ABSTRACT
To increase the sustainability of industrial wastewater treatment, biological sorbents have been explored for the removal of potentially toxic elements (PTEs). Algae are particularly attractive biosorbents due to their high concentration of negatively charged extracellular binding sites. Although there has been significant focus on dried algal sorbents for this reason, live algal sorbents may expand potential binding sites through intracellular accumulation and replenishment of active binding sites through algal growth. A barrier to the application of live algal sorbents in WWT is managing a sorbent’s metabolic requirements while achieving maximum PTE removal. Many reviews investigating the performance of live algal sorbents have focused on species-specific differences in PTE removal, without defining how intracellular detoxification mechanisms may be impacted by system conditions. The following review explores the physico-chemical conditions and sorbents qualities that affect the production of an integral intracellular ligand for PTE accumulation, phytochelatins (PCs). This review uniquely highlights how system conditions may support or inhibit the proper functioning of intracellular detoxification mechanisms governed by PCs. Opportunities for optimization and areas requiring further research are also suggested.
Introduction
Globally, there has been an increase in potentially toxic element (PTE) pollution in terrestrial (Chibuike & Obiora, Citation2014; Friedlova, Citation2010), freshwater (Islam, Ahmed, Raknuzzaman, Habibullah -Al- Mamun, & Islam, Citation2015; Lin, Li, Luan, & Dai, Citation2020; Ribeiro et al., Citation2018) and marine ecosystems (Boran & Altinok, Citation2010; Gaudry et al., Citation2007; Luo et al., Citation2020) due to the discharge of PTE-containing wastewaters (WWs). Though conventional wastewater treatment (WWT) methods, like chemical precipitation, ion exchange, adsorption, coagulation and reverse osmosis, may reduce the concentration of PTEs, these methods present several environmental and economic shortcomings (Ahluwalia & Goyal, Citation2007; Patterson, Citation1985; Sud, Mahajan, & Kaur, Citation2008). Namely, depending on the process employed, these systems can be expensive, energy-intensive, inefficient at PTE concentrations in the µg1–1 range, and may produce toxic by-products with no sustainable disposal options (Ahluwalia & Goyal, Citation2007; He & Chen, Citation2014). Biosorbents, produced from plants, bacteria, yeast, fungi, or, the subject of this review, algae, provide more sustainable and cost-effective alternatives to conventional PTE WWT methods (Lata, Singh, & Samadder, Citation2015; Sud, Mahajan, & Kaur, Citation2008).
Algal biosorbents are particularly strong candidates for PTE removal due to their resilience to environmental stressors and their high concentration of extracellular sorption sites (Lin, Li, Luan, & Dai, Citation2020). These qualities allow algae to accumulate PTEs multiple orders of magnitude higher than ambient concentrations, even when PTEs exist at mg l−1 concentrations, with order of magnitude varying depending on algae species and PTE concentration (Foster, Citation1982; Stevens, Mccarthy, & Vis, Citation2001; Zbikowski, Szefer, & Latała, Citation2007). In a WWT context, the total PTE accumulation and removal achievable by an algal biosorbent depends upon the species, biosorbent dose, present PTEs, initial PTE concentration, exposure time, pH, temperature, presence of additional compounds, and biomass state (i.e., active or inactive), among other factors (Foster, Citation1982; Lin, Li, Luan, & Dai, Citation2020; Mehta & Gaur, Citation2005).
To date, many studies have utilized dried or inactive algal biomass for PTE accumulation. The current focus on inactive biomass is likely due to the perceived complications associated with live systems. In live algal WWT systems, uptake or accumulation may be impacted by the interaction of PTEs with ambient solution constituents, disparity in optimal pH for metal accumulation and biomass maintenance, and occurrence of biomass degradation due to chronic PTE exposure and desorbing agent application (Mehta & Gaur, Citation2005; Rajfur, Citation2013). However, a more recent review has identified live algal sorbents as able to achieve comparable levels of PTE removal (Zeraatkar, Ahmadzadeh, Talebi, Moheimani, & McHenry, Citation2016). This is because in dried systems, PTE removal is restricted to surface adsorption, whereas live algal sorbents may exploit surface adsorption as well as intracellular storage of PTEs through active detoxification mechanisms. These detoxification mechanisms are a result of physiological responses following PTE exposure.
Physiological responses to PTE exposure include non-transcriptional alterations to the occurrence of extracellular (Croot, Moffett, & Brand, Citation2000; Pistocchi, Mormile, Guerrini, Isani, & Boni, Citation2000) and intracellular compounds (Cobbett & Goldsbrough, Citation2002; Sinaei, Loghmani, & Bolouki, Citation2018). Though both classes of compounds contribute to PTE tolerance through PTE entrapment, chelation, and detoxification, the fate of PTEs differs between classes (Cobbett & Goldsbrough, Citation2002; Gekeler, Grill, Winnacker, & Zenk, Citation1988; Perales-Vela, Peña-Castro, & Cañizares-Villanueva, Citation2006). Extracellular compounds, namely extracellular polymeric substances (EPSs), increase the passive binding and exclusion of ambient PTEs in the extracellular matrix. The role of EPSs in PTE sorption has already been evaluated in several research papers and reviews (Naveed et al. Citation2019; Ozturk, Aslim, Suludere, & Tan Citation2010, Citation2014). Whereas intracellular compounds, including phytochelatins (PCs) and glutathione (GSH), actively compartmentalize PTEs in inert and storable forms following PTE import into the cell (Pistocchi, Mormile, Guerrini, Isani, & Boni, Citation2000). There are several other classes of intracellular compounds that prevent oxidative damage during PTE exposure, including heat-shock proteins and antioxidants, however, these will not be covered in this review as they do not directly contribute to increases in PTE uptake, but instead function as tolerance mechanisms (Danouche, El Ghachtouli, El Baouchi, & El Arroussi, Citation2020).
The extent of extracellular or intracellular compound generation impacts the location and level of PTE storage, and subsequently PTE recovery potential. The production of these compounds forms the basis for extracellular and intracellular PTE detoxification and internal compartmentalization mechanisms in live algal sorbents. The role and production of EPSs, GSH, and PCs are summarized in .
Figure 1. A simplified diagram of potentially toxic element (PTE) detoxification by extracellular and intracellular ligands. Both passive and active entrance of PTEs into the cell are illustrated, as well as the potential EPS-PTE interactions that may occur during passive sorption. Active transport of PTEs into the cell have been illustrated with a generic transporter, though these may bePTE-specific. + symbols indicate the addition of an actor and not the relative charge. GSH = reduced glutathione,γ-glutamate-cysteine-glycine; GSH-PTE = PTE-loaded GSH; GST = glutathione S-transferase; PCS = phytochelatin synthetase; PC-PTE = PTE-loaded phytochelatin; EPS = Extracellular Polymeric Substances; ROS = reactive oxygen species; GSSG = oxidized glutathione.
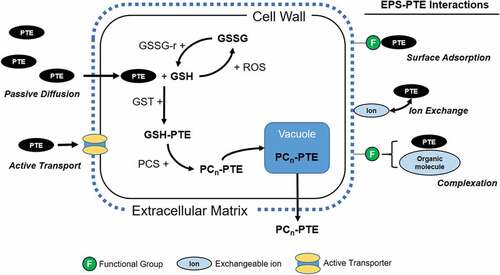
PCs, also known as class-III metallothioneins, and associated thiol peptides like GSH form the foundation for PTE detoxification and active uptake in live algal sorbents (Ahner & Morel, Citation1995a; Ahner, Wei, Oleson, & Ogura, Citation2002; Gekeler, Grill, Winnacker, & Zenk, Citation1988; Pawlik-Skowron, Citation2001; Perales-Vela, Peña-Castro, & Cañizares-Villanueva, Citation2006; Pérez-Rama, Torres Vaamonde, & Abalde Alonso, Citation2006; Soldo & Behra, Citation2000). PCs are present in bacteria, higher plants and most species of algae (Devez, Achterberg, & Gledhill, Citation2015; Perales-Vela, Peña-Castro, & Cañizares-Villanueva, Citation2006; Priyadarshanee & Das, Citation2021). Once PTEs are transported into the cell, GSH molecules chelate non-essential PTEs, or those occurring at toxic concentrations, through sulphhydryl (SH) complexes. GSH-PTE complexes then activate the production of phytochelatin synthetase (PCS) enzymes, which in turn catalyse the production of PCs from the GSH-PTE compounds (Perales-Vela, Peña-Castro, & Cañizares-Villanueva, Citation2006). PCs may exist in chains of 2 to 6 PC complexes (Gekeler, Grill, Winnacker, & Zenk, Citation1988; Kneer & Zenk, Citation1997) (). With increasing oligomer length, the PC-PTE complexes increase in stability, improving retention of PTEs and cellular tolerance (Kneer & Zenk, Citation1997; Lavoie, Le Faucheur, Fortin, & Campbell, Citation2009). In terms of PTE fate, PCs are stored in intracellular vacuoles or extruded, depending on ambient PTE concentrations (Cobbett, Citation2002; Perales-Vela, Peña-Castro, & Cañizares-Villanueva, Citation2006).
Figure 2. The chemical structure of glutathione (GSH) and the dimeric form of phytochelatin (PC). Amino acid constituents are colour-coded in both molecules.
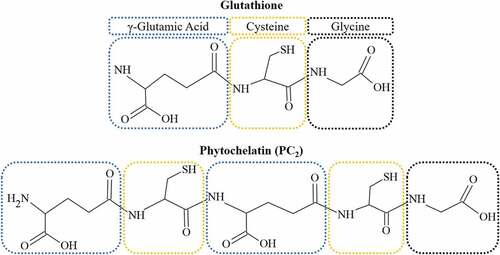
Whereas GSH mitigates oxidative damage under multiple cell stress events, PCs perform a specialized role in cellular detoxification by facilitating PTE entrapment (Oukarroum, Citation2016; Skowroński, De Knecht, Simons, & Verkleij, Citation1998). In terms of WWT and PTE remediation, PC content has been correlated to higher PTE tolerance and intracellular storage in multiple algal groups (Lavoie, Le Faucheur, Fortin, & Campbell, Citation2009; Navarrete et al., Citation2019; Skowroński, De Knecht, Simons, & Verkleij, Citation1998; Wang & Wang, Citation2011). Although PC content generally has a positive relationship to PTE exposure (), there are interspecific differences in PC synthesis and variable excitation based on physico-chemical conditions.
