3.3. Adsorption isotherm
To investigate the parameters of adsorption isotherms, several experiments were conducted in a batch setup using two distinct adsorbates: DCF and Cd2+. In each experiment, 0.1 g of the adsorbent MgO NPs was introduced into 100 mL aqueous solutions having various initial concentrations in the range 5–100 mg.L−1. The solutions were then shaken with a water bath shaker at 125 rpm and 298K. The absorbance of the solutions after filtration was measured and monitored at the time intervals, ranging from 0 to 180 min. Finally, the removal efficiency was determined using EquationEq. (2)(2)
(2) .
2.3.4. Effect of temperature
0.1g of MgO NPs was separately added to 100 ml aqueous solutions containing 25 mg.L−1 of both Cd2+and DCF, and then, the samples were shaken using a temperature-controlled bath shaker, at 125 rpm, and at 288K. Subsequently, the adsorbed amount of DCF and Cd2+ was analyzed at different contact times. The process was replicated at 298, 308, and 318 K, respectively.
2.4. Column experiments
Column experiments were set up to simulate the applicable and validate the efficiency of the respective nanomaterials in eliminating persistent pollutants.
A combination of MgO/B-nZVI and quartz sand was formulated at the ratio of (98:2, w/w) to prepare a layer of 20 cm thickness. This mixture was then introduced into columns of 5 cm diameter and 25 cm length. Furthermore, at the bottom of the columns, a 3 cm layer of processed quartz sand was placed. Before utilization, the quartz sand was washed with de-ionized water and dehydrated at 110 °C for a duration of 24 h. The solution of DCF and Cd2+ (0.01, 0.1, 10, 100 mg L−1) were prepared, and each of these solutions was introduced at 2.0 mL.min−1 to flow through the columns. The concentrations of DCF and Cd2+ remaining in the elute fractions were finally determined.
2.5. Antibacterial test
The efficiency of the nanoparticles as an antimicrobial agent was evaluated using the well-agar diffusion technique against Gram-positive bacteria, namely Enterococcus faecalis, Staphylococcus epidermidis, Micrococcus Luteus, Bacillus subtilis and Staphylococcus aureus. To prepare the composites MgO-DCF, MgO-Cd2+ and DCF-MgO-Cd2+, 0.1 g MgO was mixed with 100 ml of a 25 mg.L−1 adsorbate solution and stirred continuously at 125 rpm. After 300 min, the particles with adsorbed DCF and Cd2+ were collected and dried. Subsequently, the concentrations of adsorbed DCF and Cd2+ were measured by UV–vis spectrophotometry. The optimum concentrations of MgO, MgO-DCF, and MgO-Cd2+, DCF-MgO-Cd2+ were calculated. Each bacterial colony was suspended in sterile saline solution (0.9% NaCl) until the turbidity matched the McFarland 0.5 Standard. A sterile cotton swab was used to spread the bacterial inoculum on the surface of a Muller Hinton nutrient agar plate. Milli-Q water was used as the negative control.
The bacterial strains were evenly spread on Mueller-Hinton agar plates. A sterile probe was used to from a 6 mm well in each plate, and 50 μL of each antimicrobial agent was placed in the well. The plates were then incubated at 310 K for 24 h. Two samples were prepared for each strain, and the average diameter of the resulting inhibition zones was measured.
3. Results and discussion
3.1. Characterization analysis
The surface morphology of the nanoparticles were characterized via the FTIR, XRD, SEM, TEM and EDS. SEM and TEM techniques.
To analyze the synthesized MgO nanoparticles, which were subjected to calcination at 800 °C, FE-SEM and TEM techniques were utilized (The images are depicted in ). SEM images in show samples with aggregate formations resembling plate-like flower structures. Lacking a cotton-like appearance (Dhal, Sethi, Mishra, & Hota, Citation2015). Further examination of the product’s morphology was conducted by TEM (), revealed the existence of irregular, hexagonal, cubic, and spherical particles with an average size of 10-20 nm in consistent with the previous results (Dhal et al., Citation2015).
The elemental composition of the MgO nanoparticles was determined by energy-dispersive X-ray spectroscopy (EDS). The EDS spectrum proved the predominant presence of magnesium (Mg) and oxygen (O), along with minor traces of carbon (C) and calcium (Ca) constituents (Figure S2).
Furthermore, the TEM and SEM images reveal that the nanoscale zero-valent iron (nZVI) particles, with an average size ranging between 40 and 60 nm, are dispersed in a random manner across the surface of the flakes ().
Figure 3. The X-ray diffraction (XRD) patterns of the MgO products synthesized using citric acid as a fuel and subjected to calcination at 800 °C.
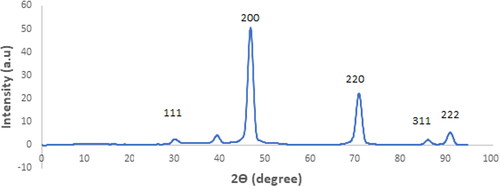
These findings were verified by the results obtained through the XRD analysis. X-ray diffraction (XRD) was used to investigate the phase composition of the synthesized MgO nanostructures. shows the XRD pattern of MgO nanoparticles calcined at 800 °C. Clear diffraction peaks were identified at 2θ values of 29.2°, 46.4°, 70.1°, 86.0°, and 90.7°, which correspond to the crystallographic planes (111), (200), (220), (311), and (222) of a face-centered cubic (FCC) arrangement of MgO (JCPDS No. 89-7746) respectively. Thus, the sharpness and clarity of XRD peaks are strong indicators of both the crystallinity and homogeneity of MgO nanoparticles, confirming their high-quality structural properties ().
