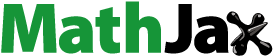
ABSTRACT
POME is an excellent cultivation medium for the production of microalgae because it includes high macro and micronutrients. Nevertheless, microalga cultivation is laborious to maintain under outdoor condition. The aim of this reseasch was to evaluate the potency of co-cultivation strategy to improve growth and lipid production of mixed algae when growing on POME under dynamic outdoor condition. Cultivation was done in outdoor condition by comparing synthetic medium and POME medium, and optimizing the enviromental and nutritional conditions by using Box-behnken response surface methodology (RSM). Results showed that high growth rate (0.35/d) and lipid (40%) were achieved when 75% POME fraction, 30 PSU salinity, and 450 mg/L urea were used. The increasing POME fraction and urea addition enhanced SFA formation to MUFA and PUFA in the fatty acid profiles. We conclude that co-cultivation strategy on POME could promote growth rate and lipid production of mixed algae under outdoor condition.
1. Introduction
Palm oil usage has been rising worldwide due to its many use as a food ingredient and for other industrial uses, particularly the development of biofuels in response to the global carbon neutrality strategy [Citation1]. Indonesia`s main source of income is oil palm, which is also considered to be one of the main factors reducing the nation’s carbon emissions due to its potential application as a biodiesel source [Citation2,Citation3]. According to estimates, Indonesia will produce 17 million tons of oil palm in 2022, which account for 19% of the world’s supply of palm oil [Citation4]. Conversely, the increase in palm oil production generates higher palm oil mill effluent. In wet processing, 1 tonne of crude palm oil will generate more than 50% of POME [Citation5]. Although POME mainly contains materials that biodegradable, its untreated discharge is a significant cause of pollution in some areas [Citation6]. However, POME are rich in macro and micronutrients that could be utilized for microorganism such as bacteria, yeast, and microalgae for the growth to produce valuable products [Citation7].
Previous researchers demonstrated the utilization of POME as medium growth of microalgae to produce biofuel, animal feed, biopolymer, and pigments as reported previously [Citation8–11]. Microalgae is a promising renewable, sustainable, and valueable source since it would not compete with other agriculture products, the cultivation does not need arable land, it can also be cultured using non-drinkable water (i.e. wastewater, seawater), and the productivity is higher compared to other agriculture commodities [Citation12,Citation13]. Several commercial microalgae are produced such as Spirulina sp., Dunaliella salina, Chaetoceros calcitrans, and Nannochloropsis oculata for several purposes, including pharmaceutical, food and fuel sources [Citation14]. The cultivation of microalgae by using wastewater to produce high lipid for biofuel and other valuable products seems promising since the production cost can be minimized [Citation15]. Citation16,reported the cultivation of Thalassiosira pseudonana by using 20% POME and resulted 46% d.w. lipid production where the fatty acid was mainly consisted of saturated fatty acid. Citation17,found that Nannochloropsis sp. could accumulate 60% lipid when growing on POME supplemented with 40% synthetic medium. POME was able to improve lipid yield of for C. pyrenoidosa [Citation18]. Previous finding reported the utilization of POME by Isocrysis sp growing under outdoor condition to produce lipid. However, only low POME had been used (5%) in the medium [Citation19]. These finding were performed in controlled nutritional and environmental conditons which were optimized for the growth.
There are several challenges in the utilization of wastewater for microalgal growth when growing on large-scale open pond outdoor condition since the effect of dynamic changes of environmental conditions, dynamic changes in wastewater, and the availability of contamination could lower the quality and quantity of the biomass produced [Citation20,Citation21,Citation22]. Therefore, scaling up of microalgae cultivation on wastewater by using monoculture is difficult to maintain and laborious [Citation23,Citation24]. It is reported that co-cultivation (polyculture) of microalgae by using bacteria, fungi, yeast, and other microalgae could solve the problems [Citation25,Citation26,Citation27]. In co-cultivation condition, microalgae grow symbiotically and could adapt better on dynamic environmental and nutritional conditions [Citation22,Citation28,Citation29]. Nutritional and environmental conditions are important for the microalgal growth when the cultivation is applied on a large scale production [Citation30]. It is hypothesized that mixed algae could grow well on high POME fractions and under outdoor condition to produce lipid and high growth rate. The aim of this research was to study microalgae cultivation under dynamic environmental and nutritional condition and comparing the cultivation mode (monoculture or mixed cultures) of Spirulina sp., Nannochloropsis sp., Dunaliella sp., and Chaetoceros calcitrans when growing on synthetic medium or POME. Optimal nutritional and evironmental conditions were also studied by using response surface methodology (RSM) to obtain optimal urea, POME, and salinity condition.
2. Material and methods
Four microalgae strains were used: Dunaliella sp, Spirulina sp., Nannochloropsis sp, and Chaetoceros calciltrans. These strains were selected due to the ability to grow in large scale/ mass cultivation for commercial production. As stated previously, Spirulina (Arthrospira) platensis could adapt well on high POME fractions, while the other strains contained high lipid yield [Citation30, Citation14]. All the strains were obtained from Balai Besar Perikanan Budidaya Air Payau (BBPBAP) Jepara, Central Java, Indonesia. Dunaliella sp. was maintained on Conway medium at 30 PSU salinity with the composition as described previously [Citation31]. Nannochloropsis sp. and Chaetoceros calcitrans were maintained on f/2 medium at 25 PSU salinity with the composition as described previously [Citation32]. Spirulina sp. was maintained on Zarrouk medium at 15 PSU salinity as described previously [Citation33]. In total, two experiments were done using a stepwise approach (). In experiment I, mixed cultures and monocultures were grown in outdoor condition by using synthetic medium at different salinity. In experiment II, mixed cultures were cultivated in outdor condition by using POME medium. Salinity, POME fractions, and urea addition were varied and optimized by using Box-behnken RSM design.