Table 1. Summary of phytochelatin (PC) response by multiple algae species following potentially toxic element (PTE) exposure. System conditions including present PTEs, PTE concentration, and exposure time are reported with units standardized where possible. PC content as well as the direction of PC change and the significance of this change are reported. PC content below the detection limit is reported as BD. When PCs are not detected, ND is reported in the PC content column. The Direction of PC change is determined from the difference between 0.0 mg PTE l−1 control and the experimental treatment. Direction of change may illustrate an increase (), decrease (
), or lack of change (
) in PC content. Significant difference between control and experimental cultures is marked with *. Significant difference between the PC content of two experimental treatments exposed to the same PTE concentration, but with different physico-chemical conditions is marked with
. The units for PC content are reported in the first entry for each evaluation. PTE concentrations have been converted to mg l−1 where necessary with original concentrations have been reported in red. Units that could not be converted are marked with ·. Additional notes on biomass qualities or physico-chemical conditions are included in the Notes column.
Many reviews to date compare the performance of live algal sorbents by evaluating the effects of sorbent species and physico-chemical conditions on PTE removal; however, they often fail to define the role of underlying physiological responses in these differences in performance. Whereas the following review focuses on how PC production is impacted by system conditions, including present PTEs, PTE concentration, exposure time, and physico-chemical conditions, and sorbent qualities, including sorbent group, species, and GSH production.
This critical review provides a comprehensive summary of the factors affecting PC production in different algae-PTE systems and highlights the opportunities for optimization through algal sorbent and physico-chemical condition selection for effective PTE removal. This review evaluates the latest research on PC production by micro- and macroalgae, with no restrictions in scope based on taxonomy or tested PTEs. Based on this review, PC production varied based on sorbent taxonomic classification, generation of GSH compounds, and experimental physico-chemical conditions. Issues with reporting or opportunities for further research are also highlighted to support the advancement of this field.
Sorbent Differences
During PTE exposure, sorbent species or group may influence the magnitude of PC production with subsequent effects on PTE removal. PC production has been observed in several algal groups, including chlorophytes macroalgae, chlorophytes microalgae, diatoms, xanthophytes, dinoflagellates, and coccolithophores (). Although most algal sorbents identified in this review produce PCs, the magnitude of this production is not uniform between or within algal groups. Differences in the favoured detoxification mechanism under certain conditions may vary within or between groups resulting in differences in PC production. These inter- and intra-group differences can help to distinguish suitable algal groups and genera. Comparing PC production between algal sorbents, however, is restricted to studies that use the same system conditions and quantify PCs using equivalent units (Dong et al., Citation2020, Citation2020; Lavoie, Le Faucheur, Fortin, & Campbell, Citation2009). Working within these restrictions, the following sections cover inter- and intra-group differences in PC production in algal sorbents exposed to various PTEs.
Inter-group Differences
The production of PC may differ between algal groups despite similar system conditions. Two studies were identified which evaluate PC production across multiple algal groups under the same system conditions, Pistocchi, Mormile, Guerrini, Isani, & Boni (Citation2000) and Ahner, Wei, Oleson, & Ogura (Citation2002). Pistocchi, Mormile, Guerrini, Isani, & Boni (Citation2000) evaluated the effects of sorbent group and genus on PC formation during long-term Cd and Cu exposure. These researchers studied two diatoms, Cylindrotheca fusiformis and Achnanthes brevipes, and one bloom-forming dinoflagellate, Prorocentrum micans. Only C. fusiformis produced measurable levels of PC complexes in response to Cd, and these Cd-PC complexes were only observable between days 15 and 20 (Pistocchi, Mormile, Guerrini, Isani, & Boni, Citation2000). In this case, differences in PC production among the tested species likely occurred due to differences in the primary detoxification mechanism utilized. More specifically, only C. fusiformis utilized intracellular detoxification mechanisms in response to Cd stress, whereas A. brevipes excluded most of the ambient Cd in its extracellular matrix and P. micans released extracellular polysaccharides into the media. These alternate detoxification mechanisms used by A. brevipes and P. micans resulted in low PC production as well as a lack of Cd adsorption and accumulation (Pistocchi, Mormile, Guerrini, Isani, & Boni, Citation2000). Based on these results, it may seem that bloom-forming groups, or those that rely on granulation via the over-production of EPS, may not rely on intracellular detoxification mechanisms, producing no or very low levels of PCs (Naveed et al., Citation2019; Pistocchi, Mormile, Guerrini, Isani, & Boni, Citation2000). However, other bloom-forming dinoflagellate species, like Lingulodinium polyedrum have been observed to produce PCs at concentrations of Cd greater than 1.0 mg l−1 (Romano, Liria, Machini, Colepicolo, & Zambotti-Villela, Citation2017). It is therefore possible that the observed differences in PC production between algal groups in the Pistocchi, Mormile, Guerrini, Isani, & Boni (Citation2000) study relate to species-specific tolerance to tested PTE concentrations rather than differences in a sorbent group’s complete detoxification response. Differences in PC production related to PTE concentration are covered in more detail in the PTE and PTE Concentration section of this review.
Ahner, Wei, Oleson, & Ogura (Citation2002) also explored intergroup differences in the production of PCs in several microalgae groups including diatoms, Phaeodactylum tricornutum, Thalassiosira pseudonana, and Thalassiosira weissflogii, a coccolithophore, Emiliania huxleyi, and a chlorophyte, Dunaliella sp. In their study, PC production increased across almost all algal groups in response to Cu and Cd exposure, except for P. tricornutum cultures grown in the lowest tested concentration of Cd (0.3 mg Cd l−1 with EDTA; ). Their results indicated that the magnitude of PC production varied between algal groups. Namely, the diatoms T. pseudonana and T. weissflogii, and the coccolithophore produced very high levels of PCs following Cu and Cd exposure, while the tested chlorophyte produced relatively low levels of PCs (Ahner, Wei, Oleson, & Ogura, Citation2002). The coccolithophore also accumulated the highest levels of Cd and Cu when compared against the Thalassiosira diatoms; however, only these three cultures were analysed for PTE accumulation and not every concentration was analysed for PTE removal (Ahner, Wei, Oleson, & Ogura, Citation2002). There were, however, further issues with the data provided in this study due to sample size and unit selection. Firstly, only the Cd exposure trials for E. huxleyi were duplicated: the PC content reported in all other species and PTEs were based on one culture of each biosorbent; therefore, more trials are required to ensure reproducibility of the reported results. Similarly, though many of these results are reported based on the average internal volume of each cell, or cell biovolume, this study also quantified PC content in relation to chlorophyll a content for a set of experiments aimed at evaluating how culture age and sorbent group may relate to PC and GSH production. This unit might, however, not provide a reliable comparison for PC content between species, since pigment content may vary between species and/or can be affected by a species reaction to physico-chemical conditions, including presence and concentration of PTE (Zucchi & Necchi, Citation2001). For this reason, those culture age results have not been reported in .
Essentially, Pistocchi, Mormile, Guerrini, Isani, & Boni (Citation2000) and Ahner, Wei, Oleson, & Ogura (Citation2002) illustrate that inter-group differences in PTE tolerance and detoxification mechanism can affect PC production and subsequent PTE accumulation. Evidence of detoxification mechanism employed by an algal group should be considered during sorbent species selection. However, as will be covered in the following sections, even among groups that utilize intracellular detoxification mechanisms, further variation in PC production and PTE accumulation may occur on a species-specific basis.
Intra-group Differences
Although it may be assumed that algal sorbents within the same group would produce similar levels of PCs, several studies have identified intra-group differences in PC production despite similar experimental physico-chemical conditions. Ahner, Wei, Oleson, & Ogura (Citation2002) also evaluated these between diatoms. For example, at the same Cd concentration. T. pseudonana and T. weissflogii produced over 5 times the level of PCs as P. tricornutum (). Lavoie, Le Faucheur, Fortin, & Campbell (Citation2009) also illustrated intra-group differences through comparison of PC production in two chlorophyte microalgae, Chlamydomonas reinhardtii and Raphidocelis subcapitata, under increasing Cd exposure (0.079 × 10−3 − 0.028 mg Cd l−1). Following Cd exposure, both species’ PC content tripled at the highest Cd concentrations (0.025–0.028 mg Cd l−1; C. reinhardtii ≈700 amol SH cell−1 and R. subcapitata ≈ 900 amol SH cell−1). This evaluation, however, also suffers from reporting and unit selection issues. Specifically, though C. reinhardtii appeared to have higher PC content under control conditions when reported by cell count, PC levels were not significantly different between species when adjusted for cell biovolume (C. reinhardtii: ~350 µM SH; R. subcapitata ~270 µM SH). Unfortunately, these researchers do not report PC production following Cd exposure adjusted for cell biovolume. If this content was adjusted for cell biovolume, which the authors state is a factor of 1.3–2.5, it may emerge that R. subcapitata actually boasted a higher relative proportion of PCs as it is the smaller cell. This distinction in PC content is important because C. reinhardtii accumulated higher levels of Cd corrected for cell biovolume. The higher intracellular storage of Cd by C. reinhardtii in Lavoie, Le Faucheur, Fortin, and Campbell (Citation2009) evaluation can likely be attributed to Cd storage in other intracellular ligands. Other evaluations have also observed the storage of PTEs intracellularly via other ligands. For example, using elution through HPLC, Howe & Merchant (Citation1992) found that in C. reinhardtii 70% of intracellular Cd was stored by PCs: this would leave 30% of intracellular Cd stored by other intracellular ligands.