Employing citric acid as a chelating agent led to the formation of pristine MgO nanostructures during the calcination process at 800 °C. The resultant crystallite size was estimated to be within the range of 10–20 nm. Additionally, an augmentation in the calcination temperature was noted to amplify the overall crystallinity of the end product. However, all of these of evidences align to emphasize the crystalline characteristics of the magnesium oxide nanoparticles (Afsin & Macit, Citation1998; Dhal et al., Citation2015).
The chemical compositions of the products were examined after calcination at 800 °C recording the corresponding FT-IR spectra (). The vibrational spectra reveal the absorptions ranging from 440 to 700 cm−1, indicating the stretching modes related to the Mg-O bond. Additionally, the bands observed in the range 840–900 cm−1 correspond to the characteristic stretching vibrations (υ1 and υ2) of magnesium-oxygen bond.
Figure 4. The FT-IR spectra of the MgO nanostructures that were produced and subsequently calcined at 800 °C, using citric acid (a) diclofenac sodium (b) MgO and (c) MgO and diclofenac sodium.
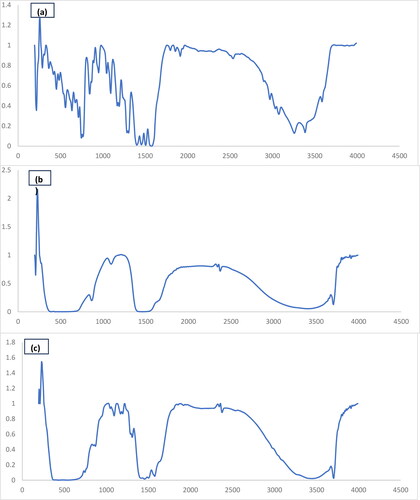
The multiplicity of the spectral features detected in the regions 3290–3650 and 1598–1600 cm−1 can be associated with the stretching and bending vibrations of hydroxyl groups of the water species adsorbed on the surface of the nanoparticles. These findings are in accordance with the data found by Dhal et al. (Citation2015).
The FTIR spectrum of DCF shows the C–C vibrational stretching band of the aromatic ring at 1504 cm−1. In addition, the vibrational bands of C–Cl are located at 766 and 745 cm−1. The most prominent absorption bands at 1452 and 1574 cm−1 originate from the symmetric and asymmetric vibrational stretching of COO–, respectively. The absorption peak at 3386 cm−1 attributed to N-H stretching, whereas the peaks at 1168 and 1192 cm−1 ascribed to the C-N stretching in secondary amines signify an appreciable change in the adsorption geometry in parallel with the evolution of the discrete DCF entities on the MgO surface (Dhal et al., Citation2015).
3.2. Removal efficiency study
An investigation was conducted to compare the adsorption capacities of B-nZVI, activated carbon and MgO nanoparticles. The effect of contact time on adsorption efficiency by these materials was investigated for the removal of DCF and Cd2+ at pH 7. 0.1 g of each adsorbent was treated with the pollutant of the initial concentrations 100 mg.L−1.
illustrates the removal efficiency of the contaminants in duration changing from 5 to 180 min. It may be seen from this figure that the adsorption capacity of the MgO adsorbent is higher than the other adsorbents, with around 90 and 80% removal for DCF and Cd2+, respectively. The removal efficiencies for the other two adsorbents were found as follows: B-nZVI exhibited approximately 66% removal for DCF and 70% for Cd2+, while activated carbon removed DCF and Cd2+ by 42 and 44%, respectively. Based on these results, the best contact time for the removal of both pollutants may be suggested as 120 min. Once equilibrium was reached, the adsorption process became stable. The notable removal efficiency of MgO nanoparticles toward DCF and Cd2+ confirms the excellent dispersibility of MgO, which facilitates the adsorption efficiency in comparison to the other two adsorbents.
Figure 5. DCF and Cd2+ removal efficiency from aqueous solution using B-nZVI composite (●), activated charcoal (▲), MgO (■) versus time, adsorbent dose 0.1 g, C0100 mg L−1, pH 7, and T = 25 °C.
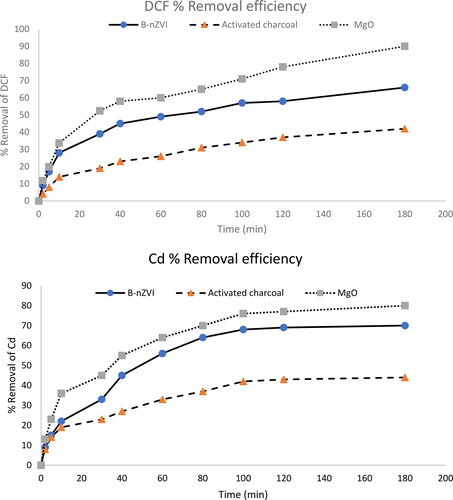
Based upon the present removal efficiency data, extensive studies were conducted to study the adsorption behavior of DCF and Cd2+ on as-prepared MgO (Al-Degs, El-Barghouthi, El-Sheikh, & Walker, Citation2008; Nassar & Abdallah, Citation2016; Nassar & Khatab, Citation2016).
3.2.1. pH effect
We conducted an investigation to understand how the preliminary pH of the solution affects the adsorption efficiency of DCF and Cd2+by MgO nanoparticles. shows that the amounts of removed DCF and Cd2+ are low at low pH values. However, when pH increased, the amount of DCF removed increase and reaches its maximum ratio of 85% at pH 7, and Cd2+ reaches the maximum removal ratio of 65%.