2.1. Pre-treatment of POME
POME was obtained from a small factory in Sumatra, Indonesia. POME was collected from final dischage after treated using traditional anaerobic and aerobic open pond treatment. POME contained 2030 mg/L chemical oxygen deman (COD), 200 mg/L total nitrogen, and 3 mg/L dissolved phosphate. POME was pretreated using GF/C filter paper (Whatman) to remove suspended solid and then autoclaved at 121°C for 15 min [Citation30,Citation34].
2.2. Microalgae cultivation in synthetic medium
Cultivation was carried out in two stages: indoor and outdoor condition (Fig S1). Indoor cultivation was performed to maintain the cells before transferring to outdoor condition. For indoor cultivation, microalgae were cultured in 1.5 L polyethilene bottles (with working volume 1 L) at 28°C, pH was maintained at 8.5 by using 0.5 N NaOH or HCl, and light intensity was provided at 200 μmol photons/ m2 /s in a 16:8 day:night shift by using fluorescent lamp (Philips, Indonesia). Salinity was adjusted using commercial salt at it`s optimal condition as stated previously. The bottles were shaken manually three times a day to mix the cultures. The growth of microalgae were monitored by using spectrophotometer Hach DR 2400 at 750 nm. When reached in exponential phase, microalgae were transfered to 200 mL (working volume 150 mL) transparant polypropylene sterile infusion bags to acclimatize the cultures in outdoor condition.
Acclimatization of the microalgae was performed in outdoor condition, located in Center of Biomass and Renewable Energy, UNDIP Tembalang, Semarang (location: 7°03ʹ16.0”S 110°26ʹ23.5”E) in the middle of January until March 2019. The acclimation process was performed for microalgae to shorten lag phase. The sterile infusion bags containing 10% v/v microalgae strain from indoor cultivation and the medium were immersed in a 100 L water basin. The container was equipped with a shading mesh to reduce the penetration of light so it would not reach above 300 μmol photons/ m2 /s. Light intensity was measured above the surface of the water basin. Salinity was maintained at 15 to 30 PSU salinity at the optimal salinity of the selected strain. pH was adjusted at 8.5 by using 0.5 N NaOH or HCl. Temperature and light intensity were not maintained and measured everyday at 09:00, 12:00, and 15:00 for 7 weeks to record the dynamic evironmental profiles. The infusing bags were manually shaken three times a day to mix the cultures. The growth of microalgae were monitored by using spectrophotometer (see analyses section below). The acclimatization was done when the culture reached the end of exponential phase and ready to be used as inoculum for further experiment.
In experiment I, 10% v/v acclimatized microalgae were used for inoculum. Each microalgae was grown in the optimized medium (Zarrouk, Conway, or f/2). Salinity was varied (15, 20, 30 PSU) by using commercial salt. Microalgae were grown in the sterile 150 mL infusing bags as mentioned above. The growth was then monitored until reach the end of exponential phase. The growth of microalgae were monitored by using spectrophotometer (see below). At the end of exponential phase, the cultures were harvested for further analysis (see section analyses below).
For co-cultivation condition, each 2.5% of total 10% v/v four acclimatized microalgae [optical density 0.4 at 750 nm): Dunaliella sp, Nannochloropsis sp, Spirulina sp, and Chaetoceros calcitrans were used as inoculum in a modified commercial medium consisting of 3 g/L NaHCO3, 1 g/L NaNO3, 20 mg/L KH2PO4, and micronutrients as described in Citation32. All the chemicals used were of technical grade obtained from a local store in Indonesia. pH was controlled in 8.5 using 0.5 M NaOH or 0.5 M HCl. Salinity was varied using commercial salt at 15, 20, and 30 PSU. Microalgae were grown in the sterile 150 mL infusing bags. The cultivation was carried out in outdoor conditions. Infusing bags were manually shaken three times a day to mix the cultures. The growth of microalgae was monitored daily by using spectrophotometer (Hach DR 2400]. Microalgae were cultured until reach the end of exponential phase and harvested for further analysis.
2.3. Effect of POME, salinity, and urea addition
In experiment II, microalgae were grown by using 2.5% of total 10% v/v four acclimatized microalgae strains (0.4 optical density at 750 nm). Box-behnken response surface methodology (RSM) was used to design the experiment. POME: 30–75% v/v (diluted using synthetic medium), urea: 0–750 mg/L, and salinity: 15–30 PSU were used to optimize the growth rate, biomass, lipid production and fatty acid ratio. Salinity was adjusted by using a commercial salt, pH was controlled in 8.5 using 0.5 M NaOH or 0.5 HCl, and POME was diluted using double distilled water. The sterile bags were placed in a water basin at outdoor condition as stated previously. The growth of microalgae was monitored daily by using spectrophotometer. Samples (5 mL) were taken and centrifuged at 2000 x g for 15 min to separate the algal biomass from POME due to the possible interference of the color from POME in the spectrophotometry measurements as described previously [Citation34]. At the end of exponential phase, the cultures were harvested for further analysis (see analysis section below).
2.4. Analyses
2.4.1. Growth rate and biomass determination
Microalgae was monitored indirectly by using a spectrophotometer at 750 nm to evaluate the growth. A linear correlation between dry biomass and absorbance was used for co-cultivation was shown in EquationEq 1(1)
(1) .
where XM is biomass dry weight (g/L) of the mixture from co-cultivation, and ODM is optical density at 750 nm from the mixture of co-cultivation: Dunaliella sp, Nannochloropsis sp, Spirulina sp., and Chaetoceros calcitrans.
For Dunaliella sp, a linear correlation between dry biomass and absorbance was shown in EquationEq. 2(2)
(2) .
where XD is biomass dry weight (g/L) for Dunaliella sp, and ODD is optical density at 750 nm for Dunaliella sp.
For Nannochloropsis sp, a linear correlation between dry biomass and absorbance was shown in EquationEq. 3(3)
(3) .
where XN is biomass dry weight (g/L) for Nannochloropsis sp, and ODN is optical density at 750 nm for Nannochloropsis sp.
For Nannochloropsis sp, a linear correlation between dry biomass and absorbance is shown in EquationEq. 4(4)
(4) .
where Xs is biomass dry weight (g/L) for Spirulina sp, and ODN is optical density at 750 nm for Spirulina sp.