Dong, Wang et al., (Citation2020) and Dong, Zhu et al., (Citation2020) also compare the PC production in two diatom species under different levels of Cd exposure. These evaluations are focused on understanding Cd toxicity and tolerance and quantify PC content as a relative proportion of total proteins (Dong et al., Citation2020, Citation2020). In these evaluations, PC content increased with increasing Cd exposure in both diatom species, P. tricornutum and Skeletonema costatum (; 400 ppm CO2) (Dong Zhu, et al., Citation2020; Dong, Wang, et al., Citation2020); however, the magnitude of PC production varied between species. Namely, P. tricornutum produced higher levels of PCs at the 0.4 mg Cd l−1 treatment as compared with S. costatum, and S. costatum produced higher levels of PCs at the 1.2 mg Cd l−1 treatment (). These differences in PC production could potentially be correlated to a higher level of intracellular Cd storage achieved with higher PC content. Intracellular Cd storage was associated with higher PC production in S. costatum; however, intracellular Cd storage was not measured in P. tricornutum as this evaluation was principally concerned with defining Cd tolerance rather than biosorption capacity. Without information on intracellular Cd storage, it is not possible to define the relative contribution of PC production in the P. tricornutum cultures. These differences in data reporting in Dong, Zhu et al., (Citation2020); Dong, Wang et al., (Citation2020)), and Lavoie, Le Faucheur, Fortin, & Campbell (Citation2009) highlight the importance of evaluating other factors alongside PC production to distinguish the differences in sorbent performance. Specifically, though the production of PCs is valuable in terms of understanding the relative PTE tolerance and indicate storage location, complementing this information with an estimate of intracellular PTE content and the role of other intracellular or extracellular ligands provides a complete picture of the PTE removal location and recovery potential.
Finally, though it may be expected that species within the same genus would boast similar detoxification responses, variation in PC generation persists even at the genus and species-level. Genus-level variation emerged in the Ahner, Wei, Oleson, & Ogura (Citation2002) study where T. pseudonana generated nearly double the total PCs of T. weissflogii.at the 3.0 mg Cd l−1 treatment () (Ahner, Wei, Oleson, & Ogura, Citation2002). This has also been observed in a comparison of xanthophytes from the Vaucheria genus (Skowroński, De Knecht, Simons, & Verkleij, Citation1998). In this evaluation, Vaucheria compacta produced 3–6 times more PCs than Vaucheria debaryana under comparable system conditions and Cd levels (Skowroński, De Knecht, Simons, & Verkleij, Citation1998). Other evaluations have observed significantly different PC production in different strains, and even morphotypes, within a single species (Ma, Zhou, Chen, & Pan, Citation2021; Torricelli, Gorbi, Pawlik-Skowronska, Di Toppi, & Corradi, Citation2004). Sorbent strains may be altered through historic adaptation to high PTE conditions to improve PC production and PTE removal. For example, in a comparison of wild-type and Cr-tolerant strains of Scenedesmus acutus, Torricelli, Gorbi, Pawlik-Skowronska, Di Toppi, & Corradi (Citation2004) found that the Cr-tolerant S. acutus strain was able to achieve higher PC content during the highest level of Cd exposure (). This evaluation suggests sorbents can develop co-tolerances to certain PTEs. In terms of WWT and sorbent selection, these differences in PC production suggest that more robust species that are tolerant to a wide range of conditions and PTE concentrations may make highly effective bioaccumulators in PTE-containing WWs. Similarly, in an evaluation of morphotype effects on PTE tolerance in P. tricornutum, cell characteristics associated with the oval morphotype of the dinoflagellate led to a doubling of PC content as compared with the fusiform or sickle-shaped morphotypes (Ma, Zhou, Chen, & Pan, Citation2021).
As evidenced by the evaluations reported in this section, though a sorbent’s species or genus may provide fundamental information on the PTE tolerance and accumulation potential of that sorbent, many studies have identified a high-level of inter- and intra-group variation in PC production. This variation may lead to case-specific differences in PTE tolerance and removal. Evidence of these differences in PC production and PTE removal may support the exploitation of a locally available or low-cost sorbent species that come from genera previously discounted for low PTE removal.
The results from these evaluations also highlight the importance of reporting PC content adjusted by cell biovolume and reporting intracellular PTE content to understand the role of PCs in the overall detoxification response. For unit selection, units weighted by cell biovolume or dry weight offer the most reliable illustration of PC production, and standardization of these units will help with comparisons between evaluations. Furthermore, evaluating the presence of other intracellular compounds or typifying the magnitude of EPS production, can help to define the role of PCs in the sorbents detoxification response. Despite these inconsistencies, the outlined studies highlight the variability among live algal sorbents, and, subsequently, the wealth of further research opportunities.
Glutathione production
As it forms the building blocks for PC production, GSH is essential for algae’s ability to cope with PTE stress and store PTEs intracellularly through PC complexes (Kawakami, Gledhill, & Achterberg, Citation2006b) (). GSH is present in plants and algae, and mitigates oxidative and radiative damage, acting as a non-specific reductant during oxidative stress and PTE exposure (Kawakami, Gledhill, & Achterberg, Citation2006b; Rezayian, Niknam, & Ebrahimzadeh, Citation2019). The production dynamics of this thiol peptide can be complex as GSH serves several antioxidative functions in the cell (Howe & Merchant, Citation1992; Kawakami, Gledhill, & Achterberg, Citation2006b; Lavoie, Le Faucheur, Fortin, & Campbell, Citation2009). GSH may also be extruded to support PTE detoxification in an alga’s immediate environment (Kawakami, Gledhill, & Achterberg, Citation2006b; Laglera & Van Den Berg, Citation2003). GSH dynamics during PTE exposure, and how they affect PC synthesis, have been quantified in several laboratory studies.
GSH typically increases with PTE exposure, however, the length of PTE exposure may alter GSH production. Initially, over the first few hours of PTE exposure, GSH production may be excited by the presence of PTEs. This dynamic has been observed in several studies (Ahner, Wei, Oleson, & Ogura, Citation2002; Dong et al., Citation2020; Kawakami, Gledhill, & Achterberg, Citation2006a, Citation2006b). As PTE exposure continues over days in batch exposure experiments, GSH stores may drop, either in response to exhaustion of GSH stores, reduction of ambient PTEs, or transformation of GSH-PTE complexes to PC-PTE complexes. All of these factors may be the source of the overwhelmingly negative trends in GSH production observed in the Ahner, Wei, Oleson, & Ogura (Citation2002) study. In this evaluation, diatom, microalgae, and coccolithophore cultures were exposed to various concentrations of Cd or Cu in “long-term” exposure experiments (exposure period defined as “two generations”). In the majority of cultures, GSH content dropped between Day 0 and the final day of sampling. It is difficult to distinguish the source of this GSH production without more detailed sampling time, or analysis of other media constituents. Navarrete et al. (Citation2019) provide a more detailed assessment of GSH dynamics, cataloguing changes to GSH production in Ulva compressa over 12 days. In U. compressa, GSH content increases over the first 5 days of exposure in solutions containing 0.3 and 0.6 mg Cu l−1, but the GSH content then declined slightly at the day 12 measurement (Navarrete et al., Citation2019). In the 1.0 mg l−1 treatment, GSH reached its peak levels on day 5 and then declined below control content by day 12 (Navarrete et al., Citation2019). This decline is likely due to PC2 content, which reaches its peak concentration in this treatment by day 12. As these researchers supplemented Cu and nutrients every 48 h, it is likely that the drop in GSH content is related to sustained PC2 production rather than nutrient deficiency or reduction in the magnitude of the GSH response after an alleviation of Cu stress. Understanding the length of time resulting in exhaustion of GSH stores can help to optimize exposure time to peak PC production and PTE accumulation. From a WWT perspective, reducing exposure time to the minimum required period will be essential in designing the most efficient WWT systems.
GSH production and exhaustion may also be affected by present PTEs. Specifically, present PTEs may interact with GSH-related enzymes to inhibit or promote GSH production. Nagalakshmi and Prasad’s (Citation2001) evaluation of S. bijugatus, for example, explores the inhibitory dynamics in greater detail. In this evaluation, the activity of the GSSG-reductase (GSSG-R) is restricted by the interaction of Cu with an SH group on the enzyme (Devez, Achterberg, & Gledhill, Citation2015; Nagalakshmi & Prasad, Citation2001). This enzyme normally reduces oxidized GSH, GSSG, back to GSH (). This reduction is an essential recovery step following oxidative stress to regenerate available GSH pools (Anjum et al., Citation2012). At Cu concentrations of ~6.5 and 13 mg l−1 GSSG-R activity declined by 44 and 69%, respectively, as compared with Cu-free rates of activity (Nagalakshmi & Prasad, Citation2001). With GSSG-R partially incapacitated, GSH replenishment is restricted. Under these conditions, PC-PTE complexes are synthesized from existing PTE-loaded GSH without replenishment of GSH from GSSG, resulting in the exhaustion of GSH stores. Though Volland, Schaumlöffel, Dobritzsch, Krauss, & Lütz-Meindl (Citation2013) also tested the effect of Cu on GSH levels, their evaluation used a Cu concentration of 0.02 mg l−1. This comparatively low concentration may not achieve detectable inhibition of the GSSG-R enzyme; however, there is also evidence that beneficial enzyme-PTE interactions in algae and higher plants may also impact GSH production. In the Nagalakshmi and Prasad’s (Citation2001), the enzyme γ-glutamylcysteine (γ-GCS) increased in response to ~3.0 and 6.0 mg Cu l−1 exposure by 6 to 18%, respectively. γ-GCS is generally the limiting enzyme in GSH production, as it is upregulated by the absence of GSH; however, in the case of S. bijugatus, GSSG-R is the limiting enzyme to GSH production as it is inhibited to a far greater extent than γ-GCS is upregulated (Nagalakshmi & Prasad, Citation2001; Noctor et al., Citation1995). Other PTEs have improved γ-GCS performance in plant systems. Cd, for example, has been observed to have a beneficial effect on the enzyme γ-GCS in plant cells, promoting GSH production (Anjum et al., Citation2012; Nagalakshmi & Prasad, Citation2001; Rüegsegger & Brunold, Citation1992; Schäfer, Haag-Kerwer, & Rausch, Citation1998). Despite a thorough literature review, upregulation of γ-GCS by Cd was not identified in this review, and there was no evidence of this effect in any of the PTE-algae systems covered in this work. Lavoie, Le Faucheur, Fortin, & Campbell (Citation2009), for example, did not identify an increase in intracellular GSH in studied microalgae species following Cd exposure (C. reinhardtii and R. subcapitata) (Lavoie, Le Faucheur, Fortin, & Campbell, Citation2009). This could be due to the concentration of Cd in this study, which was only introduced in the range of 0.078 × 10−3 to 0.03 mg l−1 or could be related to differences between algal and plant cells. Therefore, enzymes integral to GSH production may be affected by PTE exposure, having downstream consequences on GSH stores and PC production. Furthermore, the inconsistencies in enzyme behaviour between algae-PTE systems illustrate the high-level of variability between algal sorbents, and the myriad opportunities for further research in the field
In terms of WWT, adequate GSH levels are vital to the synthesis of PC-PTE complexes, intracellular PTE tolerance, and, ultimately, a culture’s ability to survive PTE exposure and accumulate PTEs (Piña-Olavide et al., Citation2020; Torricelli, Gorbi, Pawlik-Skowronska, Di Toppi, & Corradi, Citation2004). Therefore, if GSH content or production drops significantly, GSH may not be available for PC synthesis, halting proper PTE accumulation. Factors affecting GSH production, like exposure time and the interaction of present PTEs, should be considered during live algal sorbent system design.