The efficiency of MgO nanoparticles to remove DCF and Cd2+ decreases at pH values above 7. This is the surface charge of the nanoparticles at different pH intervals. At lower pH values, the surfaces of the particle adsorbents (hydrous oxide) (MOH) are likely to be coated with protons which result in positively charged (MOH+2) nanoparticles, attracting the negatively charged DCF and Cd2+ molecules. However, at higher pH values the reaction of hydrous oxide with hydroxide ions become more likely to occur and makes the nanoparticles negatively charged (MO–), which repels the DCF molecules and Cd2+ ion (Mahmoud, Ibrahim, & El-Molla, Citation2016; Salem, Ahmed, & El-Shahat, Citation2016).
DCF is an anionic molecule, that is, it has a negative charge. MgO has a zero charge point of 12.4 (Mahmoud et al., Citation2016; Salem et al., Citation2016), which indicate that it has no net charge at this pH. This means that the adsorption of DCF on MgO is likely to occur due to the electrostatic interactions between negatively charged DCF molecules and positively charged MgO particles. In addition, the competition between the negatively charged DCF molecules and the highly concentrated OH − ions favours the lower adsorption efficiency at higher pH values. The OH − ions are also negatively charged and therefore compete with the DCF molecules for adsorption sites on MgO surface. This competition decreases the amount of DCF to be adsorbed. Therefore, pH 7 may be suggested as the optimum value for the adsorption of DCF and Cd2+by MgO particles (Pavan, Dias, Lima, & Benvenutti, Citation2008; Wang, Zhu, & Yin, Citation2008).
3.2.2. Effect of MgO concentration
displays the outcomes of MgO dosage variation. The results demonstrated that the removal of DCF and Cd2+ was maximized when the amount of MgO used was 0.25 g. This may be ascribed to the larger surface area of MgO providing more adsorption sites for the DCF molecules and Cd2+ molecules to bind (Mittal, Kurup, & Gupta, Citation2005).
3.2.3. Kinetic and adsorption isotherm
The adsorption capacity of DCF and Cd2+ in the presence of 0.1 g MgO adsorbent was investigated at different initial pollutant concentrations (5–100 mg.L−1) at 25 °C. shows that the adsorption capacity increased with increasing initial pollutant concentration and was found to be stable at about 30 mg.L−1 for both pollutants. The increase in adsorption capacity with increasing initial pollutant concentration can be explained by the stronger driving forces for adsorption (e.g. concentration gradient, surface energy and affinity), which overcome the resistance of mass transfer between the liquid and solid phases (Hu & Wang, Citation2016). However, the removal efficiency decreases by about 30% when the pollutant concentration exceeds 30 mg L−1. This is because the adsorbent can only adsorb a certain amount of pollutants before the surface sites become saturated. At too high pollutant concentrations, the pollutant molecules compete for the available surface sites, which leads to a decrease in removal efficiency.
Figure 8. The effect of DCF and Cd2+ initial concentration on the removal efficiency using MgO nanoparticles.
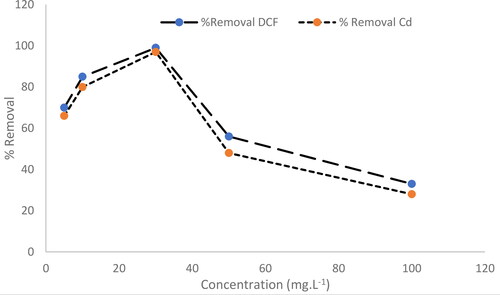
Adsorption kinetics process of DCF and Cd2+ was investigated using a composite of MgO and B-nZVI. The modified pseudo-first-order rate equation (EquationEq. (3)(3)
(3) ) and the modified pseudo-second-order rate equation (EquationEq. (4)
(4)
(4) ) were used to analyze the process and evaluate the kinetic parameters (Banerjee & Chattopadhyaya, Citation2017).
(3)
(3)
(4)
(4)
The equations have several parameters, where C0 is the initial concentration, Qe (mg.g−1) is the equilibrium mass of DCF and Cd2+ adsorbed per gram of adsorbent, and Qt is the amount of adsorbate at time t. The rate constant is denoted as k2. We have plotted t/Q against t as shown in . The results show that the second-order kinetic model with a linear regression correlation coefficient (R2) greater than 0.99 was a good fit for both adsorbents. In contrast, the pseudo-first-order model (ln (Qe − Qt) versus t) did not give a good fit for the adsorption data, as shown by the low linear regression correlation coefficient (R2) of less than 0.90.
Figure 9. Plotting the pseudo-second-order kinetic parameters for the removal of DCF and Cd2+ by MgO. (●) DCF and Cd2+ (■).
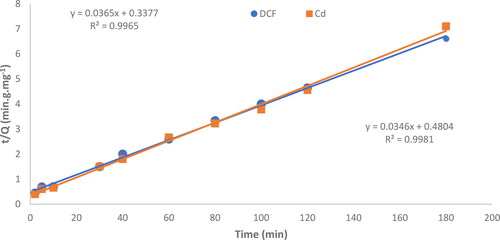
The adsorption isotherm was used to describe how the adsorbate is distributed in equilibrium between the solution and the adsorbent. Both the Langmuir and Freundlich isotherm models have been used to understand the relation between the adsorption mechanism on the adsorbent surface and its uniformity (Afshin, Rashtbari, Shirmardi, Vosoughi, & Hamzehzadeh, Citation2019; Radnia, Ghoreyshi, Younesi, & Najafpour, Citation2012). The linearized form of the Langmuir adsorption isotherm equation was used to fit the experimental data (EquationEq. (5)(5)
(5) ).
