Abstract
The enzyme α-isopropylmalate synthase (α-IPMS) catalyzes the first step of L-leucine biosynthesis and is regulated via feedback inhibition by L-leucine. The gene of α-IPMS variant from strain ARTP-L04 was cloned and sequenced. Interestingly, amino acid mutations of Gly92Asp, Ile162Val, Arg494His and Gly526Asp occurred in the α-IPMS variant. The enzyme of α-IPMS variant was characterized, and exhibited higher resistance to feedback inhibition by L-leucine. In the presence of 20 mM L-leucine, the activity of the α-IPMS variant was still retained at over 50%. The α-IPMS variant almost removed feedback inhibition by L-leucine, while there was no significant difference in specific activities of the α-IPMS variant and wild-type α-IPMS. For the α-IPMS variant, the capacity to overproduce L-leucine was evaluated by recombinant Corynebacterium glutamicum strains. Interestingly, expression of the α-IPMS variant could significantly enhance L-leucine production, especially co-expressed with acetohydroxyacid synthase (accumulating 7.79 g/L L-leucine). Collectively, our findings provided valuable insights into further development in genetic engineering for L-leucine production.
1. Introduction
Corynebacterium glutamicum has become a platform organism widely used in industry for commercially exciting compounds, such as amino acids and organic acids (Vogt et al. Citation2014; Burkovski Citation2008; Elišáková et al. Citation2005). C. glutamicum can also produce L-leucine, which is widely used as a dietary product, the component of cosmetics, pharmaceuticals, and herbicide synthesis (Ikeda Citation2003; Park and Lee Citation2010; Vogt et al. Citation2015). For example, L-leucine can improve the nutritional status of critically ill patients, prevent premature muscle fatigue, and promote skeletal muscle regeneration (Vogt et al. Citation2014; Freund et al. Citation1982; Daniel et al. Citation2015; Calder Citation2006).
As can be seen from Figure , α-isopropylmalate synthase (α-IMPS, EC 2.3.3.13) catalyzes the reaction between α-ketoisovalerate (α-KIV) and acetyl coenzyme A (AcCoA) in the first step of L-leucine biosynthesis (Ayako et al. Citation2018). L-leucine is finally synthesized by the following enzymes of 3-isopropylmalate dehydratase (IPMI), 3-isopropylmalate dehydrogenase (IPMD) and aminotransferase (TA). The precursors of L-leucine, L-isoleucine, and L-valine are initially synthesized by acetohydroxyacid synthase (AHAS, EC 4.1.3.8). Therefore, although it is not specific, AHAS is also vital for L-leucine synthesis.
Figure 1. Biosynthesis of branched chain amino acids and its regulation in C. glutamicum. Feedback regulation by various amino acids is indicated by dashed double arrow. Repression regulation by various amino acids is indicated by solid double arrow.
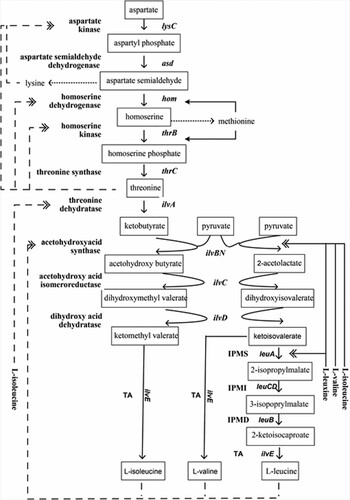
L-leucine has disadvantages in yield during multivalent regulation and long synthesis pathways (Vogt et al. Citation2015; Becker et al. Citation2011; Nakamura et al. Citation2007). Therefore, a few studies on L-leucine production have been performed by metabolic engineering (Tsuchida and Momose Citation1975; Tsuchida et al. Citation1974; Gusyatiner et al. Citation2004; Katashkina et al. Citation2006; Vot et al. 2013). However, the productivity of these strains is not as well as that of L-lysine and L-glutamate (Vogt et al. Citation2015). Therefore, efficient production processes are strongly in demand.