Physico-chemical conditions
Due to the metabolic requirements of live sorbents, PC production may be impacted by physico-chemical conditions including pH, temperature, light intensity (LI), and CO2 enrichment. Beginning with pH, pH is often considered the key physio-chemical condition restricting the use of live algal sorbents for WWT. The reason for this restriction is the incongruence between the pH at which PTE availability is generally optimized (pH 3–5), and the pH at which aquatic and marine alga generally thrive (pH 6.5–8). Although pH-related mobility and availability vary between PTEs, the optimal pH for a PTE will be a range at which the PTE exists in solution rather than precipitating or volatilizing out of solution (Wood, Citation1985). For this reason, pH may indirectly affect PC production in multi-PTE solutions by affecting the bioavailability of present PTEs. Pawlik-Skowron (Citation2001), for example, evaluated PC dynamics in Stigeoclonium mats in response to synthetic mine drainage containing multiple PTEs. In this study, wild-harvested Stigeoclonium mats were cleaned and exposed to synthetic mine drainage containing µM concentrations of Zn, Cu, Cd, Pb, Ca, Mg, and Fe (Pawlik-Skowrońska & Bačkor, Citation2011). Depending on the solution pH and resulting bioavailability of the present PTEs, PC production increased by up to ~2.5–2.8 fold (Pawlik-Skowron, Citation2001). The researchers suggest that a ~ 4× increase in the labile proportion of Zn in the pH 6.8 mine drainage led to the steep increase in PC production as compared with Stigeoclonium grown in the pH 8.2 synthetic mine drainage. pH may also directly affect algal sorbents by impacting individual survival (Hansen, Citation2002), growth rate (Chen & Durbin, Citation1994; Hansen, Citation2002), biochemical profile (Khalil, Asker, El-Sayed, & Kobbia, Citation2010; Moheimani, Citation2013), photosynthetic rate (Qiu, Gao, Lopez, & Ogden, Citation2017), protonation of negatively charged surface structures (Holan & Volesky, Citation2014; Tüzün et al., Citation2005), and CO2 uptake (Chen & Durbin, Citation1994). However, there were no evaluations identified in this review comparing metabolic changes.
Similar to pH, temperature may indirectly affect PC generation through changes to culture productivity, or directly impact it by effecting enzymatic activity necessary for its synthesis (Hedayatkhah et al., Citation2018; Lambert et al., Citation2016; Wang & Wang, Citation2008). Impact of temperature on subcellular PC pools has also been investigated in benthic macroalgae and diatom species (Skowroński, De Knecht, Simons, & Verkleij, Citation1998). Skowroński, De Knecht, Simons, and Verkleij (Citation1998) explored changes to Cd accumulation in Vaucheria compacta and V. debaryana. In this evaluation, Cd accumulation correlated significantly with temperature, Cd concentration, and exposure time in the studied species. For V. compacta, increasing the temperature from 8°C to 16°C led to a doubling of PC2 and PC3, and measurable production of PC4 (33; ). By changing the temperature from 4°C to 18°C, V. debaryana exhibited a smaller increase of PC production in PC2 at 31%, but PC3 content increased by 56%. These temperature-dependent changes to PC production also resulted in increases to the Cd content. For example, for V. debaryana, when temperature increased from 4°C to 18°C, Cd accumulation increased from 17.6 ± 2.2 to 24.3 ± 3.4 µmol Cd g−1 DW. A similar result was obtained for V. compacta, where increasing the temperature from 8°C to 16°C, led to ~40% increase in Cd accumulation (12.9 ± 1.5 to 20.3 ± 3.4 µmol SH g−1 DW). At lower temperatures, reduced efficiency of PC production and Cd accumulation may be attributed to reduced Cd ion transport into the cell and diminishing functionality of the temperature-dependent PC synthase enzyme, which has an optimal temperature of 35°C (Skowroński, De Knecht, Simons, & Verkleij, Citation1998). Wang and Wang (Citation2008) also found that increasing the temperature from 18° to 24°C increased subcellular PC pools, resulting in higher intracellular Cd accumulation in the diatom Thalassiosira nordenskioeldii, increasing from 523 ± 14.5 to 718 ± 18.6 µmol Cd mol C−1, respectively. These authors connect the temperature-dependent Cd accumulation to changes in PCs as well as other thiol-containing compounds; however, there was a limit to the beneficial effects of temperature. Though the Vaucheria sp. study suggests that PC synthase enzyme is optimal at 35°C, Wang and Wang (Citation2008) observed a drastic drop in intracellular Cd, 211 ± 2.34 µmol Cd mol C−1, when T. nordenskioeldii was grown at 30.5°C, accompanied by a reduction in the magnitude of PC pools as compared with the cultures grown in similar levels of Cu exposure at 18°C and 24°C. However, the magnitude of this decline in Cd accumulation and detoxification can only partially be explained by reduced PC production, as temperature changes may also affect other aspects of the detoxification process, including GSH, resulting in higher Cd sensitivity (Wang & Wang, Citation2008).
In industrial algae production, LI is most often considered for its effect on biomass production (Peckol et al., Citation1994) or fatty acid synthesis (Pinto et al., Citation2011). Similar to other physio-chemical conditions, algal sorbents may require different optimal LI, which depends on the culture density and algae species. Culture density had a parabolic relationship to LI. In densely packed cultures, light can be attenuated quickly, leading to uneven LI and reductions in growth rate (Wahal & Viamajala, Citation2010). On the other hand, low density cultures can be overexposed to light, leading to the inhibition of photosynthesis (Wahal & Viamajala, Citation2010). Only one evaluation assessed the impact of LI on PC generation using two macroalgae species from the Vaucheria genus, V. compacta and V. debaryana. In this study, though PCs were produced in response to Cd in both light and dark conditions, PC pools in the dark conditions were 50–60% lower than those observed in the light sufficient conditions (Skowroński, De Knecht, Simons, & Verkleij, Citation1998). This reduced generation resulted in Cd accumulation falling by ~47% and 43% in V. compacta and V. debaryana, respectively. These trends in PC content and Cd accumulation were most likely related to photosynthetic activity and available energy. With reduced photosynthetic activity, there may be lower levels of energy available for cellular “work”, and high-energy detoxification methods like PC production may be restricted. Alterations to photosynthetic capacity relating to high irradiance may also impact PC production and PTE accumulation. For example, in Sarcodia suiae, increasing LI negatively impacted the accumulation of As, due to damage to photosynthetic organs, resulting in depigmentation (Libatique, Lee, & Yeh, Citation2019). Although this evaluation does not measure PC pools, it is possible that a reduction in photosynthetic activity restricted available energy for PC generation.
Finally, energy availability may also modulate PC pools in CO2 enriched environments, however, not in the same manner that occurs based on temperature and LI. Dong, Wang, et al. (Citation2020) and Dong, Zhu, et al. (Citation2020) tested PC production in response to Cd exposure marine diatoms, Skeletonema costatum and P. tricornutum, respectively, at normal CO2 content (400 ppm CO2) and CO2 content typical of ocean acidification (1500 ppm CO2). Both studies identified a reduced generation of PCs as a result of CO2 enrichment, but despite this reduction, toxicity related to Cd exposure was mitigated, allowing for higher growth and chlorophyll a content over long-term exposure. For example, under CO2 enrichment, P. tricornutum cultures grown in 0.4 and 1.2 mg l−1 of Cd reached densities 160.69% and 187.59%, respectively, greater than the control cultures at 0 mg Cd l−1 and 400 ppm CO2 (Dong, Wang, et al. (Citation2020) and Dong, Zhu, et al. (Citation2020). The authors traced this mitigated toxicity to a shift in the principle ligand responsible for intracellular detoxification from PCs to GSH (Dong et al., 2020a, 2020b). In order to conserve energy under the combined stress of CO2 enrichment and Cd exposure, it seems that both marine diatoms favoured production of the multifunctional GSH molecules to combat oxidative damage, rather than investing in costly and PTE-specific PCs (Dong, Wang, et al. Citation2020; Dong, Zhu, et al. Citation2020; Kawakami, Gledhill, & Achterberg, Citation2006b). Despite the switch in ocean acidification conditions, S. costatum cultures’ intracellular Cd accumulation significantly increased from ~2.2 to ~3.2 mg Cd kg−1 DW and ~2.4 to ~4.1 mg Cd kg−1 DW in the 0.4 and 1.2 mg Cd l−1 treatments, respectively (Dong, Wang, et al., Citation2020). In terms of wastewater treatment, this increased intracellular PTE tolerance under CO2 enrichment could support the inclusion of flue gas into certain PTE remediation systems, though not through the increased generation of PC pools (Onyancha, Lubbe, & Brink, Citation2021).