(5)
(5)
where Qm is the maximum quantity of DCF and Cd2+ that can be adsorbed per gram of adsorbent (mg/g), equivalent to the maximum quantity of adsorbate that can from a monolayer on the surface which is considered as the measure of the adsorbent’s ability to bind to the adsorbate. The Langmuir constant, KL represents the affinity between the adsorbate and the adsorbent and it is related to the energy required to break the bonds between the adsorbate and the solvent and to form new bonds between the adsorbate and the adsorbent. It is expressed in units of liters per milligram (L.mg−1).
The Freundlich isotherm model can be used to describe multilayer adsorption, which is applicable to heterogeneous adsorbent surfaces having different adsorption sites and energies (Al-Jabari et al., Citation2019; Thilagan, Gopalakrishnan, & Kannadasan, Citation2013). The linearized form of the Freundlich isotherm is given by the following equation:
(6)
(6)
The Freundlich constant kf indicates the amount of adsorbate that can be adsorbed onto the adsorbent at a given equilibrium concentration, and the exponential constant n (adsorption intensity) is a measure of the heterogeneity of the adsorbent surface. Higher n value indicates more heterogeneous surface. The experimental data for the adsorption of DCF and Cd2+ onto the composite of MgO and B-nZVI were analyzed using EquationEqs. (1)(1)
(1) and Equation(2)
(2)
(2) and the values of these constants are presented in .
Table 1. The Langmuir isotherm model was utilized to obtain values of K, qmax, and the coefficient of determination (R2) for the removal of both DCF and Cd2+ using the B-nZVI composite at 25 °C.
The results show that the Langmuir model is a good approximation of the actual adsorption process. shows that Langmuir’s model fits the data better than Freundlich’s model, as evidenced by the higher R2 values. This indicates that the adsorption of DCF and Cd2+ on the MgO and B-nZVI composite mainly occurs in a monolayer, suggesting a homogeneous rather than a heterogeneous adsorption process. The maximum amounts of DCF and Cd2+ that can be adsorbed by MgO were determined as 66.2 and 50.8 mg.g−1, respectively. The maximum amounts of DCF and Cd2+ adsorbed by the B-nZVI composite were determined as 46.5 and 43.3 mg.g−1, respectively.
Figure 10. Linear graphs representing the Langmuir isotherm model for the adsorption of DCF and Cd2+ using MgO nanoparticles and the B-nZVI composite at of 298 K, respectively.
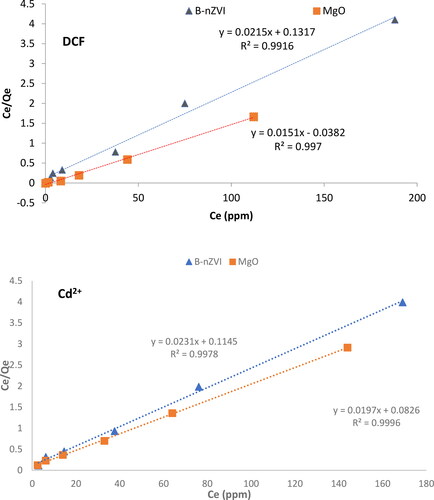
These results also show that MgO has a higher affinity and capacity for removing DCF and Cd2+ than the other known adsorbents. For example, it has been found in a previous study that MgO nanoparticles can remove 97.42% of Malachite green and 92.68% of Congo red (Dhal et al., Citation2015). Another study showed that the maximum adsorption capacity of DCF (Qmax) onto Fe0NPs and Fe0-PG composite was 23.1 and 48.1 mg.g−1, respectively (Al-Rimawi, Al-Jabari, Sulaiman, Nazal, & Idrees, Citation2022). Additionally, MgO seems more efficient in the removal of pollutants than B-nZVI. The improved adsorption capabilities of MgO nanoparticles can be attributed to the reduced aggregation of the particles and thus, the increased surface area (Al-Jabari et al., Citation2019; Sulaiman & Al-Jabari, Citation2021).
3.2.4. Effect of temperature
The effect of temperature on the adsorption capacity of MgO was examined. shows that the adsorption efficiency of both pollutants increases up to 298 K and then decreases slightly as the temperature is increased further. The % removal of DCF and Cd2+ at 288 K was determined as 70 and 66%, respectively. At 298 K, the percentage of removal increased significantly to 98 and 95%, respectively. Which indicate that the adsorption process on MgO is endothermic. The slight decrease in the adsorption ratio at higher temperatures can be attributed to the tendency of the pollutant molecules to return from the solid to the liquid phase at equilibrium by overcoming the weak attraction between the adsorbate and adsorbent (Venkatesha, Viswanatha, Arthoba Nayaka, & Chethana, Citation2012).
3.3. Thermodynamic parameters
The thermodynamic parameters of the adsorption of DCF and Cd2+ on MgO nanoparticles, namely the changes in standard enthalpy (ΔH°), standard entropy (ΔS°) and standard Gibbs free energy (ΔG°), were determined using the following equations.
(7)
(7)
where, Rd = [qe/Ce]
(8)
(8)
(9)
(9)
The accurate determination of parameters in sorption studies is challenging due to several factors. One limitation is that the Rd parameter, commonly used as an empirical constant, does not represent a thermodynamic equilibrium constant specific to a particular set of reaction conditions. Additionally, the calculated value of Rd is affected by the initial concentration (Sulaiman & Al-Jabari, Citation2021).
To evaluate the thermodynamic parameters of adsorption, equilibrium was achieved by varying the initial concentrations of DCF and Cd2+ solutions using 0.1 g of MgO nanoparticles at different temperatures. The values of ΔH°, ΔS°, and ΔG° were calculated and presented in .
Table 2. The thermodynamic parameters determined at various concentrations.