For Chaetoceros calcitrans, a linear correlation between dry biomass and absorbance is shown in EquationEq. 5(5)
(5) .
where XC is biomass dry weight (g/L) for Chaetoceros calcitrans, and ODC is optical density at 750 nm for Chaetoceros calcitrans.
To prepare dry biomass, the samples were collected by using Whatmant GF/C filter paper, washed using NH3HCO3 and oven-dried at 95°C until constan weight reached [Citation30a]. Gravimetric analysis was used to determine the dry biomass.
2.4.2. Lipid extraction
Lipid extraction was performed by using modified Bligh-dyer method as stated previously with little modifications [Citation35]. The extraction was done in a single-step procedure by using 8 mL of 2:1 chloroform-methanol (v/v) mixture. Dry biomass (100 mg) was placed in a glass centrifuge tube, mixed with the solvents and extracted in a ultrasonic water bath equipped with a water heater (120 W) for 15 min at 40°C. After extraction, 2 ml of a 0.73% NaCl water solution was introduced to the tube, then centrifugated at 1000 x g for 5 min. The lower phase containing lipid and chloroform was collected, gently transferred in a pre-weighed dried aluminum cup, and evaporated at 80°C for 1 h under N2 in acseptic hood to avoid oxidation of the lipids. The collected lipid was analyzed by gravimetric analysis.
2.4.3. Fatty acid analysis
Fatty acid determinated was performed as stated previously [Citation36]. Lipids were extracted as mentioned previously (see section 2.4.2). After extraction, dry lipid was resuspended in a mixture of 1 mL chloroform and toluene (1:1, v/v) in a glass tube, then a known amount of nonadecanoic methyl ester (Sigma) was added as an internal standard. The lipids were transesterified to produce fatty acid methyl esters (FAMEs). One milliliter of MeOH–KOH (0.2 N) was added, and the mixture was incubated for 15 min at 37°C. After neutralizing to pH 6 with acetic acid (1 M), 2 mL of chloroform and 2 mL of Milli-Q water were added to the tube. After centrifugation, the chloroform phase with the FAMEs was recovered and the nonpolar FAMEs were separated from the polar compounds over a small Al2O3 column using dichloromethane (DCM) as eluent.
FAMEs were analyzed on a Hewlett-Packard 5890 gas chromatograph equipped with both a flame ionization detector (FID) and a HP 5972 mass spectrometer detector (MSD). Samples of 5 μL were injected using an HP 6890 autosampler at 60°C on a Restek RTX 1701 (60 m × 0.25 mm, film thickness 0.25 µm) column. The temperature was held at 60°C for 1 min and then increased at a rate of 10°C/min until 180°C and then to a final temperature of 250°C at 5°C/min, which was maintained for 15 min. The injection temperature was 250°C, and the detector temperatures were both 280°C. Long-chain fatty acids (number of carbons ≥14) were identified from mass spectra and retention times by comparison with those of PUFA No. 1 standard mixture (Matreya LLC, USA). Quantification of the fatty acids was done by integration of peak areas calibrated with the known internal standard which was added to the samples.
2.5. Statistical analysis
Differences between treatments and the interaction of monocultivation and co-cultivation in synthetic medium (section 2.2) were analyzed with analysis of variance (ANOVA). Minitab ver. 18 (demo version) was employed for statistical analysis and evaluation for Box-behnken RSM design in experiment II (section 2.3). Significant (P < 0.05) differences among treatments were further analyzed by pairwise comparisons using Tukey (Post Hoc Test). The experimental results were recorded based on three replicates (n = 3) except for fatty acid profiles, and expressed as the means and standard deviations (± SD).
3. Results
3.1. Co-cultivation in outdoor condition
In experiment I, monocultivation and co-cultivation were grown in outdoor condition. It is recorded that in January, the temperature was around 26 to 27°C, while in February, the temperature was increased from 27 to 29°C () due to the shifting from rainy to dry season. While for light intensity, the value was in range of 100 to 300 μmol photons/ m2 /s (). In monoculture, high salinity significantly reduced the growth in Spirulina sp. and Chaetoceros calcitrans (P < 0.05). The lowest growth rate was recorded from Spirulina sp., at 30 PSU salinity (0.14/d), followed by Chaetoceros calcitrans at 30 PSU salinity (0.27/d). For Nannochloropsis sp. and Dunaliella sp., and mixed culture, salinity did not have significant effect to the growth rate (). At overall, different cultures influenced the growth, while salinities did not significantly influence (P > 0.05). The interaction of salinity and different kind of species did not significantly influence the growth rate (P > 0.05).
Figure 2. Outdoor temperature inside water basin.O is recorded at 09:00 AM, Δ is recorded at 12:00 AM, and ▢ is recorded at 15:00 PM.
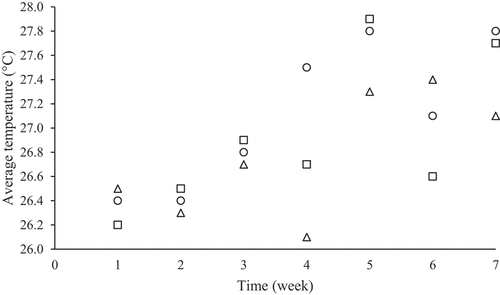
Figure 3. Outdoor light intensity at the surface of the water basin. SymbolO is recorded at 09:00 AM, symbol ▢ is recorded at 12:00 AM, and symbol Δ is recorded at 15:00 PM.
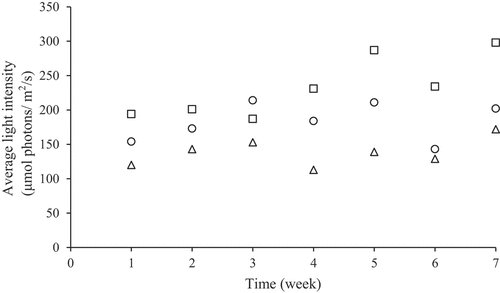
Table 1. Effect of different salinites on growth rate, final biomass, and lipid production of microalgae growing under outdoor condition. Average values (n = 3) are shown. SD are shown after ± symbol. Values that do not share letter are significantly different (P < 0.05).