Essentially, physico-chemical conditions can alter the PC pools either through contribution to cellular stress or alterations to energy available for the energy-intensive detoxification mechanism. In active sorbents, like live algae, metabolic requirements must be met in order to maintain cell productivity, support a sufficient detoxification response, and ensure PTE accumulation. Identifying the optimal system conditions for each sorbent to maximize PC production and PTE accumulation is essential to ensure the viability of these WWT tools; however, observing shifts in the predominant detoxification response will also help to understand if shifting conditions simply alter the favoured detoxification route without negatively impacting PTE removal.
PTE and PTE concentration
Since PC production plays a dominant role in detoxification and subsequent tolerance to PTE pollution, PCs predominantly have a positive relationship to PTE exposure and increasing PTE concentrations. In terms of present PTEs, PC production has been linked to Cd, Cu, Cr, Zn, Pb, and Hg exposure (). However, the occurrence and magnitude of PC production is not uniform in response to these PTEs. For instance, Ahner & Morel (Citation1995b) found that PC production by T. weissflogii was highly dependent on present PTE. In this study, T. weissflogii, was grown in the presence of Cd, Pb, Ni, Cu, Zn, Co, Ag, and Hg. Cd, Zn, and Cu, to a lesser extent, excited PC formation in short-term exposure experiments; however, these results were reported in nmol PCs per chlorophyll a content (Ahner & Morel, Citation1995b). As outlined previously, this unit is not reliable due to the changeable nature of chlorophyll a. Subsequently, though PCs were generated in response to these PTEs, the relative magnitude of PC production between PTEs cannot be determined. Volland, Schaumlöffel, Dobritzsch, Krauss, and Lütz-Meindl (Citation2013) also evaluated the varying effects of single-PTE solutions on PC production. In short-term batch exposures to Cd, Cr, and Cu, Micrasterias denticulata produced PCs in response to Cd and Cr, but not Cu (Volland, Schaumlöffel, Dobritzsch, Krauss, & Lütz-Meindl, Citation2013). The lack of PC production during PTE exposure may indicate an alternate detoxification method for Cu, e.g., exclusion in the EPS or alternate intracellular ligands in this particular sorbent (Pawlik-Skowrońska, Citation2003). These evaluations illustrate the complex variation in live algal sorbent performance in PTE remediation and highlight the high-level of optimization available through sorbent selection.
Most evaluations in this review, however, define PC excitation following exposure to single-PTE solutions without evaluating the effects of mixed-PTE solutions. In mixed-PTE solutions, PTEs may interact at binding sites, working in coordination or competition, altering the magnitude of PC production through changes to intracellular PTE transport. Understanding the dynamics of multi-PTE solutions is therefore essential for proper WWT, as multiple PTEs may be present in WW effluent. Mixed-PTE solutions have been evaluated in PTE removal more generally, but this review did not find evidence of a comprehensive analysis of PC production in mixed-PTE solutions, except for Pawlik-Skowron’s (Citation2001) evaluation of Stigeoclonium mats. As previously mentioned in the Physico-Chemical Conditions section, PC production was chiefly affected by changes in Zn concentration in the mixed PTE solution. In this same evaluation, the researchers also exposed Stigeoclonium mats to 10 µM of single-PTE solutions of Cd, Pb, and Zn. Although pH changes in the mixed-PTE solutions identified Zn as the major determinant of PC production in the mixed-PTE solution, in single-PTE solutions, Cd produced the highest total PC response, followed by Pb and then Zn. These differences in PC production in single- versus mixed-PTE solutions illustrate the value of testing both exposure mediums to determine changes to sorbent performance. Although this was performed in the Pawlik-Skowron’s (Citation2001) study, in the wider literature there is a lack of information on the changes to PC synthesis and PTE storage in response to mixed-PTE solutions. Understanding the relative contribution of PTEs to PC production, in single- and mixed-PTE solutions is essential for defining the sorbent’s suitability to WW effluents where PTE content and concentration may be varied and episodic.
In terms of PTE concentration, several studies identified changes to PC production based on PTE concentration (Ahner, Wei, Oleson, & Ogura, Citation2002; Dong Wang, et al., Citation2020; Dong, Zhu, et al., Citation2020; Navarrete et al., Citation2019; Skowroński, De Knecht, Simons, & Verkleij, Citation1998; Torricelli, Gorbi, Pawlik-Skowronska, Di Toppi, & Corradi, Citation2004). All of these evaluations observe an increase in PC production at higher PTE concentrations (). Ahner & Morel (Citation1995a) also found that PC levels declined as PTE concentration decreased in the media over the exposure period (Ahner & Morel, Citation1995a). This study indicated that PC production was highly reactive to PTE stress as well as the alleviation of that stress. Wang and Wang (Citation2011) also explored this principal further, evaluating how PC production reacted to periods of recovery following PTE stress. In this study, T. nordenskioeldii PC production was dependent on ambient Cd concentration, quickly responding to increased Cd concentration as well as to the reduction of it. In this evaluation, following 24 h of recovery from Cd exposure, T. nordenskioeldii produced higher levels of PC2 in response to another round of high Cd exposure (0.944×10−5 mg l−1) (Wang & Wang, Citation2011). Changes to PTE removal following short-periods of recovery have also been observed in Microcystis aeruginosa exposed to Cd and Zn (Zeng, Yang, & Wang, Citation2009). In this evaluation, the accumulation increased following a 24 hr recovery from PTE exposure; however, the researchers do not measure PC production and so the nature and magnitude ligand’s role in this change is unclear. From a WWT perspective, elevated accumulation following a period of recovery could improve sorbent performance in WWs where PTE exposure is episodic.
Wang & Wang (Citation2011), however, also found that when ambient Cd concentration is reduced, PCs may be quickly extruded resulting in a decline in intracellular Cd (Ahner & Morel, Citation1995a; Wang & Wang, Citation2011). This response resulted in Cd returning to the ambient environment instead of being locked in sorbent cells. Although this presents issues in WW streams with intermittent PTE pollution, it also presents an opportunity for non-destructive PTE recovery. For example, after WWT, PTE-loaded algal sorbents could be transferred to a much smaller volume of clean media, allowing for the controlled release of accumulated PTEs. This method of recovery is an area of emerging research in macro- and microalgae with studies predominately focusing on the release of PTE-loaded PCs to indicate individual and culture resilience rather than as a PTE recovery method (Pesce, Ghiglione, & Martin-Laurent, Citation2017). Alternatively, there is evidence that, in certain sorbents, PCs are stable over short-term recovery periods. Navarrete et al. (Citation2019) evaluation of U. compressa found that although accumulated PTEs were extruded following a period of Cu-free recovery, they also identified that PCs were not released into the PTE-free seawater. Instead, PTEs were extruded via GSH during the recovery period (Navarrete et al., Citation2019). This lack of exudation of PC complexes shows a route for stable PTE retention, potentially cushioning PTE loss during episodic PTE exposure. The mismatch in PC exudation in T. nordenskioeldii and U. compressa also illustrates the level of variability between live algal sorbents.
In terms of the effects of PTE and PTE concentration on PC production, future research should consider more complex PTE solutions like those containing a mixture of PTEs or other potentially competitive ions. PTE interactions have been explored in higher plant systems and indicate that combined exposure can increase negative consequences to the exposed sorbent. For example, in Brassica napus exposed to Cr and Cu, combined exposure enhanced oxidative damage, reduced pigment content and impacted plant quality (Li et al., Citation2018). Although trends observed in higher plants may not directly translate to algal systems, it does provide information on the behaviour of PTEs in related detoxification systems. The role of mixed-PTE solutions on PC production across multiple species were not identified in this exhaustive literature review. On the other hand, nutrient concentrations may shift temporally and spatially in WWs, and these shifts may affect PC production and, ultimately, PTE removal. There are studies that have evaluated the effects of nutrient conditions on PTE import without defining changes to PC production (Hedayatkhah et al., Citation2018; Neumann, De Souza, Pickering, & Terry, Citation2003; Taboada-de la Calzada, Villa-Lojo, Beceiro-González, Alonso-Rodrı́guez, & Prada-Rodrı́guez, Citation1998). For example, Taboada-de la Calzada et al. (Citation1998) found evidence that toxic AsO33– may share a common uptake route with NO3– and PO43– in C. vulgaris. Under nutrient depleted conditions, C. vulgaris accumulated 12% more AsO33– than under nutrient sufficient conditions (Taboada-de la Calzada, Villa-Lojo, Beceiro-González, Alonso-Rodrı́guez, & Prada-Rodrı́guez, Citation1998). The researchers suggest that the toxic metalloid species has a similar chemistry to these nutrients suggesting that AsO33– may exploit the same uptake route as NO3– and PO43–. This dynamic has also been observed in Se systems, where selenate (SeO42−) shares the sulphate (SO42−) uptake route in freshwater Chlorella sp. (Neumann, De Souza, Pickering, & Terry, Citation2003). Neumann, De Souza, Pickering, & Terry (Citation2003) identified that under nutrient deprivation 90% of the 20 mM of provided selenate was accumulated by the Chlorella biomass; however, when supplemented with 1 mM of sulphate, the remediation efficiency dropped to 1.8% (Neumann, De Souza, Pickering, & Terry, Citation2003). Although there were no studies in this review that directly analysed the effects of nutrient deprivation on PTE transport and PC production, it would be necessary to define these interactions before field-scale testing of many of the algal sorbents identified in this review.
Overall, there were several examples of increased PC synthesis in response to Cd, Cu, Zn, Pb, and Hg, and some algae-PTE systems showed evidence of highly responsive and dynamic PC production in response to changes in PTE exposure. Though many systems showed that PC production changed with PTE exposure, the magnitude of PC change was not standardized in all algae-PTE systems or between PTEs. This inconsistency shows the importance of assessing multiple live sorbents and PTEs under the same system conditions to understand the comparative effects. Similar to other sections, units need to be standardized and consider cell bio-volume and potential changes to water content following PTE exposure.