The negative value of ΔH° means that the adsorption process is exothermic, i.e. heat is released. This suggests that DCF and Cd2+ are more likely to adsorb the surface at temperatures lower than 298 K. In an exothermic process, increasing the temperature reverses the natural direction of the reaction, thus, making it the interaction of DCF and Cd2+ with the adsorbent surface less likely.
The experimental data was used to determine the standard Gibbs free energy change (ΔG°) of adsorption at different initial concentrations. As shown in , the negative ΔG° value indicates that the adsorption of DCF and Cd2+ on the surface of MgO nanoparticles occurs spontaneously, indicating. That the adsorption equilibrium formation of adsorbed DCF and Cd2+ is favoured.
The values of adsorption enthalpy for DCF (ΔH° = −14.77 kJ.mol−1) and Cd2+ (ΔH°= −9.56 kJ.mol−1) are smaller than the values typically associated with chemical bond formation (ΔH°> 50 kJ.mol−1). This suggests that physisorption rather than chemisorption has occurred (Sulaiman & Al-Jabari, Citation2021).
Based the ΔG° and ΔH° values, the standard entropy (ΔS°) of the adsorption process were determined using EquationEq. (9)(9)
(9) . The negative ΔS° value indicates that as adsorption takes place, the adsorbate molecules will have less energetic freedom to move at the liquid-solid interface.
3.4. Column experiment
The DCF and Cd2+ solutions were filtered using a 1:49 w/w combination of MgO nanoparticles and extra sand. The results, summarized in reveal that the MgO filter removed both pollutants more effectively than the alternative adsorbent B-nZVI. Furthermore, the (MgO: sand) filter is more effective in removing DCF than Cd2+. A potential approach for water filtration in tertiary treatment systems is the use of (MgO: sand) filters. The findings suggest that the efficacy of removal of persistent NSAID and heavy metal residues from wastewater treatment facilities already in use might be increased by addition of MgO sand filters.
Table 3. The filtration procedure involved the passage of 1 liter of pure water solutions containing DCF and Cd2+ at concentrations of 100, 10, 1.0 and 0.01 mg.L−1 through laboratory filters.
3.4.1. Antibacterial activity
The study investigated the reusability of adsorbed DCF and Cd2+ as well as the antibacterial efficiency of MgO nanoparticles against several bacterial strains (Figure S3, ). The size of the inhibition zone surrounding a well reveals the potency of a certain DCF or Cd2+ concentration against a specific bacterial strain. The larger the inhibition zone, the more effective the treatment.
Table 4. Antibacterial activity of Cd2+, DCF, MgO, MgO + DCF, MgO + Cd2+, MgO + Cd2+ + DCF, levo the positive control to gram-positive bacteria and the negative control (water) in relation to the inhibition zone using the agar well diffusion method.
The effectiveness of cadmium ions (Cd2+) against different types of bacteria was investigated. Levofloxacin, a known antibiotic against Gram-positive bacteria, was used as positive control. Neither magnesium oxide (MgO) nor water (H2O) had any antibacterial activity against the bacteria tested. However, Cd2+ was very effective against all the bacterial strains, with clear zones around the wells ranging from 23.8 to 36.8 mm in diameter.
Diclofenac (DCF) was effective against all the bacteria tested, except for two types: M. luteus and E. faecalis. These two types of bacteria did not show any antibacterial activity against other microorganisms. The clear zones around the wells ranged from 16.7 to 25.8 mm in diameter.
The combination of magnesium oxide (MgO) and diclofenac (DCF) was effective against two types of bacteria: B. subtilis and S. epidermidis. The combination of MgO and cadmium ions (Cd2+) was effective against all the bacteria tested except M. luteus, with clear zones around the wells ranging from 16.2 to 24.5 mm in diameter. The combination of MgO, Cd2+, and DCF was effective against all the bacteria tested except S. aureus, with clear zones around the wells ranging from 20 to 29.3 mm in diameter.
The results also show that the effect of diclofenac (DCF) and cadmium ions (Cd2+) changes after they are adsorbed onto the surface of magnesium oxide (MgO), comparing to their pure forms. Although the MgO-DCF-Cd composite is not as effective as pure DCF and Cd2+, the biological activity test confirms that DCF and Cd2+ will still have an influence on bacterial life in aquatic environments.
The magnesium hydroxide that developed in the presence of MgO might be responsible for the reduction in Cd2+ activity. Some of the Mg(OH)2 then breaks down to release hydroxide ions (OH−), which react with cadmium ions (Cd2+) to form insoluble cadmium hydroxide (Cd(OH)2) (Afsin & Macit, Citation1998). At the same time, Gram-positive bacteria are killed and turned to minerals, which release inorganic ions such as CO32−. These inorganic ions combine with Cd2+ in the solution to form insoluble CdCO3.
The potential of MgO nanoparticles in addressing environmental pollution and regulating antibiotic release in drug delivery systems seems promising for the recovery and reuse of pharmaceuticals and heavy metals. The newly synthesized MgO nanoparticles in the present study may provide a valuable channel for these applications.
5. Conclusion
MgO nanoparticles (NPs) prepared using the sol-gel method were characterized and used to remove Cd2+ ions and DCF from water. The controlled aggregation of MgO NPs resulted in better performance regarding removal efficiency of DCF and Cd2+from aqueous solutions when compared to B-nZVI, and AC.
Based upon the examination of various factors, the adsorption of DCF and Cd2+ was best described by the pseudo-second-order kinetics and Langmuir’s isothermal model for both adsorbents. The value of the adsorption enthalpy indicates the physisorption mechanism the removal of DCF and Cd2+ from an aqueous solution. In addition, it was found that the highest removal efficiency of the adsorbates was achieved at pH 7.0.