The highest final biomass production was recorded in mixed cultures group (around 220–230 mg/L). Different cultures and different salinities significantly influenced final biomass. The interaction of salinities and cultures influenced the final biomass (P < 0.05). Low final biomass was recorded in Nannochloropsis sp. (111 mg/L), Spirulina sp. (58 mg/L), and Chaetoceros calcitrans (72 mg/L) when cultured in 30 PSU salinity, while Dunaliella sp produced low final biomass (111–142 mg/L) below 30 PSU salinity.
shows lipid production of different cultures cultivated in different salinities. The highest lipid production was found on mixed cultures followed by monoculture of Nannochloropsis sp cultivated in all salinites (33–35%dw). The lowest lipid production was found on Spirulina sp. in 15 PSU salinity (10%). When salinity was increased to 30 PSU, lipid production was increased to 15% in Spirulina sp. For Dunaliella sp., the cultivation at lower salinity produced higher lipid production. Salinity, cultures and the interaction between salinity and the cultures significantly influenced lipid production (P < 0.05). Co-cultivation strategy enhanced lipid production more than 30% compared to monoculture of Dunaliella sp and Spirulina sp.
3.2. Effect of POME, salinity, and urea
When growing on POME, the increasing of salinity significantly enhanced growth rate of mixed cultures (P < 0.05), while the other factors (POME, urea) did not influence growth rate (, Table S1). Higher POME fractions resulted higher growth rate, but not significant (P > 0.05). The highest growth rate (0.35/day) was recorded at optimal salinity 22 PSU and optimal urea 450 mg/L. The interaction of salinity and urea was slightly significant (P = 0.072).
Figure 4. Response surface plot (3D) of growth rate (/day) from co-cultivation of mixed cultures as a function of POME, salinity, and urea addition at hold value a) 50% POME, b) 22.5 PSU salinity, and c) 450 mg/L urea.
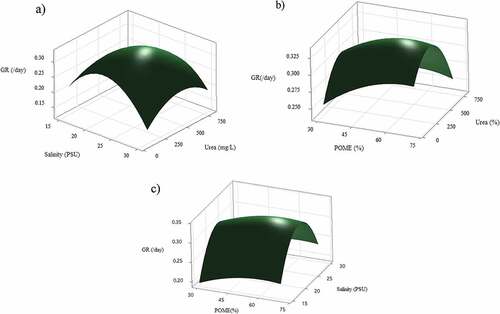
shows Box-behnken RSM of lipid production from co-cultivation in different POME, salinity, and urea addition. The interaction of POME and salinity significantly enhanced lipid production, followed by effect of salinity, and the interaction of POME and urea addition (Fig S2). High urea addition above 450 mg/L significantly lower lipid production when high POME fraction was added (). Low POME fraction and low salinity significantly promoted lipid production to 50% d.w., while high salinity (above 25 PSU) and high POME fraction (75%) increased lipid production to 40% d.w. (). A model of lipid production was generated from Box-behnken RSM (EquationEq 6)(6)
(6) .
Figure 5. Response surface plot (3D) of lipid production (% d.w.) from co-cultivation of mixed cultures as a function of POME, salinity, and urea addition at hold value a) 450 mg/L urea, b) 22.5 PSU salinity, and c) 50% POME.
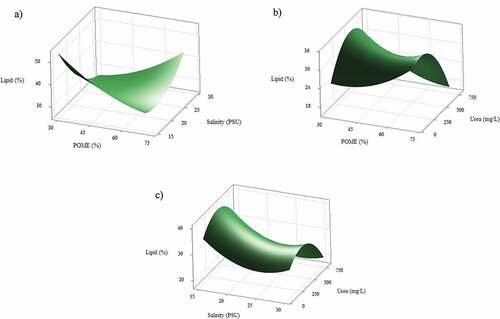
where X1, X2, and X3 are POME fraction (%), salinity (PSU), and urea (mg/L), respectively. The observed and predicted value of lipid production generated from Box-behnken RSM was shown in .
Table 2. Observed and predicted value of PUFA+MUFA to SFA ratio from mixed algae growing on different POME fractions, salinity, and urea addition. Average values of observed lipid are shown (n = 3). SD are shown after ± symbol.
shows Box-behnken RSM of polyunsaturated fatty acid (PUFA) and monounsaturated fatty acid (MUFA) to saturated fatty acid (SFA) ratio from mixed algae growing on different POME fractions, salinity, and urea addition. Urea addition strongly influenced fatty acid profiles in mixed cultures (, Table S2). Higher MUFA and PUFA were found in the fatty acid (FA) profiles when higher urea above 500 mg/L or low urea below 250 mg/L was added to the medium (P < 0.05). When 250–500 mg/L urea was added to the medium, higher SFA dominated fatty acid profiles (). The interaction of POME and urea significantly influenced fatty acid profiles (P < 0.05). When growing on low POME fraction, low urea addition promoted MUFA and PUFA instead of SFA (). The interaction of high salinity and low POME fractions seems increased MUFA and PUFA to SFA, but not significant (P > 0.05) () (Table S2). In overall, salinity was not significantly influence the fatty acid ratio.
4. Discussion
Cultivation was done in outdoor condition from the middle of January to March 2019. Based on , temperature was recorded at around 26–28°C which is suitable for tropical microalgae to grow [Citation37]. Maximal light intensity on the surface ot the water basin was recorded at 300 μmol photons/ m2 /s. Light intensity below 300 μmol photons/ m2 /s was needed to avoid saturation in photosynthetic process for selected microalgae during the growth [Citation30a].