Exposure time
PC production had a positive relationship to exposure time. This trend has been observed over long-term exposure to PTEs in C. fusiformis and U. compressa (Navarrete et al., Citation2019; Pistocchi, Mormile, Guerrini, Isani, & Boni, Citation2000). In Pistocchi, Mormile, Guerrini, Isani, & Boni (Citation2000) study, C. fusiformis produced PCs in response to Cd exposure, and the level of production was positively correlated to exposure time. In this evaluation during exposure to 0.5 mg Cd l−1, Cd accumulation by PCs increased from 79% to 90% between days 15 and 21. This rise suggests that PC pools would have increased between day 15 to 21 to accommodate the higher percentage of PC-bound Cd (, 16). Navarrete et al. (Citation2019) also found increasing PC production in semi-batch exposure experiments, providing a more detailed account PC content. In this evaluation, researchers observed the complementary detoxification response of GSH, PCs, and class-II metallothioneins in the marine seaweed. Navarrete et al. (Citation2019) also identified that the production of different PC oligomers was affected by time in U. compressa’s Cu detoxification response. In terms of PC production, over a 12-day exposure period, PC dimers (PC2) and tetramers (PC4) were consistently produced at a high level in the 0.843 mg Cu l−1 treatment. There was also a slower increase in PC dimers in 0.3, 0.6, and 1.0 mg Cu l−1. Peak PC2 content occurred on the final sampling day in the 1.0 mg Cu l−1. Production of PC4 occurred to a much lower extent, peaking at ~80 nmol G−1 FW on day 1 in the 0.843 mg Cu l−1 treatment, and after 12 days of exposure the highest PC4 content was in the 1.124 mg Cu l−1 treatment (~60 nmol g−1 FW), again to a much lower extent than PC2. This increase in longer PC polymers over long-term exposure at the highest Cd concentration may be specific to the U. compressa – Cu system, but it suggests that exposure time may improve the stability of PC-PTE complexes as longer oligomers are synthesized over time in the higher PTE treatments (Kneer & Zenk, Citation1997; Lavoie, Le Faucheur, Fortin, & Campbell, Citation2009; Navarrete et al., Citation2019). This response, however, was dependent on the concentration of PTEs.
These evaluations into the effects of continued PTE exposure on PC production provide interesting insights into PTE storage location and stability over long-term exposure. As there is evidence PC production may continually increase with PTE exposure time, exposure time may be modulated to control PTE storage location. For example, if PTEs must be easily recovered through non-destructive processes, such as desorption, shorter exposure times may prevent high levels of intracellular PTE accumulation, or a sorbent which predominately uses exclusion as the main detoxification response could be selected instead. On the other hand, long-term exposure with a PC producing algal sorbent could improve the stability of PC-PTE complexes as longer chain oligomers are synthesized. Although it is not clear if these trends would be mirrored in other systems,
In conclusion, overall, sorbent species, present PTEs, PTE quantity, exposure time, and changes to physico-chemical conditions may affect PC synthesis and, subsequently, PTE removal. Changes in PC production as a result of these factors highlight the range of opportunities for system optimization to maximize PTE removal through increased PC production by different algal species.
With the ligand only being identified in higher plants in the 1980s, however, this area of research is still relatively new and there is a range of opportunities for standardization of experimental methods and further research (Singh & Schwan, Citation2019). In terms of standardizations, unit choice was one of the key restricting factors to comparing PC production in different sorbents. At the moment, units relating PC content to SH content per cell, pigmentation, or protein content leave room for confounding results. Even Navarrete et al. (Citation2019) use of fresh weight in the PC content unit for U. compressa could be skewed by osmotic responses to PTE toxicity (Sánchez-Thomas et al., Citation2020). Adjusting PC content for cell biovolume, as in Lavoie, Le Faucheur, Fortin, & Campbell (Citation2009), or dry weight may help to reduce confounding factors affecting comparison of live algal sorbents.
Ultimately, in the field of bioaccumulation, there are a wealth of opportunities for optimization and further research, particularly as it pertains to understanding and optimizing the role PCs play in live algal biosorbents. Specifically, defining the impact of system conditions on PC production, will help to vet the efficacy of algal biosorbents for certain WW streams and define routes for PTE recovery.
Abbreviations
EPS | = | Extracellular Polymeric Substances |
GSH | = | Glutathione |
LI | = | Light Intensity |
PC | = | Phytochelatins |
PTE | = | Potentially Toxic Element |
RE | = | Removal Efficiency |
SD | = | Standard Deviation |
SH | = | sulphhydryl |
WW | = | Wastewater |
WWT | = | Wastewater Treatment |
Author Contributions
S.K.R., M.E.R., A.J.C.S., and M.C.G. conceived and planned the project. S.K.R. reviewed the literature. S.K.R. wrote the manuscript. S.K.R., M.E.R., A.J.C.S., and M.C.G. reviewed and edited the manuscript. M.E.R., A.J.C.S., and M.C.G supervised the project. S.K.R. acquired the funding.
Acknowledgements
We would like to acknowledge the support of the British Phycological Society through their Covid-19 Recovery Award.
Disclosure statement
No potential conflict of interest was reported by the authors.
Additional information
Funding
References
- Ahluwalia, S. S., & Goyal, D. (2007). Microbial and plant derived biomass for removal of heavy metals from wastewater. Bioresource Technology, 98, 2243–2257. doi:10.1016/j.biortech.2005.12.006
- Ahner, B. A., & Morel, F. M. M. (1995a). Phytochelatin production in marine algae. 1. An Interspecies comparision. Limnology and Oceanography, 40, 658–665. doi:10.4319/lo.1995.40.4.0658
- Ahner, B. A., & Morel, F. M. M. (1995b). Phytochelatin production in marine algae. 2. Induction by various metals. Limnology and Oceanography, 40, 658–665. doi:10.4319/lo.1995.40.4.0658
- Ahner, B. A., Wei, L., Oleson, J. R., & Ogura, N. (2002). Glutathione and other low molecular weight thiols in marine phytoplankton under metal stress. Marine Ecology Progress Series, 232, 93–103. doi:10.3354/meps232093
- Anjum, N. A., Ahmad, I., Mohmood, I., Pacheco, M., Duarte, A. C., Pereira, E. … Prasad, M. N. V. (2012). Modulation of glutathione and its related enzymes in plants’ responses to toxic metals and metalloids-a review. Environmental and Experimental Botany, 75, 307–324. doi:10.1016/j.envexpbot.2011.07.002
- Boran, M., & Altinok, I. (2010). A review of heavy metals in water, sediment and living organisms in the black sea. Turkish Journal of Fisheries and Aquatic Sciences, 10, 565–572. doi:10.4194/trjfas.2010.0418
- Chen, C. Y., & Durbin, E. G. (1994). Effects of pH on the growth and carbon uptake of marine phytoplankton. Marine Ecology Progress Series, 109, 83–94. doi:10.3354/meps109083
- Chibuike, G. U., & Obiora, S. C. (2014). Heavy metal polluted soils: effect on plants and bioremediation methods. Applied and Environmental Soil Science, 1–12. doi:https://doi.org/10.1155/2014/752708
- Cobbett, C. (2002). Phytochelatins and their roles in heavy metal detoxification. Plant Physiology, 123, 825–832. doi:10.1104/pp.123.3.825
- Cobbett, C., & Goldsbrough, P. (2002). Phytochelatins and metallothioniens: roles in heavy metal detoxification and homeostasis. Annual Review of Plant Biology, 53, 159–182. doi:10.1146/annurev.arplant.53.100301.135154
- Croot, P. L., Moffett, J. W., & Brand, L. E. (2000). Production of extracellular Cu complexing ligands by eucaryotic phytoplankton in response to Cu stress. Limnology and Oceanography, 45, 619–627. doi:10.4319/lo.2000.45.3.0619
- Danouche, M., El Ghachtouli, N., El Baouchi, A., & El Arroussi, H. (2020). Heavy metals phycoremediation using tolerant green microalgae: enzymatic and non-enzymatic antioxidant systems for the management of oxidative stress. Journal of Environmental Chemical Engineering, 8, 104460. doi:10.1016/j.jece.2020.104460
- Devez, A., Achterberg, E., & Gledhill, M. (2015). 15 metal ion-binding properties of phytochelatins and related ligands. In Metallothioneins and Related Chelators. doi:10.1515/9783110436273-020
- Dong, F., Wang, P., Qian, W., Tang, X., Zhu, X., Wang, Z. … Wang, J. (2020). Mitigation effects of CO2-driven ocean acidification on Cd toxicity to the marine diatom skeletonema costatum. Environmental Pollution, 259, 113850. doi:10.1016/j.envpol.2019.113850
- Dong, F., Zhu, X., Qian, W., Wang, P., & Wang, J. (2020). Combined effects of CO2-driven ocean acidification and cd stress in the marine environment: enhanced tolerance of phaeodactylum tricornutum to cd exposure. Marine Pollution Bulletin, 150, 110594. doi:https://doi.org/10.1016/j.marpolbul.2019.110594
- Foster, P. L. (1982). Species associations and metal contents of algae from rivers polluted by heavy metals. Freshwater Biology, 12, 17–39. doi:10.1111/j.1365-2427.1982.tb00601.x
- Friedlova, M. (2010). The influence of heavy metals on soil biological and chemical properties. Soil and Water Research, 5, 21–27. doi:10.17221/11/2009-swr
- Gaudry, A., Zeroual, S., Gaie-Levrel, F., Moskura, M., Boujrhal, F. -Z., El Moursli, R. C. , andDelmas, R. (2007). Heavy metals pollution of the Atlantic marine environment by the Moroccan phosphate industry, as observed through their bioaccumulation in Ulva lactuca. Water, Air, and Soil Pollution, 178, 267–285. doi:10.1007/s11270-006-9196-9
- Gekeler, W., Grill, E., Winnacker, E. L., & Zenk, M. H. (1988). Algae sequester heavy metals via synthesis of phytochelatin complexes. Archives of Microbiology, 150, 197–202. doi:10.1007/BF00425162
- Gómez-Jacinto, V., García-Barrera, T., Gómez-Ariza, J. L., Garbayo-Nores, I., & Vílchez-Lobato, C. (2015). Elucidation of the defence mechanism in microalgae Chlorella sorokiniana under mercury exposure. identification of Hg–phytochelatins. Chemico-Biological Interactions, 238, 82–90. doi:10.1016/j.cbi.2015.06.013
- Hansen, P. J. (2002). Effect of high pH on the growth and survival of marine phytoplankton: Implications for species succession. Aquatic Microbial Ecology, 28, 279–288. doi:10.3354/ame028279
- He, J., & Chen, J. P. (2014). A comprehensive review on biosorption of heavy metals by algal biomass: Materials, performances, chemistry, and modeling simulation tools. Bioresource Technology, 160, 67–78. doi:10.1016/j.biortech.2014.01.068
- Hedayatkhah, A., Cretoiu, M. S., Emtiazi, G., Stal, L. J., Bolhuis, H., Hedayatkhah, A. … Bolhuis, H. (2018). Bioremediation of chromium contaminated water by diatoms with concomitant lipid accumulation for biofuel production. Journal of Environmental Management, 227, 313–320. doi:10.1016/j.jenvman.2018.09.011
- Holan, Z. R., & Volesky, B. (2014). Biosorption of heavy metals: Review. Journal of Chemical Science and Technology, 3, 74–102.