As a key enzyme in L-leucine synthesis, α-IMPS has been investigated in a few microorganisms, notably C. glutamicum, Salmonella typhimurium, and Saccharomyces cerevisiae (Kohlhaw Citation1988; Kohlhaw and Leary Citation1969; Nayden et al. Citation2004; Pátek et al. Citation1994). It has been found there are significant sequence similarities among bacteria, fungi, and plants. Therefore, this enzyme is highly conserved. α-IPMS is subject to feedback inhibition by L-leucine, showing 50% activity in the presence of 0.4 mM L-leucine (Wandee et al. Citation2009; Aguirre-López et al. Citation2020; Vigueras-Meneses et al. Citation2022). In Mycobacterium tuberculosis, the N-terminal domain contains the active site, and the C-terminal domain contains the L-leucine binding site of the enzyme (Kulwant and Vinod Citation2007). There is an N-terminal TIM barrel catalytic domain (residues 51–368), a subdivided linker domain (residues 369–424 and 434–490), and a C-terminal regulatory domain (residues 491–644) (Patrick Citation2012). Interestingly, mutations of T482I, E386 K, G479C, and G462D can decrease feedback inhibition, and the IC50 for L-leucine is greater than 10 mM in Escherichia coli (Mikhail et al. Citation2002). In C. glutamicum, mutations of R529H and G532D with other rational design could cause high productivity of 4.3 mmol/L/h (Vogt M et al. Citation2013).
It is often challenging to further improve the performance of random mutant strains. Therefore, systems metabolic engineering has been proposed to make efficient production processes (Lee et al. Citation2005; Park and Lee Citation2008). In the present study, we aimed to characterize the α-IPMS variant from ARTP-L04 (L-leucine-producing strain) and evaluated its capacity to overproduce L-leucine in C. glutamicum. This study provided valuable insights into further development in gene engineering for L-leucine production.
2. Materials and methods
Prime STAR HS DNA polymerase, restriction endonucleases, Genomic Extraction Kit, Fragment Purification Kit, Agarose Gel DNA Extraction Kit, and Plasmid Purification Kit were purchased from TaKaRa (Beijing, China). DNA sequencing was performed by Sangon (Shanghai, China). Isopropyl-β-D-l-thiogalactopyranoside (IPTG) was obtained from Sangon (Shanghai, China).
2.1 Strains, plasmids, primers, and medium
The bacterial strains, plasmids, and primers used in this work are listed in Table .
Table 1. Strains, plasmids and primers used in this study.
Strain ARTP-L04 was kept in our lab, and could accumulate 18 g/L L-leucine. It was screened from strain ATCC 13032 by Atmospheric Room Temperature Plasma Mutagenesis (ARTP). E. coli DH5α was used as the host cells for propagating plasmids, and E. coli BL21 (DE3) was used as the host cells for protein expression. The E. coli strain was cultivated in Luria–Bertani (LB) medium at 37°C with vibration at 200 rpm. The C. glutamicum strain was grown in LBG medium (LB containing 5 g/L glucose) at 30°C. When appropriate, kanamycin (30 µg/mL for C. glutamicum, 50 µg/mL for E. coli) and 0.5 mM IPTG were added to the culture medium.
LBG medium was used as a seed culture medium. The fermentation medium was composed of 90 g/L glucose, 35 g/L (NH4)2SO4, 20 g/L corn steep liquor, 1 g/L KH2PO4, 0.5 g/L MgSO4·7H2O, 10 g/L CaCO3, 50 µg/L D-biotin, and 100 µg/L thiamine-HCl. D-biotin and thiamine-HCl were filter-sterilized and aseptically added to the medium. The medium component of CaCO3 was sterilized alone by dry heat sterilization at 160°C for 90 min before being added to the medium. The pH of both media was adjusted to pH 7.5 using KOH.
2.2 Constructions of plasmids
Routine methods of molecular cloning (PCR, DNA restriction, and ligation) were carried out as previously described (Sambrook et al. Citation1989). EcoR I and Xho I restriction sites and protective bases were introduced into primers of leuA-F1 and leuA-R1, respectively. The wild-type leuA gene was amplified with primers of leuA-F1 and leuA-R1 by PCR using genomic DNA of strain ATCC 13032 as a template. With the same primers, the leuAV gene was obtained using genomic DNA of strain ARTP-L04 as a template. These two fragments were cleaved with EcoR I and Xho I and then ligated into vector pET28a, resulting in recombinant plasmids pET28a-leuA and pET28a-leuAV, respectively. Next, the gene of ilvBNV was amplified with primers of ilvBN-F and ilvBN-R by PCR using genomic DNA of strain ARTP-L04 as a template. Subsequently, the fragment ilvBNV was ligated into vector pUC19, resulting in recombinant plasmid pUC19-ilvBNV. All resulting plasmids were sequenced for identification.