In this research, co-cultivation was performed in outdoor condition where the environmental conditions were not controlled (). Co-cultivation of microalgae was found to produce more stable and consistent growth compared to monoculture. When co-cultivation was done in different salinities, the final biomass and growth rate were stable (). Previous researcher mentioned that co-cultivation strategy composed of various strains would be less susceptible to environmental shifts (temperature and light intensity), and produce higher biomass than a monoculture [Citation38,Citation39]. Other finding reported that co-cultivation of two microalgae (Chlorella sp. and Monoraphidium sp.) significantly increased the accumulation of total biomass and enhanced the lipid productivity compared to mono cultivation [Citation40]. Citation41,informed that co-cultivation of Chlorella vulgaris and Tetradesmus obliquus in outdoor and non-sterile medium condition produced higher biomass productivity. Mixed cultures form as a colony or consortium in the microalgal-microalgal co-culture system which each individual in a co-culture system has the ability to benefit from the activities of the others in a symbiotic relationship [Citation27]. One species gains an advantage at the expense of the other’s declining fitness through parasitism or exploitation in a competitive relationship [Citation42].
When using POME medium, the growth of mixed cultures was not significantly different compared to synthetic medium (, ). The species could adapt better on 75% POME fractions that contain higher organic compounds (phenols, acids). Latest researcher reported that organic acids (acetic acid, butyric acid, formic acid) contained in POME exhibit the growth of mixed cultures (Coelastrella sp. UKM 4, Chlorella sp. UKM 8 and Scenedesmus sp) [Citation43]. It is reported that the biomass production of microalgae co-culture was higher when growing on 15% POME compared to bold basal medium [Citation44]. Previous researchers mentioned that co-cultivation strategy in wastewater could push the biomass production and nutrient removal due to the stabilty of the culture grown in a wide range environmental and nutritional conditions [Citation22]. Since microalgal monocultures are laborious to maintain in wastewater, mixed cultures offer improved system stability and robustness [Citation29]. Previous researchers highlighted that when growing on synthetic wastewater, mixed culture systems consisted of Chlorella vulgaris, Chlamydomonas reinhardtii, Selenastrum capricornutum, Microcystis aeruginosa, and Eustigmatos vischeri showed more stable biomass growth as compared to monocultures [Citation45].
When mixed cultures were grown on low salinity, lower POME fractions exhibited lipid accumulation. The interaction of POME and salinity significantly influenced lipid production in mixed cultures (P < 0.05). Previous research mentioned that high lipid production was generated when microalgae is grown in unfavorable environmental or nutritional condition by storing the energy in the form of lipid [Citation46,Citation47]. When high salinity and high POME fractions were exposed to the medium, it seems that microalgae which grown optimal in 15–20 PSU salinity (i.e. Spirulina sp.) may produce higher lipid. Conversely, when low salinity and low POME fractions were exposed, marine microalgae such as Dunaliella and Nannochloropsis could produce higher lipid production. When mixed culture was cultivated on low POME and low salinity, high exopolysaccharide might be generated from Dunaliella and Nannochloropsis due to growing in unfavored conditions. The increasing of soluble extracellular materials (e.g. biopolymers) is important as a protective mechanism when growing in dynamic nutritional and environmental conditions such as extreme salinity, high temperature, and toxicity [Citation22, Citation30, Citation45a]. Nevertheless, high exopolysaccharide may inhibit mass transfer of nutrient uptake. Citation48,informed that nutrient uptake efficiency of ammonium, phosphate, and total organic carbon was higher in monoculture compared to co-cultivation of Scenedesmus obliquus and wild algae. Lower nutrient uptake by microalgae may induce the cells to store more energy due to the response of unfavorable condition. Previous research informed that high exopolysaccharide production is associated to the activity of microalgae in storing energy as lipid or carbohydate [Citation30a]. Citation49,found that lipid and exopolysaccharide were increased when Scenedesmus sp was exposed to microwave treatment during the cultivation. Furthermore, Citation50,found that co-cultivation of Monoraphidium sp. and Heveochlorella sp increased lipid productivity compared to monoculture due to upregulation of correlative key genes in lipid anabolic metabolism. Further investigation is needed to understand the interaction of selected factors when growing on POME for monoculture and compared the growth and lipid production to mixed cultures. A model has been proposed for lipid production (EquationEq 6)(6)
(6) . Based on , the predicted lipid content shows similar result as compared to actual lipid content. This indicated that the proposed model could be used when the cultivation would be scaled up in the large production.
In this research, the presence of high POME fraction (75%) and high salinity (35 PSU) could enhance lipid production in mixed cultures from 30 up to 40% d.w. Conversely, when low POME (30%) and low salinity (15 PSU) were used, the production of lipid could reach up to 55% (, S2). The addition of urea from 0 to 750 mg/L on 75% POME significantly decreased lipid content (from 30 to 18%). While the addition of urea at 400–500 mg/L promoted lipid production up to 36% on 30% POME and 20 PSU salinity. Previous research mentioned that the ratio of carbon to nitrogen in the medium plays significant role in lipid accumulation since microalgae could accumulate higher lipid when nitrogen source become limited [Citation51]. The presence of higher POME fraction in the medium, which containing organic acids, could also influence lipid accumulation in microalgae. As stated by previous research, the addition of POME fraction to medium could shift the cultivation condition from autotrophic to mixotrophic [Citation43,Citation52]. In mixotrophic cultivation, microalgae utilize organic and inorganic carbon in the presence of light as dual source of energy, thus resulting higher lipid production in mixed cultures. Citation53,reported that co-cultivation strategy in mixotrophic condition was favorable to accumulate higher lipid production, while co-cultivation in autotrophic condition produced higher protein and carbohydrate content.
It is found that the ratio of MUFA and PUFA to SFA increased from 0.1 to 0.3 when higher POME fraction from 30 to 75% was presented. In this condition, mixed cultures may consume higher organic carbon which consisted of organic acids when higher POME (75%) was presented [Citation43]. Previous research reported that fatty acid profile was influenced by the addition of organic carbon concentration in mixotrophic condition. The increasing of initial glucose in mixotrophic condition resulted lower PUFA content in Chlorella sorokiniana and Auxenochlorella protothecoides [Citation54,Citation55]. Citation56,found that lower PUFA was detected in Chlorella zofingiensis when growing in heterotrophic condition rather than autotrophic condition. High PUFA formation is triggered by inorganic carbon when microalgae is grown in autotrophic condition [Citation57]. It seems that unsaturated fatty acid in microalgae is linked with chlorophyll-a content. When high light intensity and organic carbon were presented, microalgae may produce lower chlorophyll-a to maintain the energy supply in the cells, thus producing high SFA rather than PUFA.