The thermodynamic data analysis proved that the removal of DCF and Cd2+ occurred spontaneously and more favorable at elevated temperatures, indicating an exothermic process with a negative entropy value. Moreover, in column filtration experiments, the MgO and sand composite exhibited a considerable advantage over the B-nZVI and sand composite in removal of DCF and Cd2+ from aqueous solution. The DCF and Cd2+ adsorbed on MgO were tested against different gram-positive bacterial strains, revealing that MgO and Cd2+ display antibacterial activity against a variety of strains.
The present results suggest that the utilization of MgO nanoparticles which have the advantages of preparation, low-cost, environmentally friendly, and framework for high-efficiency pollutant removal holds great promise as a new material for treating wastewater contaminated with pharmaceuticals and heavy metals.
Abbreviations | ||
MgO | = | Magnesium oxide |
NPs | = | Nanoparticles |
DCF | = | Diclofenac sodium |
UV | = | Spectroscopy ultra violet spectroscopy |
FTIR | = | Fourier transform infrared spectroscopy |
EDX | = | Energy-dispersive X-ray spectroscopy |
SEM | = | Scanning electron microscopy |
TEM | = | Transmission electron microscopy |
XRD | = | X-ray diffraction |
AC | = | Activated carbon |
B-nZVI | = | Bentonite-nano zero valent iron |
ROS | = | Reactive oxygen species |
FAC | = | Fine Activated Carbon |
UV–vis | = | Spectrophotometer ultra violet visible spectrophotometer |
nZVI | = | Nano zero-valent iron |
FCC | = | Face-centered cubic |
SAED | = | Selected area electron diffraction |
Fe0-PG | = | Iron zero pencil graphite |
ΔH° | = | Standard enthalpy change |
ΔS° | = | Standard entropy change |
ΔG° | = | Standard Gibbs free energy change |
NSAID | = | Nonsteroidal anti-inflammatory drugs |
Supplemental Material
Download PDF (691.3 KB)Acknowledgment
The authors would like to acknowledge the financial support of the Scientific Research Committee of Birzeit University. The authors also thank their colleagues at Birzeit University: A.N. Dudin, and Mohammad Samhan for their technical support and kind help.
Disclosure statement
The authors declare that they have no financial conflicts of interest or personal relationships that could influence the impartiality of the results reported in this paper.
References
- Al-Rimawi, N. L., Al-Jabari, M. H., Sulaiman, S. M., Nazal, M. K., & Idrees, A. S. (2022). Pencil graphite synergistic improvement of zero-valent iron composite for the removal of diclofenac sodium in aqueous solutions: Kinetics and comparative study. Advanced Powder Technology, 33(6), 103610. doi:10.1016/j.apt.2022.103610
- Afshin, S., Rashtbari, Y., Shirmardi, M., Vosoughi, M., & Hamzehzadeh, A. (2019). Adsorption of Basic Violet 16 dye from aqueous solution onto mucilaginous seeds of Salvia sclarea: Kinetics and isotherms studies. Desalination and Water Treatment, 161, 365–375. doi:10.5004/dwt.2019.24265
- Afsin, B., & Macit, M. (1998). Weakly chemisorbed ammonia species at a Cu (110) surface. Phys. Low-Dimens. Struct, 3-4, 191–198.
- Al-Degs, Y. S., El-Barghouthi, M. I., El-Sheikh, A. H., & Walker, G. M. (2008). Effect of solution pH, ionic strength, and temperature on adsorption behavior of reactive dyes on activated carbon. Dyes and Pigments, 77(1), 16–23. doi:10.1016/j.dyepig.2007.03.001
- Al-Jabari, M. H., Sulaiman, S., Ali, S., Barakat, R., Mubarak, A., & Khan, S. A. (2019). Adsorption study of levofloxacin on reusable magnetic nanoparticles: Kinetics and antibacterial activity. Journal of Molecular Liquids, 291, 111249. doi:10.1016/j.molliq
- Al-Jabari, M., Imtiaz, K., Sulaiman, S., Alawi, I., & Shilo, J. (2018). Synthesis, characterization, kinetic and thermodynamic investigation of silica nanoparticles and their application in Mefenamic acid removal from aqueous solution. Desalination and Water Treatment, 129, 160–167. doi:10.5004/dwt.2018.23083
- Banerjee, S., & Chattopadhyaya, M. (2017). Adsorption characteristics for the removal of a toxic dye, tartrazine from aqueous solutions by a low-cost agricultural byproduct. Arabian Journal of Chemistry, 10, S1629–S1638. doi:10.1016/j.arabjc.2013.06.005
- Cai, Y., Li, C., Wu, D., Wang, W., Tan, F., Wang, X., … Qiao, X. (2017). Highly active MgO nanoparticles for simultaneous bacterial inactivation and heavy metal removal from aqueous solution. Chemical Engineering Journal, 312, 158–166. doi:10.1016/j.cej.2016.11.134
- Chen, Y., He, M., Wang, C., & Wei, Y. (2014). A novel polyvinyltetrazole-grafted resin with high capacity for adsorption of Pb (II), Cu (II) and Cr (III) ions from aqueous solutions. Journal of Materials Chemistry A, 2(27), 10444–10453. doi:10.1039/c4ta01512f
- Choe, J. K., Bergquist, A. M., Jeong, S., Guest, J. S., Werth, C. J., & Strathmann, T. J. (2015). Performance and life cycle environmental benefits of recycling spent ion exchange brines by catalytic treatment of nitrate. Water Research, 80, 267–280. doi:10.1016/j.watres.2015.05.007
- Dhal, J. P., Sethi, M., Mishra, B. G., & Hota, G. (2015). MgO nanomaterials with different morphologies and their sorption capacity for removal of toxic dyes. Materials Letters, 141, 267–271. doi:10.1016/j.matlet.2014.10.055
- Fominykh, K., Feckl, J. M., Sicklinger, J., Döblinger, M., Böcklein, S., Ziegler, J., … Bein, T. (2014). Ultrasmall dispersible crystalline nickel oxide nanoparticles as high-performance catalysts for electrochemical water splitting. Advanced Functional Materials, 24(21), 3123–3129. doi:10.1002/adfm.201303600
- Guangyu, Y., & Thiruvenkatachari, V. (2003). Heavy-metal removal from aqueous solution by fungus Mucor rouxii. Water Research, 37, 4486–4496.