Using genomic DNA of strain ARTP-L04 as a template, the leuAV gene was obtained with primers of leuA-F2 (containing Xba I site) and leuA-R2 (containing EcoR I site) and subsequently ligated into vector pXMJ19 (Jakoby et al. Citation1999), resulting in plasmid pXMJ19-leuAV. With the same primers, plasmid pXMJ19-leuA was also obtained using the genomic DNA of strain ATCC 13032 as a template. Meanwhile, ilvBNV gene was amplified with primers of ilvBN-F and ilvBN-R using genomic DNA of strain ARTP-L04 as template. After digestion with Hind III and Xba I, the final PCR product was ligated into vector pXMJ-19 (similarly digested), resulting in recombinant plasmid pXMJ19-ilvBNV (Table ). With Xba I and EcoR I, the fragment leuAV was digested and subsequently ligated into plasmid pXMJ19-ilvBNV (similarly digested), resulting in plasmid pXMJ19-ilvBNV-leuAV (Table ). All resulting plasmids were sequenced for identification.
2.3 Constructions of recombinant strains
For protein (α-IMPS) purification, plasmids of pET28a-leuAV and pET28a-leuA were transformed into E. coli BL21 (DE3), resulting in recombinant E. coli strains (Table ). To verify the effect on L-leucine synthesis, plasmids pXMJ19-leuAV, pXMJ19-leuA, and pXMJ19-ilvBNV-leuAV were transformed into C. glutamicum ATCC 13032 by electrotransformation method (Tan et al. Citation2012), resulting in recombinant C. glutamicum strains (Table ).
2.4 Protein expression and purification
Recombinant E. coli strains were grown in LB medium at 37°C till an OD600 of 0.6 was achieved, followed by induction using 0.5 mM IPTG. After 4 h of induction, cells were harvested by centrifugation at 6,000×g for 10 min at 4°C. Cells were disrupted by sonication in buffer A (0.5 M NaCl, 10 mM imidazole, and 20 mM HEPES, pH 8.0), and then the crude extract was obtained. The pET28a vector endows α-IPMS protein with an N-terminal His-tag. Therefore, the α-IPMS protein was purified by the Ni-NTA affinity column under buffer B (0.5 mM NaCl, 0.5 mM imidazole, and 20 mM HEPES, pH 8.0). The recombinant protein was then eluted with 0.5 mL elution buffer (50 mM NaH2PO4, 300 mM NaCl, 250 mM imidazole, pH 8.0) and then dialyzed against 50 mM phosphate buffer (pH 7.0). The purified protein was stored in 50% glycerol at −70°C.
2.5 Enzyme assay
The α-IPMS activity was analyzed using 5,5'-dithio-bis (2-nitrobenzoic acid) (DTNB) to detect the formation of coenzyme A (CoA) at a wavelength of 412 nm (Kohlhaw and Leary Citation1969; Wandee et al. Citation2009). The enzyme assay was performed in 150 µL buffer containing 50 µM Tris-HCl (pH 8.5), 20 µM KCl, 0.2 µM acetyl CoA, and 0.5 µM α-ketoisovaleric acid. The reaction was initiated by adding 100 µL purified proteins to 150 µL reaction buffer, followed by incubation at 37°C for 5 min. The reaction was stopped by adding 0.75 mL absolute ethanol and 0.5 mL 1 mM DTNB.
Feedback inhibition was determined at different concentrations of L-leucine. The enzyme kinetics curve was obtained by altering L-leucine concentrations. L-leucine was added to reaction mixtures at various final concentrations (0.1, 0.2, 0.3, 0.4, 0.5, 0.8, 1.0, 2.0, 3.0, 4.0, 5.0, 10, and 20 mM).
Enzyme activity was defined as units (of enzyme) per milligram of protein. One unit of the enzyme was defined as the amount catalyzing the formation of 1 µM CoA per minute. Protein concentrations were determined as previously described (Bradford Citation1976). Relevant standards and controls were treated in the same manner.
2.6 Fermentation
Strains of ATCC 13032/leuA, ATCC 13032/leuAV, ATCC 13032/ilvBNV, and ATCC 13032/ilvBNV-leuAV were grown in culture medium. L-leucine levels of these strains were evaluated. Firstly, strains were inoculated in an LBG medium by transferring single colonies from LBG plates, followed by incubation at 30°C and 200 rpm. After growing to the exponential phase (14-16 h), the seed culture was inoculated into a 500-mL flask containing 30 mL fermentation medium, and 0.5 mM IPTG was added to the medium. The resulting culture was incubated at 30°C for 72 h at 200 rpm.