With regard to the influence of urea concentration on the total fatty acid composition, the increasing of urea concentration from 0 to 750 mg/ significantly increased PUFA and MUFA to SFA ratio from 0.15 to 0.45 when co-cultivation was performed on 75% POME. This condition indicated that nitrogen source was sufficient both from urea and inorganic nitrogen contained in POME. This result was in line with previous researchers who reported that higher PUFA and MUFA in Nitzschia closterium were influenced by the addition of higher nitrate concentration in the cultivation medium [Citation58]. Citation59,informed that the addition of nitrogen in the cultivation medium enhanced chlorophyll-a, reduced SFA and promoted unsaturated fatty acid (MUFA and PUFA) in Isochrysis galbana. It seems that high pigment chlorophyll-a content in the cells is linked to high unsaturated fatty acid. Chlorophyll-a is used for microalgae to capture light and synthesize energy in photosynthetic reaction [Citation60]. When the supply of nitrogen depleted, microalgae produce lower chlorophyll-a, and PUFA content in the fatty acid may be used to protect the cells from damage, thus the fatty acid composition is shifted from unsaturated to saturated profile.
As according to , mostly the cultivation condition for microalgae growing on POME was employed in indoor and controlled condition. The highest lipid content was achieved in Chlamydomonas incerta which resulted 60% lipid and utilized 250 mg/L COD from POME [Citation61]. Other findings reported that 10 to 30% POME could be utilized for green microalgae to produce around 27 to 39% lipid [Citation62]. A modified POME and external organic carbon could enhance lipid content [Citation63, Citation65]. Citation19,reported that outdoor cultivation of Isochrysis sp on 5% POME could produce higher biomass and lipid content compared to indoor cultivation. However, this low POME is impractical when adapted in large scale and commercial process.
Table 3. Lipid production from microalgae cultivated on POME in different conditions.
In this experiment, the best condition to reach high growth rate was by using high POME fractions (75%) and produce around 30–40% lipid, which is difficult to reach by monocultivation condition. Previous research found that semi continuous cultivation for Spirulina sp. was able to utilize 100% POME without dilution to produce C-PC pigment. However, this mode cultivation is on controlled condition and it is not practical when applied in large scale condition. In the present study, the cultivation of mixed culture could utilize high POME fractions in batch and outdoor environmental condition. This finding could be applied in the large and industrial scale since light and temperature were two main difficult factors to maintain. While external nutrition (such as urea) and salinity were two main nutritional and environmental factors that are easily to control. Nevertheless, further investigation should be done to understand the wastewater parameters before and after used as medium growth of the mixed cultures.
4. Conclusions
The experimental results showed that mixed cultivation of Dunaliella sp, Spirulina sp.,Nannochloropsis sp, and Chaetoceros calciltrans could adapt better when growing under otdoor condition rather than mono cultivation. The highest growth rate (0.35/day) for mixed culture was found on high POME fraction (75%) with salinity 15 PSU and urea addition 450 mg/L. Low POME fraction enhanced lipid accumulation up to 55%. Salinity, urea concentrations, and POME fractions influenced fatty acid profiles. High POME fraction and urea addition enhanced SFA accumulation in the fatty acid profiles. Co-cultivation of microalgae could be alternative strategy for mass scale production in dynamic environmental and nutritional condition.
Notes on Contributions
Muhamad Maulana Azimatun Nur: conceptualization, methodology, investigation, formal analysis, writing—review & editing. Ira Nurhayati Djarot: conceptualization. Peter Boelen: conceptualization, methodology, formal analysis, supervision. Hadiyanto: methodology, supervision. H.J. Heeres: formal analysis, supervision. All authors reviewed the manuscript.
Supplemental Material
Download MS Word (1.8 MB)Acknowledgments
This research is supported by Lembaga Pengelola Dana Pendidikan (LPDP), Ministy of Funds, Republik Indonesia.
Disclosure statement
No potential conflict of interest was reported by the author(s).
Supplementary material
Supplemental data for this article can be accessed online at https://doi.org/10.1080/26395940.2022.2147098
Additional information
Funding
References
- Yusoff MHM, Ayoub M, Nazir MH, et al. Comprehensive review on biodiesel production from palm oil mill effluent. Chem Bio Eng Reviews. 2021;8(5):439–462.
- Farobie O, Hartulistiyoso E. Palm oil biodiesel as a renewable energy resource in indonesia: current status and challenges. Bioenergy Res. 2022;15(1):93–111.
- Uning R, Latif MT, Othman M, et al. A review of Southeast Asian oil palm and Its CO2 fluxes. Sustain. 2020;12(12):5077.
- Saputera WH, Amri AF, Daiyan R, et al. Photocatalytic technology for palm oil mill effluent (POME) wastewater treatment: current progress and future perspective. Materials. 2021;14(11):2846.
- Ahmad AL, Ismail S, Bhatia S. Water recycling from palm oil mill effluent (POME) using membrane technology. Desalination. 2003;157(1–3):87–95.
- Ratnasari A, Zaidi NS, Syafiuddin A, et al. Prospective biodegradation of organic and nitrogenous pollutants from palm oil mill effluent by acidophilic bacteria and archaea. Bioresour Technol Rep. 2021;15:1–11.
- Dominic D, Baidurah S. Recent developments in biological processing technology for palm oil mill effluent treatment—A review. Biology (Basel). 2022;11(4):525.
- Nur MMA. Co-production of fucoxanthin and lipid from Indonesian diatom and green algae growing on palm oil mill effluent under mixotrophic condition. Biocat Agric Biotechnol. 2021; 38:102228.
- Nur MMA. Co-production of polyhydroxybutyrate and C-phycocyanin from Arthrospira platensis growing on palm oil mill effluent by employing UV-C irradiation. J Appl Phycol. 2022;34:1389–1396.