- Hu, D., & Wang, L. (2016). Adsorption of amoxicillin onto quaternized cellulose from flax noil: Kinetic, equilibriumand thermodynamic study. Journal of the Taiwan Institute of Chemical Engineers, 64, 227–234. doi:10.1016/j.jtice.2016.04.028
- Huang, M. R., Lu, H. J., & Li, X. G. (2012). Synthesis and strong heavy-metal ion sorption of copolymer microparticles from phenylenediamine and its sulfonate. Journal of Materials Chemistry, 22(34), 17685–17699. doi:10.1039/c2jm32361c
- Jin, T., & He, Y. (2011). Antibacterial activities of magnesium oxide (MgO) nanoparticles against foodborne pathogens. Journal of Nanoparticle Research, 13(12), 6877–6885. doi:10.1007/s11051-011-0595-5
- Jin, Z., Jia, Y., Zhang, K., Kong, L., Sun, B., Shen, W., … Liu, J. (2016). Effective removal of fluoride by porous MgO nanoplates and its adsorption mechanism. Journal of Alloys and Compounds, 675, 292–300. doi:10.1016/j.jallcom.2016.03.118
- Kang, T., Park, Y., Choi, K., Lee, J. S., & Yi, J. (2004). Ordered mesoporous silica (SBA-15) derivatized with imidazole-containing functionalities as a selective adsorbent of precious metal ions. Journal of Materials Chemistry, 14(6), 1043–1049. doi:10.1039/b315829b
- Krishnamoorthy, K., Manivannan, G., Kim, S. J., Jeyasubramanian, K., & Premanathan, M. (2012). Antibacterial activity of MgO nanoparticles based on lipid peroxidation by oxygen vacancy. Journal of Nanoparticle Research, 14(9), 1063. doi:10.1007/s11051-012-1063-6
- Liang, Y., Zhang, Y., Wang, Y., Zhang, H., & Liu, J. (2015). High flux, positively charged loose nanofiltration membrane by blending with poly (ionic liquid) brushes grafted silica spheres. Journal of Hazardous Materials, 287, 373–383. doi:10.1016/j.jhazmat.2015.01.057
- Mahmoud, H. R., Ibrahim, S. M., & El-Molla, S. A. (2016). Textile dye removal from aqueous solutions using cheap MgO nanomaterials: Adsorption kinetics, isotherm studies and thermodynamics. Advanced Powder Technology, 27(1), 223–231. doi:10.1016/j.apt.2015.12.006
- Mbamba, C. K., Batstone, D. J., Flores-Alsina, X., & Tait, S. (2015). A generalised chemical precipitation modelling approach in wastewater treatment applied to calcite. Water Research, 68, 342–353. doi:10.1016/j.watres.2014.10.011
- Mittal, A., Kurup, L., & Gupta, V. K. (2005). Use of waste materials-bottom ash and de-oiled soya, as potential adsorbents for the removal of amaranth from aqueous solutions. Journal of Hazardous Materials, 117(2-3), 171–178. doi:10.1016/j.jhazmat.2004.09.016
- Moussavi, G., & Mahmoudi, M. (2009). Removal of azo and anthraquinone reactive dyes from industrial wastewaters using MgO nanoparticles. Journal of Hazardous Materials, 168(2–3), 806–812. doi:10.1016/j.jhazmat.2009.02.097
- Nassar, M. Y., & Abdallah, S. (2016). Facile controllable hydrothermal route for porous CoMn2O4 nanostructure: Synthesis, characterization, and textile dye removal from aqueous media. RSC Advances, 6(87), 84050–84067. doi:10.1039/C6RA12424K
- Nassar, M. Y., & Khatab, M. (2016). Cobalt ferrite nanoparticles via a template-free hydrothermal route as an efficient nano-adsorbent for potential textile dye removal. RSC Advances, 6(83), 79688–79705. doi:10.1039/C6RA12852A
- Pavan, F. A., Dias, S. L. P., Lima, E. C., & Benvenutti, E. V. (2008). Removal of Congo red fromaqueous solution by anilinepropylsilica xerogel. Dyes and Pigments, 76(1), 64–69. doi:10.1016/j.dyepig.2006.08.027
- Purwajanti, S., Zhou, L., Ahmad, N. Y., Zhang, J., Zhang, H., Huang, X., & Yu, C. (2015). Synthesis of magnesium oxide hierarchical microspheres: A dual-functional material for water remediation. ACS Applied Materials & Interfaces, 7(38), 21278–21286. doi:10.1021/acsami.5b05553
- Radnia, H., Ghoreyshi, A. A., Younesi, H., & Najafpour, G. D. (2012). Adsorption of Fe (II) ions from aqueous phase by chitosan adsorbent: Equilibrium, kinetic, and thermodynamic studies. Desalination and Water Treatment, 50(1-3), 348–359. doi:10.1080/19443994.2012.720112
- Salem, A.-N. M., Ahmed, M. A., & El-Shahat, M. F. (2016). Selective adsorption of amaranth dye on Fe3O4/MgO nanoparticles. Journal of Molecular Liquids, 219, 780–788. doi:10.1016/j.molliq.2016.03.084
- Sawai, J., Kojima, H., Igarashi, H., Hashimoto, A., Shoji, S., Sawaki, T., … Shimizu, M. (2000). Antibacterial characteristics of magnesium oxide powder. World Journal of Microbiology and Biotechnology, 16(2), 187–194. doi:10.1023/A:1008916209784