2.7 Statistical analysis
All of the experiments were independently performed at least three times, and data were represented as mean and standard deviation (±SD). Student’s test was used to compare the statistical difference among experimental groups.
2.8 Analytical methods
Samples were collected and centrifuged at 1, 2000×g for 10 min at 4°C. Proteins were purified from the supernatant by trichloroacetic acid (30 g/L) and filtered through a membrane (pore size = 0.22 µm). Amino acids were pre-column derivatized by o-phthaldialdehyde (OPA). The assay was performed in 200 µL 0.1 M sodium borate buffer (pH 9.3) containing 0.8 mM OPA and 4.5 mM 3-mercaptopropionic acid (3-MPA). The sodium borate buffer was stored away from light for 90 min. Subsequently, 800 µL sample was added to the sodium borate buffer and then subjected to light-free derivatization for 15 min. Amino acids were analyzed on an UltiMate 3000 UHPLC system (Thermo Technologies, USA) equipped with a reverse-phase column (Zorbax Eclipse-AAA) and a UV detector at 338 nm according to the published procedure (Zhang et al. Citation2014; Zhang et al. Citation2013).
3. Results
3.1 Cloning and sequencing of α-IPMS variant and AHAS variant from strain ARTP-L04
It is known that α-IPMS is specific for L-leucine synthesis and is strongly inhibited by the end product (L-leucine). In C. glutamicum, α-IPMS is encoded by leuA. Therefore, genes of leuA were obtained from wild-type C. glutamicum and strain ARTP-L04, respectively. Sequence alignment of the α-IPMS variant and wild-type α-IPMS was performed. We found that there were mutations in the α-IPMS variant from strain ARTP-L04. As seen from Figure , mutations of Gly92Asp, Ile162Val, Arg494His and Gly526Asp occurred in the α-IPMS variant. Similarly, amino acid exchanges also occurred in the AHAS variant. In addition, it was observed that mutations of A226S, V233I and Y252H occurred in the large subunit, and mutations of S41 V and G156 V occurred in the small subunit.
3.2 Characterization of α-IPMS variant and wild-type α-IPMS
α-IPMS is the crucial enzyme in the L-leucine synthesis pathway, which is inhibited by the end product. In order to determine the specific activity of α-IPMS, strain BL21 (DE3)/leuAV and strain BL21 (DE3)/leuA were constructed, respectively. Crude extracts of these two strains were prepared, and proteins were purified. The protein band around 66 kDa was observed (Figure ), indicating that the α-IPMS variant and α-IPMS were well expressed in E. coli BL21 (DE3).
Figure 2. SDS-PAGE analysis of α-IPMS variant and wild-type α-IPMS. Lane M, molecular markers; lane CK, cell lysates of BL21 (DE3); lane C1, cell lysates of BL21 (DE3)/leuA; lane P1, purified protein of wild-type α-IPMS; lane C2, cell lysates of BL21 (DE3)/leuAV; lane P2, purified protein of α-IPMS variant.
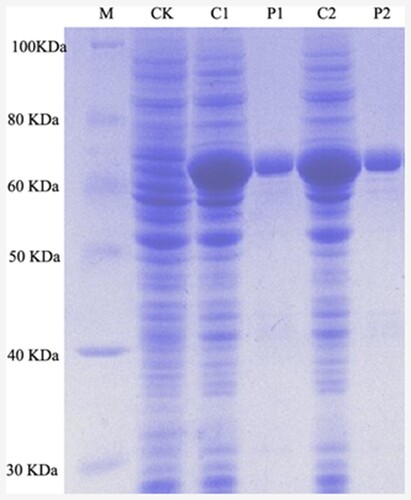
The specific activities of the α-IPMS variant and wild-type α-IPMS were determined (Table ). We found that mutations caused no-to-minor changes in the specific activity. For 2-ketoisovalerate, wild-type α-IPMS and α-IPMS variant exhibited similar affinity. Similarly, for acetyl-CoA, there was no significant difference in affinity between wild-type α-IPMS and α-IPMS variant.
Table 2. Characterization of α-IPMS variant and wild-type α-IPMS.