- Nur MMA, Swaminathan MK, Boelen P, et al. Sulfated exopolysaccharide production and nutrient removal by the marine diatom Phaeodactylum tricornutum growing on palm oil mill effluent. J Appl Phycol. 2019;31(4):2335–2348.
- Nur MMA, Buma AG. Opportunities and challenges of microalgal cultivation on wastewater, with special focus on palm oil mill effluent and the production of high value compounds. Waste Biomass Valorization. 2019;10(8):2079–2097.
- Acién G LT, Acién G. Microalgae for the food industry: from biomass production to the development of functional foods. Foods. 2022;11(5):765.
- Sirohi R, Choi HI, Sim SJ. Microalgal fuels: promising energy reserves for the future. Fuel. 2022;312:1–11.
- Rizwan M, Mujtaba G, Memon SA, et al. Exploring the potential of microalgae for new biotechnology applications and beyond: a review. Renew Sust Energ Rev. 2018;92:394–404.
- Bhatia SK, Mehariya S, Bhatia RK, et al. Wastewater based microalgal biorefinery for bioenergy production: progress and challenges. Sci Total Environ. 2021;751:141599.
- Palanisamy KM, Maniam GP, Sulaiman AZ, et al. Palm oil mill effluent for lipid production by the diatom Thalassiosira pseudonana. Fermentation. 2022;8(1):23.
- Resdi R, Lim JS, Idris A. Batch kinetics of nutrients removal for palm oil mill effluent and recovery of lipid by Nannochloropsis sp. J Water Process Eng. 2021;40:101767.
- Kamyab H, Chelliapan S, Lee CT, et al. Improved production of lipid contents by cultivating Chlorella pyrenoidosa in heterogeneous organic substrates. Clean Technol Envir. 2019;21(10):1969–1978.
- Vairappan CS, Yen AM. Palm oil mill effluent (POME) cultured marine microalgae as supplementary diet for rotifer culture. J Appl Phycol. 2008;20(5):603–608.
- Gupta S, Pawar SB, Pandey RA. Current practices and challenges in using microalgae for treatment of nutrient rich wastewater from agro-based industries. Sci Total Environ. 2019;687:687 1107–1126.
- Pessi BA, Pruvost E, Talec A, et al. Does temperature shift justify microalgae production under greenhouse? Algal Res. 2022;61:1–10.
- Ray A, Nayak M, Ghosh A. A review on co-culturing of microalgae: a greener strategy towards sustainable biofuels production. Sci Total Environ. 2022;802:149765.
- Maryjoseph S, Ketheesan B. Microalgae based wastewater treatment for the removal of emerging contaminants: a review of challenges and opportunities. Case Studies Chem Enviro Eng. 2020;2:1–10.
- Nie X, Mubashar M, Zhang S, et al. Current progress, challenges and perspectives in microalgae-based nutrient removal for aquaculture waste: a comprehensive review. J Clean Prod. 2020;277:124209.
- Caroppo C, Pagliara P. Microalgae: a Promising Future. Microorganisms. 2022;10(8):1488.
- Chu R, Li S, Zhu L, et al. A review on co-cultivation of microalgae with filamentous fungi: efficient harvesting, wastewater treatment and biofuel production. Renewable Sustainable Energy Rev. 2021;139:110689.
- Das PK, Rani J, Rawat S, et al. Microalgal co-cultivation for biofuel production and bioremediation: current status and benefits. Bioenergy Res. 2022;15(1):1–26.
- Arutselvan C, Narchonai G, Pugazhendhi A, et al. Evaluation of microalgal strains and microalgal consortium for higher lipid productivity and rich fatty acid profile towards sustainable biodiesel production. Bioresour Technol. 2021;339:125524.
- Azarpour A, Zendehboudi S, Mohammadzadeh O, et al. A review on microalgal biomass and biodiesel production through Co-cultivation strategy. Energy Convers Manag. 2022;267:1–35.
- Nur MM, Garcia GM, Boelen P, et al. Enhancement of C-phycocyanin productivity by Arthrospira platensis when growing on palm oil mill effluent in a two-stage semi-continuous cultivation mode. J Appl Phycol. 2019;31(5):2855–2867.
- Tompkins J, de Ville MM, Day JG, et al. Culture Collection of Algae & Protozoa: Catalogue of Strains. Kendal, UK: Titus Wilson and Son Ltd.; 1995.
- Guillard RR. Culture of phytoplankton for feeding marine invertebrates. In: Smith WL, Chanley MH, editors. Culture of marine invertebrate animals. Boston MA: Springer; 1975. p. 29–60.
- Zarrouk C (1966) Contribution a l’etude d’une cyanobacterie: influence de divers facteurs physiques et chimiques sur la croissance et la photosynthese de Spirulina maxima (Setchell et Gardner) Geitler. PhD thesis, University of Paris, France.
- Nur MM, Garcia GM, Boelen P, et al. Influence of photodegradation on the removal of color and phenolic compounds from palm oil mill effluent by Arthrospira platensis. J Appl Phycol. 2021;33(2):901–915.
- Axelsson M, Gentili F, Kusano M. A single-step method for rapid extraction of total lipids from green microalgae. PloS one. 2014;9(2):e89643.
- Boelen P, Van Mastrigt A, Van De Bovenkamp HH, et al. Growth phase significantly decreases the DHA-to-EPA ratio in marine microalgae. Aquac Int. 2017;25(2):577–587.
- Teoh ML, Phang SM, Chu WL. Response of Antarctic, temperate, and tropical microalgae to temperature stress. J Appl Phycol. 2013;25(1):285–297.
- Mattsson L, Sörenson E, Capo E, et al. Functional diversity facilitates stability under environmental changes in an outdoor microalgal cultivation system. Front Bioeng Biotechnol. 2021;9. DOI:10.3389/fbioe.2021.651895
- Stirk WA, Bálint P, Maróti G, et al. Comparison of monocultures and a mixed culture of three Chlorellaceae strains to optimize biomass production and biochemical content in microalgae grown in a greenhouse. J Appl Phycol. 2021;33(5):2755–2766.