- Howe, G., & Merchant, S. (1992). Heavy metal-activated synthesis of peptides in Chlamydomonas reinhardtii. Plant Physiology, 98, 127–136. doi:10.1104/pp.98.1.127
- Islam, M. S., Ahmed, M. K., Raknuzzaman, M., Habibullah -Al- Mamun, M., & Islam, M. K. (2015). Heavy metal pollution in surface water and sediment: A preliminary assessment of an urban river in a developing country. Ecological Indicators, 48, 282–291. doi:10.1016/j.ecolind.2014.08.016
- Kawakami, S. K., Gledhill, M., & Achterberg, E. P. (2006a). Effects of metal combinations on the production of phytochelatins and glutathione by the marine diatom Phaeodactylum tricornutum. BioMetals, 19, 51–60. doi:10.1007/s10534-005-5115-6
- Kawakami, S. K., Gledhill, M., & Achterberg, E. P. (2006b). Production of phytochelatins and glutathione by marine phytoplankton in response to metal stress. Journal of Phycology, 42, 975–989. doi:10.1111/j.1529-8817.2006.00265.x
- Khalil, Z. I., Asker, M. M. S., El-Sayed, S., & Kobbia, I. A. (2010). Effect of pH on growth and biochemical responses of Dunaliella bardawil and Chlorella ellipsoidea. World Journal of Microbiology & Biotechnology, 26, 1225–1231. doi:10.1007/s11274-009-0292-z
- Kneer, R., & Zenk, M. H. (1997). The formation of cd-phytochelatin complexes in plant cell cultures. Phytochemistry, 44, 69–74. doi:10.1016/S0031-9422(96)00514-6
- Laglera, L. M., & Van Den Berg, C. M. G. (2003). Copper complexation by thiol compounds in estuarine waters. Marine Chemistry, 82, 71–89. doi:10.1016/S0304-4203(03)00053-7
- Lambert, A. S., Dabrin, A., Morin, S., Gahou, J., Foulquier, A., Coquery, M., & Pesce, S. (2016). Temperature modulates phototrophic periphyton response to chronic copper exposure. Environmental Pollution, 208, 821–829. doi:10.1016/j.envpol.2015.11.004
- Lata, S., Singh, P. K., & Samadder, S. R. (2015). Regeneration of adsorbents and recovery of heavy metals: A review. International Journal of Environmental Science and Technology, 12, 1461–1478. doi:10.1007/s13762-014-0714-9
- Lavoie, M., Le Faucheur, S., Fortin, C., & Campbell, P. G. C. (2009). Cadmium detoxification strategies in two phytoplankton species: Metal binding by newly synthesized thiolated peptides and metal sequestration in granules. Aquatic Toxicology, 92, 65–75. doi:10.1016/j.aquatox.2008.12.007
- Libatique, M. J. H., Lee, M. –. C., & Yeh, H. –. Y. (2019). Effect of light intensity on the mechanism of inorganic arsenic accumulation and patterns in the red macroalga, Sarcodia suiae. Biological Trace Element Research, 195, 291–300. doi:10.1007/s12011-019-01833-0
- Lin, Z., Li, J., Luan, Y., & Dai, W. (2020). Application of algae for heavy metal adsorption: A 20-year meta-analysis. Ecotoxicology and Environmental Safety, 190, 110089. doi:10.1016/j.ecoenv.2019.110089
- Li, L., Zhang, K., Gill, R. A., Islam, F., Farooq, M. A., Wang, J., & Zhou, W. (2018). Ecotoxicological and Interactive Effects of Copper and Chromium on Physiochemical, Ultrastructural, and Molecular Profiling in Brassica napus L. BioMed research international. doi:10.1155/2018/9248123
- Luo, H., Wang, Q., Liu, Z., Wang, S., Long, A., & Yang, Y. (2020). Potential bioremediation effects of seaweed Gracilaria lemaneiformis on heavy metals in coastal sediment from a typical mariculture zone. Chemosphere, 245, 125636. doi:10.1016/j.chemosphere.2019.125636
- Ma, J., Zhou, B., Chen, F., & Pan, K. (2021). How marine diatoms cope with metal challenge: Insights from the morphotype-dependent metal tolerance in Phaeodactylum tricornutum. Ecotoxicology and Environmental Safety, 208, 111715. doi:10.1016/j.ecoenv.2020.111715
- Mehta, S. K., & Gaur, J. P. (2005). Use of algae for removing heavy metal ions from wastewater: Progress and prospects. Critical Reviews in Biotechnology, 25, 113–152. doi:10.1080/07388550500248571
- Moheimani, N. R. (2013). Inorganic carbon and pH effect on growth and lipid productivity of tetraselmis suecica and Chlorella sp (chlorophyta) grown outdoors in bag photobioreactors. Journal of Applied Phycology, 25, 387–398. doi:10.1007/s10811-012-9873-6
- Nagalakshmi, N., & Prasad, M. N. V. (2001). Responses of glutathione cycle enzymes and glutathione metabolism to copper stress in Scenedesmus bijugatus. Plant Science, 160, 291–299. doi:10.1016/S0168-9452(00)00392-7
- Navarrete, A., González, A., Gómez, M., Contreras, R. A., Díaz, P., Lobos, G. … Moenne, A. (2019). Copper excess detoxification is mediated by a coordinated and complementary induction of glutathione, phytochelatins and metallothioneins in the green seaweed Ulva compressa. Plant Physiology and Biochemistry, 135, 423–431. doi:10.1016/j.plaphy.2018.11.019
- Naveed, S., Li, C., Lu, X., Chen, S., Yin, B., Zhang, C., & Ge, Y. (2019). Microalgal extracellular polymeric substances and their interactions with metal(loid)s: A review. Critical Reviews in Environmental Science and Technology, 49, 1769–1802. doi:10.1080/10643389.2019.1583052
- Neumann, P. M., De Souza, M. P., Pickering, I. J., & Terry, N. (2003). Rapid microalgal metabolism of selenate to volatile dimethylselenide. Plant, Cell & Environment, 26, 897–905. doi:10.1046/j.1365-3040.2003.01022.x
- Noctor, G., Strohm, M., Jouanin, L., Kunert, K., Foyer, C. H., & Rennenberg, H. (1995). Synthesis of glutathione in leaves of transgenic poplar overexpressing y-glutamylcysteine synthetase. Plant Physiology, 112, 1071–1078. doi:10.1104/pp.112.3.1071
- Onyancha, F., Lubbe, D., & Brink, H. G. (2021). Enhancing low-carbon wastewaters with flue gas for the optimal cultivation of Desmodesmus multivariabilis. Chemical Engineering Transactions, 86, 355–360. doi:10.3303/CET2186060
- Oukarroum, A. (2016). Alleviation of Metal-Induced Toxicity in Aquatic Plants by Exogenous Compounds: A Mini-Review. Water, Air, & Soil Pollution, 227. doi:10.1007/s11270-016-2907-y
- Ozturk, S., Aslim, B., & Suludere, Z. (2010). Cadmium(ii) sequestration characteristics by two isolates of Synechocystis sp. in terms of exopolysaccharide (EPS) production and monomer composition. Bioresource Technology, 101, 9742–9748. doi:10.1016/j.biortech.2010.07.105
- Ozturk, S., Aslim, B., Suludere, Z., & Tan, S. (2014). Metal removal of cyanobacterial exopolysaccharides by uronic acid content and monosaccharide composition. Carbohydrate Polymers, 101, 265–271. doi:10.1016/j.carbpol.2013.09.040
- Patterson, J. W. (1985). Industrial wastewater treatment technology (Second edition (2nd ed.). Stoneham, USA: Butterorth Publisher.