However, dramatic differences in the activities of these two enzymes were observed in the presence of L-leucine. As shown in Figure (b), the activity of wild-type α-IPMS was inhibited by 58.7% in the presence of 0.5 mM L-leucine. Interestingly, the α-IPMS variant provided 96% retention of the enzyme activity, in the presence of 0.5 mM L-leucine (Figure (a)). Therefore, the α-IPMS variant exhibited higher resistance to feedback inhibition than wild-type α-IPMS. The specific activity of the α-IPMS variant was decreased more slowly in the presence of L-leucine. In the presence of 20 mM L-leucine, the activity of the α-IPMS variant was retained at over 50%. Therefore, the α-IPMS variant almost eliminated the feedback inhibition by L-leucine.
3.3 Application of α-IPMS variant for L-leucine synthesis
To evaluate the capacity of the α-IPMS variant for L-leucine production, strains of ATCC 13032/leuA, ATCC 13032/leuAV, ATCC 13032/ilvBNV, and ATCC 13032/ilvBNV-leuAV were further constructed and cultivated for 72 h to evaluate L-leucine production. In contrast, strain ATCC 13032 was used as the control group. In fact, strain ATCC 13032 accumulated little L-leucine. On the other hand, the expression of α-IPMS could enhance L-leucine production. As shown in Figure , the L-leucine titer of strain ATCC 13032/leuA was 0.72 g/L. Strains of ATCC 13032/ilvBNV, ATCC 13032/leuAV, and ATCC 13032/ilvBNV-leuAV produced 0.40, 2.34, and 7.80 g/L L-leucine, respectively. Compared with strain ATCC 13032/leuA, L-leucine titers of strain ATCC 13032/leuAV and strain ATCC 13032/ilvBNV-leuAV were increased by 3.25 and 10.8 folds, respectively. These results further indicated that mutations that occurred in the α-IPMS variant played a crucial role in the feedback inhibition by L-leucine.
4. Discussion
In the present study, the α-IMPS variant from strain ARTP-L04 was cloned and characterized. Since strain ARTP-L04 could accumulate 18 g/L L-leucine, feedback inhibition of the α-IMPS variant was further investigated in detail. The capacity of the α-IMPS variant for L-leucine production was finally evaluated by recombinant C. glutamicum strains, and the result was positive.
The enzyme α-IMPS, encoded by leuA, catalyzes the reaction between α-KIV and AcCoA in the first step of L-leucine biosynthesis. This key enzyme is regulated by attenuation and is subject to allosteric feedback inhibition by its end product (L-leucine) (Nayden et al. Citation2004). In the presence of 0.4 mM L-leucine, the α-IMPS activity is reduced by 50%, leading to very deficient L-leucine production in C. glutamicum (Pátek et al. Citation1994; Michael et al. Citation2014). Therefore, α-IPMS is specific for L-leucine synthesis in C. glutamicum, which can be the target for genetic engineering. Consequently, purification and characterization of the α-IPMS variant were the main focus of this study. Moreover, an evaluation of the α-IMPS variant for L-leucine production was performed.
It is well known that amino acid analogs are artificial analogs of natural amino acids that can be used for crosslinking protein complexes (Suchanek et al. Citation2005). Therefore, chemical mutagenesis is widely used for breeding L-leucine-producing strains by screening of analog-resistant mutants. These mutants are commonly resistant to 2-azaleucine, 3-hydroxyleucine, or nitroso-guanidin (NTG), which may possess α-IPMS and AHAS resistance to inhibition by the end products (Tsuchida and Momose Citation1975; Tsuchida and Momose Citation1986). To obtain branch carbon flux from L-valine synthesis, the performance of L-leucine producers can also be improved by genetic manipulation of leu (particularly leu A) genes in the L-valine strain (Hutson et al. Citation2013; Pátek Citation2007). Therefore, α-IPMS (encoded by leu A) can be modified to reduce sensibility to feedback inhibition by random mutation or genetic engineering. Traditionally, L-leucine-producing strains are developed by chemical mutagenesis. Strain ARTP-L04 is developed to produce 18 g/L L-leucine by this traditional method efficiently. It is inferred that the specific enzyme for L-leucine synthesis would have some mutations at a certain point. In the present study, mutations of Gly92Asp, Ile162Val, Arg494His, and Gly526Asp occurred in the α-IPMS variant from strain ARTP-L04. High specific activity or resistance to feedback inhibition is necessary for the high performance of the L-leucine-producing strain. Interestingly, the α-IPMS variant from strain ARTP-L04 exhibited higher activity than wild-type α-IPMS in the presence of L-leucine (Figure ). In the presence of 0.5 mM L-leucine, the activity of the wild-type α-IPMS was nearly reduced by 60%, which was consistent with the reported data (Pátek et al. Citation1994; Wandee et al. Citation2009; Michael et al. Citation2014). On the contrary, the activity of the α-IPMS variant retained at over 95% in the presence of 0.5 mM L-leucine. Furthermore, the