- Zhao P, Yu X, Li J, et al. Enhancing lipid productivity by co-cultivation of Chlorella sp. U4341 and Monoraphidium sp. FXY-10. J Biosci Bioeng. 2014;118(1):72–77.
- Tejido-Nuñez Y, Aymerich E, Sancho L, et al. Co-cultivation of microalgae in aquaculture water: interactions, growth and nutrient removal efficiency at laboratory-and pilot-scale. Algal Res. 2020;49:1–8.
- Ishika T, Moheimani NR, Bahri PA. Sustainable saline microalgae co-cultivation for biofuel production: a critical review. Renew Sust Energ Rev. 2017;78:356–368.
- Jasni J, Arisht SN, Yasin NHM, et al. Comparative toxicity effect of organic and inorganic substances in palm oil mill effluent (POME) using native microalgae species. J Water Process Eng. 2020;34:101165.
- Sabilil MS, Agus SE. Biomass Composition of Microalgae Local Mixed Culture using POME (Palm Oil Mill Effluent) Medium. Res J Biotechnol. 2021;16:5.
- Luo Y, Le-Clech P, Henderson RK. Characterisation of microalgae-based monocultures and mixed cultures for biomass production and wastewater treatment. Algal Res. 2020;49:1–10.
- Shi TQ, Wang LR, Zhang ZX, et al. Stresses as first-line tools for enhancing lipid and carotenoid production in microalgae. Front Bioeng Biotechnol. 2020;8:610.
- Shigetomi Y, Ishimura Y, Yamamoto Y. Trends in global dependency on the Indonesian palm oil and resultant environmental impacts. Sci Rep. 2020;10(1):1–11.
- Qu Z, Duan P, Cao X, et al. Comparison of monoculture and mixed culture (Scenedesmus obliquus and wild algae) for C, N, and P removal and lipid production. Environ Sci Pollut Res. 2019;26(20):20961–20968.
- Sivaramakrishnan R, Suresh S, Pugazhendhi A, et al. Response of Scenedesmus sp. To microwave treatment: enhancement of lipid, exopolysaccharide and biomass production. Bioresour Technol. 2020;312:123562.
- Feng Y, Xiao J, Cui N, et al. Enhancement of Lipid Productivity and Self-flocculation by Cocultivating Monoraphidium sp. FXY-10 and Heveochlorella sp. Yu Under Mixotrophic Mode. Appl Biochem Biotechnol. 2021;193(10):3173–3186.
- Vyas S, Patel A, Risse EN, et al. Biosynthesis of microalgal lipids, proteins, lutein, and carbohydrates using fish farming wastewater and forest biomass under photoautotrophic and heterotrophic cultivation. Bioresour Technol. 2022;359:127494.
- Montero E, Olguín EJ, De Philippis R, et al. Mixotrophic cultivation of Chlorococcum sp. Under non-controlled conditions using a digestate from pig manure within a biorefinery. J Appl Phycol. 2018;30(5):2847–2857.
- Rashid N, Ryu AJ, Jeong KJ, et al. Co-cultivation of two freshwater microalgae species to improve biomass productivity and biodiesel production. Energy Convers Manag. 2019;196:640–648.
- Krzemińska I, Oleszek M. Glucose supplementation-induced changes in the Auxenochlorella protothecoides fatty acid composition suitable for biodiesel production. Biores Technol. 2016;218:1294–1297.
- Zheng Y Yu L Chen S LT, Zheng Y, Yu L. Mixotrophic cultivation of a Chlorella sorokiniana strain for enhanced biomass and lipid production. Biomass Bioener. 2014; 66:204–213.
- Huang J Sun Z Zhong Y Jiang Y Chen F LJ, Huang J, Sun Z. Differential lipid and fatty acid profiles of photoautotrophic and heterotrophic Chlorella zofingiensis: assessment of algal oils for biodiesel production. Bioresour Technol. 2011;102(1):106–110.
- Guihéneuf F, Stengel DB. LC-PUFA-enriched oil production by microalgae: accumulation of lipid and triacylglycerols containing n-3 LC-PUFA is triggered by nitrogen limitation and inorganic carbon availability in the marine haptophyte Pavlova lutheri. Mar Drugs. 2013;11(11):4246–4266.
- Liu W, Huang Z, Li P, et al. Formation of triacylglycerol in Nitzschia closterium f. Minutissima under nitrogen limitation and possible physiological and biochemical mechanisms. J Exp Mar Biol Ecol. 2012;418:24–29.
- Zarrinmehr MJ, Farhadian O, Heyrati FP, et al. Effect of nitrogen concentration on the growth rate and biochemical composition of the microalga, Isochrysis galbana. Egyptian Jaquatic Res. 2020;46(2):153–158.
- Mohsenpour SF, Richards B, Willoughby N. Spectral conversion of light for enhanced microalgae growth rates and photosynthetic pigment production. Bioresour Technol. 2012;125:75–81.
- Kamyab H, Din MFM, Keyvanfar A, et al. Efficiency of microalgae Chlamydomonas on the removal of pollutants from palm oil mill effluent (POME). Energy Procedia. 2015;75:2400–2408.
- Shah SMU, Ahmad A, Othman MF, et al. Effects of palm oil mill effluent media on cell growth and lipid content of Nannochloropsis oculata and Tetraselmis suecica. Int J Green Energy. 2016;13(2):200–207.
- Cheah WY, Show PL, Yap YJ, et al. Enhancing microalga Chlorella sorokiniana CY-1 biomass and lipid production in palm oil mill effluent (POME) using novel-designed photobioreactor. Bioengineered. 2020;11(1):61–69.
- Cheah WY, Show PL, Juan JC, et al. Microalgae cultivation in palm oil mill effluent (POME) for lipid production and pollutants removal. Energy Convers Manag. 2018a;174:430–438.
- Cheah WY, Show PL, Juan JC, et al. Enhancing biomass and lipid productions of microalgae in palm oil mill effluent using carbon and nutrient supplementation. Energy Convers Manag. 2018b;164:188–197.