- Shahid, S., Ejaz, A., Javed, M., Mansoor, S., Iqbal, S., Elkaeed, E. B., … Nazim Sarwar, M. (2022). The anti-inflammatory and free radical scavenging activities of bio-inspired nano magnesium oxide. Frontiers in Materials, 9, 875163. doi:10.3389/fmats.2022.875163
- Shannon, M. A., Bohn, P. W., Elimelech, M., Georgiadis, J. G., Mariñas, B. J., & Mayes, A. M. (2008). Science and technology for water purification in the coming decades. Nature, 452(7185), 301–310. doi:10.1038/nature06599
- Stoimenov, P. K., Klinger, R. L., Marchin, G. L., & Klabunde, K. J. (2002). Metal oxide nanoparticles as bactericidal agents. Langmuir, 18(17), 6679–6686. doi:10.1021/la0202374
- Sulaiman, S., & Al-Jabari, M. (2021). Enhanced adsorptive removal of diclofenac sodium from aqueous solution by bentonite supported nanoscale zero-valent iron. Arab Journal of Basic and Applied Sciences, 28(1), 51–63. doi:10.1080/25765299.2021.1878655
- Sulaiman, S., & Shahwan, T. (2017). Mefenamic acid stability and removal from wastewater using bentonite-supported nanoscale zero-valent iron and activated charcoal. Desalination and Water Treatment, 97, 175–183. doi:10.5004/dwt.2017.21633
- Sulaiman, S., Khamis, M., Nir, S., Lelario, F., Scrano, L., Bufo, S. A., & Karaman, R. (2015). Stability and removal of spironolactone from wastewater. Journal of Environmental Science and Health. Part A, Toxic/Hazardous Substances & Environmental Engineering, 50(11), 1127–1135. doi:10.1080/10934529.2015.1047668
- Sulaiman, S., Scrano, L., Khamis, M., Nir, S., Bufo, S. A., & Karaman, R. (2016). Diazepam stability in wastewater and removal by advanced membranes technology, activated carbon and micelle- bentonite complex. Desalination and Water Treatment, 57(7), 3098–3106. doi:10.1080/19443994.2014.981225
- Sulaimana, S., Khamis, M., Nir, S., Lelario, F., Scrano, L., Bufo, S. A., & Karaman, R. (2014). Stability and removal of dexamethasone sodium phosphate from wastewater using different techniques. Environmental Technology, 35(13–16), 1945–1955. doi:10.1080/09593330.2014.888097
- Tang, Z. X., & Lv, B. F. (2014). MgO nanoparticles as antibacterial agent: Preparation and activity. Brazilian Journal of Chemical Engineering, 31(3), 591–601. doi:10.1590/0104-6632.20140313s00002813
- Thilagan, J., Gopalakrishnan, S., & Kannadasan, T. (2013). A comparative study on adsorption of copper (ii) ions in aqueous solution by; (a) chitosan blended with cellulose and cross linked by formaldehyde,(b) chitosan immobilised on red soil,(c) chitosan reinforced by banana stem fibre. International Journal of Applied Engineering & Technology, 3, 35–60.
- Venieri, D., Fraggedaki, A., Kostadima, M., Chatzisymeon, E., Binas, V., Zachopoulos, A., … Mantzavinos, D. (2014). Solar light and metal-doped TiO2 to eliminate water transmitted bacterial pathogens: Photocatalyst characterization and disinfection performance. Applied Catalysis B: Environment and Energy, 154, 93–101.
- Venkatesha, T. G., Viswanatha, R., Arthoba Nayaka, Y., & Chethana, B. K. (2012). Kinetics and thermodynamics of reactive and vat dyes adsorption on MgO nanoparticles. Chemical Engineering Journal and the Biochemical Engineering Journal, 198–199, 1–10. doi:10.1016/j.cej.2012.05.071
- Wang, X., Zhu, N., & Yin, B. (2008). Preparation of sludge-based activated carbon and its application in dye wastewater treatment. Journal of Hazardous Materials, 153(1-2), 22–27. doi:10.1016/j.jhazmat.2007.08.011
- Wetteland, C. L., Nguyen, N.-Y. T., & Liu, H. (2016). Concentration-dependent behaviors of bone marrow derived mesenchymal stem cells and infectious bacteria toward magnesium oxide nanoparticles. Acta Biomaterialia, 35, 341–356. doi:10.1016/j.actbio.2016.02.032
- Wu, D., Wang, W., Ng, T. W., Huang, G., Xia, D., Yip, H. Y., … Wong, P. K. (2016). Visible-light-driven photocatalytic bacterial inactivation and the mechanism of zinc oxysulfide under LED light irradiation. Journal of Materials Chemistry A, 4(3), 1052–1059. doi:10.1039/C5TA08044D
- Zhang, M., Gao, B., Yao, Y., Xue, Y., & Inyang, M. (2012). Synthesis of porous MgO-biochar nanocomposites for removal of phosphate and nitrate from aqueous solutions. Chemical Engineering Journal and the Biochemical Engineering Journal, 210, 26–32. doi:10.1016/j.cej.2012.08.052