α-IPMS variant could exhibit 87% activity even in the presence of 20 mM L-leucine. The performance of α-IPMS variant might be attributed to the four mutations of Gly92Asp, Ile162Val, Arg494His, and Gly526Asp in this variant. It is known that L-leucine-binding sites are located in the C-domain of α-IPMS, especially at the end of the C-domain (Ayako et al. Citation2018). It has been reported that mutations of R529H and G532D can mostly remove feedback inhibition by L-leucine, and mutations with other rational designs can produce 24 g/L L-leucine, in C. glutamicum (Michael et al. Citation2014). It is also found that mutations of Thr482Ile, Glu386Lys, Pro428Leu, Gly479Cys, and Gly462Asp can decrease feedback inhibition to different degrees (Stieglitz and Calvo Citation1974). However, mutations of Gly92Asp, Ile162Val, Arg494His and Gly526Asp occurring in the α-IPMS variant were novel residue sites in the C-domain. In this work, the α-IPMS variant almost removed feedback inhibition by L-leucine, which might be attributed to Arg494His and Gly526Asp occurring in the C-domain of α-IPMS. However, there were no dramatic differences in the specific activity between wild-type α-IPMS and the α-IPMS variant (Table ). For Km 2-ketoisovalerate and Km acetyl-CoA, the α-IPMS variant was almost the same as wild-type α-IPMS. This finding could infer that mutations of Arg494His and Gly526Asp were responsible for the L-leucine binding site, and mutations of Gly92Asp and Ile162Val had insignificance. Obviously, mutations of Gly92Asp, Ile162Val, Arg494His, and Gly526Asp in the α-IPMS variant might only play a role in the feedback resistance of the holoenzyme. However, this α-IPMS variant could also be preferable for L-leucine synthesis.
Figure reveals that the pathway of L-leucine synthesis was long and complex, and carbon flux to L-leucine was more complicated than L-valine and L-isoleucine. Therefore, an effective strategy for L-leucine production was to draw ketoisovalerate flux towards 2-isopropylmalate. In the present work, the α-IPMS variant exhibited high performance in anti-feedback inhibition. By genetic engineering, recombinant C. glutamicum strains were further constructed to evaluate the capacity of the α-IPMS variant for L-leucine production. At the end of shake flask fermentation, L-leucine production of strain ATCC 13032/leuA was much less than strain ATCC 13032/leuAV since the α-IPMS variant was insensitive to feedback inhibition (Figure (a,c)). Compared with wild-type α-IPMS, the α-IPMS variant exhibited higher resistance to feedback inhibition in the presence of L-leucine. These results further indicated that the α-IPMS variant could significantly enhance L-leucine synthesis.
L-leucine producers may be improved in L-valine-producing strains, which might be due to its synthesis branching from the L-valine synthesis pathway (Figure ) (Hutson et al. Citation2013; Pátek Citation2007). Since acetohydroxy butyrate (a precursor of L-isoleucine) and 2-acetolactate (a precursor of L-valine and L-leucine) are formed similarly by AHAS, it controls carbon flux through this branch point. It determines the relative rates of L-isoleucine, L-valine, and L-leucine synthesis, respectively. Therefore, AHAS also plays a vital role in L-leucine synthesis, and an increase in AHAS activity is necessary to develop L-leucine producers (Michael et al. Citation2014; Leyval et al. Citation2003). However, the over-expression of the AHAS variant from strain ARTP-L04 could not significantly enhance L-leucine production. Only 0.43 g/L L-leucine was accumulated (Figure (b)). This result might be attributed to the fact that α-IPMS was inhibited by L-leucine, causing ketoisovalerate flux to L-valine synthesis. Hence, the α-IPMS and AHAS variants were co-expressed by plasmid pXMJ19 to enhance carbon flux to L-leucine, in C. glutamicum. The resulting transformant gave a relatively high production of L-leucine (7.8 g/L). However, the production was not as high as that of strain ARTP-L04 (18 g/L), which possessed only a single copy of each gene. This finding suggested that many unidentified mutations introduced by random mutagenesis might be effective for L-leucine overproduction, not only the point of α-IPMS and AHAS. AHAS catalyzed mostly ketoisovalerate flux to L-valine synthesis, while the α-IPMS variant converted ketoisovalerate to L-leucine titer. Therefore, the α-IPMS and AHAS variants were also important for the overproduction. In this work, strain ATCC 13032/ilvBNV accumulated 2.53 g/L L-valine, and strain ATCC 13032/ilvBNV-leuAV accumulated 7.8 g/L L-leucine (Table ). Therefore, AHAS synthesized the precursor of L-valine and L-leucine, and then the α-IPMS variant efficiently converted the precursor to the pathway of L-leucine synthesis. Furthermore, a high titer of L-leucine might be achieved by enhancing the supply of pyruvic acid and reducing the synthesis of L-methionine, L-arginine, and L-lysine.