- Pawlik-Skowron, B. (2001). Phytochelatin production in freshwater algae Stigeoclonium in response to heavy metals contained in mining water; effects of some environmental factors. Aquatic Toxicology, 52, 241–249. doi:10.1016/S0166-445X(00)00144-2
- Pawlik-Skowrońska, B. (2003). When adapted to high zinc concentrations the periphytic green alga Stigeoclonium tenue produces high amounts of novel phytochelatin-related peptides. Aquatic Toxicology, 62, 155–163. doi:10.1016/S0166-445X(02)00080-2
- Pawlik-Skowrońska, B., & Bačkor, M. (2011). Zn/pb-tolerant lichens with higher content of secondary metabolites produce less phytochelatins than specimens living in unpolluted habitats. Environmental and Experimental Botany, 72, 64–70. doi:10.1016/j.envexpbot.2010.07.002
- Peckol, P., DeMeo-Anderson, D., River, J., Valiela, I., Maldonado, M., & Yates, J. (1994). Growth, nutrient uptake capacities and tissue constituents of the macroalgae Cladophora vagabunda and Gracilaria tikvahiae related to site-specific nitrogen loading rates. Marine Biology, 121, 175–185. doi:10.1007/BF00349487
- Perales-Vela, H. V., Peña-Castro, J. M., & Cañizares-Villanueva, R. O. (2006). Heavy metal detoxification in eukaryotic microalgae. Chemosphere, 64, 1–10. doi:10.1016/j.chemosphere.2005.11.024
- Pérez-Rama, M., Torres Vaamonde, E., & Abalde Alonso, J. (2006). Composition and production of thiol constituents induced by cadmium in the marine microalga Tetraselmis suecica. Environmental Toxicology and Chemistry, 25, 128–136. doi:10.1897/05-252R.1
- Pesce, S., Ghiglione, J. F., & Martin-Laurent, F. (2017). Microbial Communities as Ecological Indicators of Ecosystem Recovery Following Chemical Pollution. In C. Cravo-Laureau, C. Cagnon, B. Lauga, & R. Duran (Eds.), Microbial Ecotoxicology Cham: Springer. doi:10.1007/978-3-319-61795-4_10
- Piña-Olavide, R., Paz-Maldonado, L. M. T., Alfaro-De La Torre, M. C., García-Soto, M. J., Ramírez-Rodríguez, A. E., Rosales-Mendoza, S. , and García De la-Cruz, R. F. (2020). Increased removal of cadmium by Chlamydomonas reinhardtii modified with a synthetic gene for γ-glutamylcysteine synthetase. International Journal of Phytoremediation, 22, 1269–1277. doi:10.1080/15226514.2020.1765138
- Pinto, E., Carvalho, A. P., Cardozo, K. H. M., Malcata, F. X., dos Anjos, F. M., & Colepicolo, P. (2011). Effects of heavy metals and light levels on the biosynthesis of carotenoids and fatty acids in the macroalgae Gracilaria tenuistipitata (var. liui Zhang & Xia). Brazilian Journal of Pharmacognosy, 21, 349–354. doi:10.1590/S0102-695X2011005000060
- Pistocchi, R., Mormile, M. A., Guerrini, F., Isani, G., & Boni, L. (2000). Increased production of extra- and intracellular metal-ligands in phytoplankton exposed to copper and cadmium. Journal of Applied Phycology, 12, 469–477. doi:10.1023/A:1008162812651
- Priyadarshanee, M., & Das, S. (2021). Biosorption and removal of toxic heavy metals by metal tolerating bacteria for bioremediation of metal contamination: A comprehensive review. Journal of Environmental Chemical Engineering, 9, 104686. doi:10.1016/j.jece.2020.104686
- Qiu, R., Gao, S., Lopez, P. A., & Ogden, K. L. (2017). Effects of pH on cell growth, lipid production and CO2 addition of microalgae Chlorella sorokiniana. Algal Research, 28, 192–199. doi:10.1016/j.algal.2017.11.004
- Rajfur, M. (2013). Algae - heavy metals biosorbent. Ecological Chemistry and Engineering, 20, 23–40. doi:10.2478/eces-2013-0002
- Rezayian, M., Niknam, V., & Ebrahimzadeh, H. (2019). Oxidative damage and antioxidative system in algae. Toxicology Reports, 6, 1309–1313. doi:10.1016/j.toxrep.2019.10.001
- Ribeiro, C., Couto, C., Ribeiro, A. R., Maia, A. S., Santos, M., Tiritan, M. E. … Almeida, A. A. (2018). Distribution and environmental assessment of trace elements contamination of water, sediments and flora from Douro River estuary, Portugal. The Science of the Total Environment, 639, 1381–1393. doi:10.1016/j.scitotenv.2018.05.234
- Romano, R. L., Liria, C. W., Machini, M. T., Colepicolo, P., & Zambotti-Villela, L. (2017). Cadmium decreases the levels of glutathione and enhances the phytochelatin concentration in the marine dinoflagellate Lingulodinium polyedrum. Journal of Applied Phycology, 29, 811–820. doi:10.1007/s10811-016-0927-z
- Rüegsegger, A., & Brunold, C. (1992). Effect of cadmium on γ-glutamylcysteine synthesis in maize seedlings. Plant Physiology, 99, 428–433. doi:10.1104/pp.99.2.428
- Sánchez-Thomas, R., García-García, J. D., Marín-Hernández, Á., Pardo, J. P., Rodríguez-Enríquez, S., Vera-Estrella, R. … Moreno-Sánchez, R. (2020). The intracellular water volume modulates the accumulation of cadmium in Euglena gracilis. Algal Research, 46, 101774. doi:10.1016/j.algal.2019.101774
- Schäfer, H. J., Haag-Kerwer, A., & Rausch, T. (1998). cDNA cloning and expression analysis of genes encoding GSH synthesis in roots of the heavy-metal accumulator Brassica juncea L.: Evidence for Cd-induction of a putative mitochondrial γ-glutamylcysteine synthetase isoform. Plant Molecular Biology, 37, 87–97. doi:10.1023/A:1005929022061
- Sinaei, M., Loghmani, M., & Bolouki, M. (2018). Application of biomarkers in brown algae (Cystoseria indica) to assess heavy metals (Cd, Cu, Zn, Pb, Hg, Ni, Cr) pollution in the northern coasts of the Gulf of Oman. Ecotoxicology and Environmental Safety, 164, 675–680. doi:10.1016/j.ecoenv.2018.08.074
- Singh, S. P., & Schwan, A. L. (2019). Sulfur metabolism in plants and related biotechnologies. In Comprehensive Biotechnology (Vol. 4, Third Edit ed.). Elsevier. doi:10.1016/B978-0-444-64046-8.00225-1
- Skowroński, T., De Knecht, J. A., Simons, J., & Verkleij, J. A. C. (1998). Phytochelatin synthesis in response to cadmium uptake in Vaucheria (xanthophyceae). European Journal of Phycology, 33, 87–91. doi:10.1080/09670269810001736573
- Soldo, D., & Behra, R. (2000). Long-term effects of copper on the structure of freshwater periphyton communities and their tolerance to copper, zinc, nickel and silver. Aquatic Toxicology, 47, 181–189. doi:10.1016/S0166-445X(99)00020-X
- Stevens, A. E., Mccarthy, B. C., & Vis, M. L. (2001). Metal content of Klebsormidium-dominated (chlorophyta) algal mats from acid mine drainage waters in Southeastern Ohio. Journal of the Torrey Botanical Society, 128, 226–233. doi:10.2307/3088714
- Sud, D., Mahajan, G., & Kaur, M. P. (2008). Agricultural waste material as potential adsorbent for sequestering heavy metal ions from aqueous solutions - a review. Bioresource Technology, 99, 6017–6027. doi:10.1016/j.biortech.2007.11.064
- Taboada-de la Calzada, A., Villa-Lojo, M. C., Beceiro-González, E., Alonso-Rodrı́guez, E., & Prada-Rodrı́guez, D. (1998). Determination of arsenic species in environmental samples : Use of the alga Chlorella vulgaris for Arsenic (III) retention. Trends in Analytical Chemistry, 17, 167–175. doi:10.1016/S0165-9936(98)00002-8
- Torricelli, E., Gorbi, G., Pawlik-Skowronska, B., Di Toppi, L. S., & Corradi, M. G. (2004). Cadmium tolerance, cysteine and thiol peptide levels in wild type and chromium-tolerant strains of Scenedesmus acutus (chlorophyceae). Aquatic Toxicology, 68, 315–323. doi:10.1016/j.aquatox.2004.03.020
- Tüzün, I., Bayramoǧlu, G., Yalçin, E., Başaran, G., Çelik, G., & Arica, M. Y. (2005). Equilibrium and kinetic studies on biosorption of Hg(II), Cd(II) and Pb(II) ions onto microalgae Chlamydomonas reinhardtii. Journal of Environmental Management, 77, 85–92. doi:10.1016/j.jenvman.2005.01.028
- Volland, S., Schaumlöffel, D., Dobritzsch, D., Krauss, G., & Lütz-Meindl, U. (2013). Chemosphere identification of phytochelatins in the cadmium-stressed conjugating green alga Micrasterias denticulata. Chemosphere, 91, 448–454. doi:10.1016/j.chemosphere.2012.11.064
- Wahal, S., & Viamajala, S. (2010). Maximizing algal growth in batch reactors using sequential change in light intensity. Applied Biochemistry and Biotechnology, 161, 511–522. doi:10.1007/s12010-009-8891-6
- Wang, M. J., & Wang, W. X. (2008). Temperature-dependent sensitivity of a marine diatom to cadmium stress explained by subcelluar distribution and thiol synthesis. Environmental Science & Technology, 42, 8603–8608. doi:10.1021/es801470w
- Wang, M. J., & Wang, W. X. (2011). Cadmium sensitivity, uptake, subcellular distribution and thiol induction in a marine diatom: Recovery from cadmium exposure. Aquatic Toxicology, 101, 387–395. doi:10.1016/j.aquatox.2010.11.012
- Wood, J. M. (1985). Effects of Acidification on the Mobility of Metals and Metalloids : An Overview. Environmental Health Perspective, 63, 115–119.
- Zbikowski, R., Szefer, P., & Latała, A. (2007). Comparison of green algae Cladophora sp. and Enteromorpha sp. as potential biomonitors of chemical elements in the southern Baltic. The Science of the Total Environment, 387, 320–332. doi:10.1016/j.scitotenv.2007.07.017
- Zeng, J., Yang, L., & Wang, W. X. (2009). Acclimation to and recovery from cadmium and zinc exposure by a freshwater cyanobacterium, Microcystis aeruginosa. Aquatic Toxicology, 93, 1–10. doi:10.1016/j.aquatox.2009.02.013
- Zeraatkar, A. K., Ahmadzadeh, H., Talebi, A. F., Moheimani, N. R., & McHenry, M. P. (2016). Potential use of algae for heavy metal bioremediation, a critical review. Journal of Environmental Management, 181, 817–831. doi:10.1016/j.jenvman.2016.06.059
- Zucchi, M. R., & Necchi, O. J. (2001). Effects of temperature, irradiance and photoperiod on growth and pigment content in some freshwater red algae in culture. Phycological Research, 49, 103–114. doi:10.1111/j.1440-1835.2001.tb00240.x