Table 3. Production of L-leucine and L-valine in different recombination strains.
5. Conclusions
It is difficult to further develop high-performance L-leucine-producing strains due to unknown alterations in traditional strains. Therefore, analysis of critical enzymes in L-leucine synthesis is vital to improve L-leucine production. In this study, the gene of leuA (encoding the α-IPMS variant) from strain ARTP-L04 was cloned and analyzed. As a result, amino acid exchanges of Gly92Asp, Ile162Val, Arg494His and Gly526Asp occurred in the α-IPMS variant, leading to almost elimination of the feedback inhibition by the end product (L-leucine). Furthermore, the expression of the α-IPMS variant could significantly enhance L-leucine production, especially when it was co-expressed with the AHAS variant. Collectively, our current findings provided valuable insights into further development in gene engineering.
Author contributions
Methodology, Y.-F.G. and Y.-L. W.; investigation, Y.-F.G., Y. L. and. L. S.; data curation, L. S. and J.-H. L.; writing-original draft preparation, Y.-F.G. and Y.-L. W; writing-review and editing, G.-Q. M. and J.-H. L.; supervision, G.-Q. M. and Y.-L. W.; project administration, G.-Q. M.; funding acquisition, G.-Q. M. and Y.-L. W. All authors have read and agreed to the published version of the manuscript.
Acknowledgments
This work has been published as a preprint by Research Square (https://www.researchsquare.com/article/rs-1717587/v1). This work was supported by the Qingchuang Science and Technology Support Program of Shandong Provincial College with regard to use of laboratory and equipment.
Disclosure statement
No potential conflict of interest was reported by the author(s).
Data availability statement
The authors confirm that raw data supporting the findings of this study are available at https://www.scidb.cn/anonymous/UXJlWWpp.
Additional information
Funding
References
- Aguirre-López B, Escalera-Fanjul X, Hersch-González J. 2020. In kluyveromyces lactis a pair of paralogous isozymes catalyze the first committed step of leucine biosynthesis in either the mitochondria or the cytosol. Front Microbiol. 11:1843.
- Ayako Y, Saori K, Makoto N. 2018. Characterization of two 2-isopropylmalate synthase homologs from Thermus thermophilus HB27. Biochem Biophys Res Commun. 501:465–470.
- Becker J, Zelder O, Haefner S, Schröder H, Wittmann C. 2011. From zero to hero—Design-based systems metabolic engineering of Corynebacterium glutamicum for l-lysine production. Metab Eng. 13:159–168.
- Bradford MM. 1976. A rapid and sensitive method for the quantitation of microgram quantities of protein utilizing the principle of protein-dye binding. Anal Biochem. 72:248–254.
- Burkovski A. 2008. Molecular mechanisms of nitrogen control in Corynebacteria. Wymondham, UK: Caister Academic Press. pp. 183–201.
- Calder PC. 2006. Branched-chain amino acids and immunity. J Nutr. 136:288S–293S.
- Columbus DA, Fiorotto ML, Davis TA. 2015. Leucine is a major regulator of muscle protein synthesis in neonates. Amino Acids. 47:259–270.
- Elišáková V, Pátek M, Holátko J, Nešvera J, Leyval D, Goergen JL, Delaunay S. 2005. Feedback-resistant acetohydroxy acid synthase increases valine production in Corynebacterium glutamicum. Appl Environ Microbiol. 71(1):207–213.
- Freund H, Dienstag J, Lehrich J, Yoshimura N, Bradford RR, Rosen H, Atamian S, Simmer E, Holroyde J, Fische JE. 1982. Infusion of branched-chain enriched amino acid solution in patients with hepatic encephalopathy. Ann Surg. 196:209–220.
- Gusyatiner MM, Voroshilova EB, Rostova YG, Ivanovskaya LV, Lunts MG, Khourges EM. 2004. Method for producing L-leucine. US Patent. 0091980 A1.
- Hutson SM, Sweatt AJ, LaNoue KF. 2013. Branched-chain amino acids (BCAAs). Encyclopedia Biol Chem. 244–249.
- Ikeda M. 2003. Amino acid production processes. Adv Biochem Eng Biotechnol. 79:1–35.
- Jakoby M, Ngouoto-Nkili CE, Burkovski A. 1999. Construction and application of new Corynebacterium glutamicum vectors. Biotechnol Tech. 13:437–441.
- Katashkina JY, Lunts MG, Doroshenko VG, Fomina SA, Skorokhodova AY, Ivanovskaya LV, Mashko SV. 2006. Method for producing an L-amino acid using a bacterium with an optimized level of gene expression. US Patent. 0063240 A1.
- Kohlhaw GB. 1988. α-isopropylmalate synthase from yeast. Methods Enzymol. 166:414–423.
- Kohlhaw GB, Leary TR. 1969. α-Isopropylmalate synthase from Salmonella typhimurium: purification and properties. J Biol Chem. 244:2218–2225.
- Kulwant S, Vinod B. 2007. Cation induced differential effect on structural and functional properties of Mycobacterium tuberculosis α-isopropylmalate synthase. BMC Struct Biol. 7:39.
- Lee SY, Lee DY, Kim TY. 2005. Systems biotechnology for strain improvement. Trends Biotechnol. 23:349–358.
- Leyval D, Uy D, Delaunay S, Goergen JL, Engasser JM. 2003. Characterisation of the enzyme activities involved in the valine biosynthetic pathway in a valine-producing strain of Corynebacterium glutamicum. J Biotechnol. 104:241–252.
- Michael V, Sabine H, Simon K, Tino P, Lothar E, Vo J, Michaelm B. 2014. Pushing product formation to its limit: Metabolic engineering of Corynebacterium glutamicum for L-leucine overproduction. Metab Eng. doi: 10.1016/j.ymben.2013.12.001.
- Mikhail MG, Maria GL, Yuly IK, Lirina VI, Elvira BV. 2002. DNA coding for mutant isopropylmalate -synthase L-leucine-producing microorganism and method for producing L-leucine. United States Patent. US6403342B1.
- Nakamura J, Hirano S, Ito H, Wachi M. 2007. Mutations of the Corynebacterium glutamicum NCgl1221 gene, encoding a mechanosensitive channel homolog, induce L-glutamic acid production. Appl Environ Microbiol. 73:4491–4498.
- Nayden K, Christopher JS, Edward NB. 2004. Crystal structure of LeuA from Mycobacterium tuberculosis, a key enzyme in leucine biosynthesis. PNAS. 101(22):8395–8300.
- Park JH, Lee SY. 2008. Towards systems metabolic engineering of microorganisms for amino acid production. Curr Opin Biotechnol. 19:454–460.
- Park JH, Lee SY. 2010. Fermentative production of branched chain amino acids: a focus on metabolic engineering. Appl Microbiol Biotechnol. 85:491–506.
- Pátek M. 2007. Branched-chain amino acids. Microbiol Monogr. 5:129–162.
- Pátek M, Krumbach K, Eggeling L, Sahm H. 1994. Leucine synthesis in Corynebacterium glutamicum: enzyme activities, structure of leuA, and effect of leuA inactivation on lysine synthesis. Appl Environ Microbiol. 60:133–140.
- Patrick AF. 2012. Structural and functional characterization of α-isopropylmalate synthase and citramalate synthase, members of the LeuA dimer superfamily. Arch Biochem Biophys. 519:202–209.
- Sambrook J, Fritsch E, Maniatis T. 1989. Molecular cloning: a laboratory manual, 2nd ed. Cold Spring Harbor, NY, USA: Cold Spring Harbor Laboratory Press. pp. 23–38.
- Stieglitz BI, Calvo JM. 1974. Distribution of the isopropylmalate pathway to leucine among diverse bacteria. J Bacteriol. 118(3):935–941.
- Suchanek M, Radzikowska A, Thiele C. 2005. Photo-leucine and photo-methionine allow identification of protein-protein interactions in living cells. Nat Methods. 2:261–268.
- Tan Y, Xu D, Li Y, Wang X. 2012. Construction of a novel sacB-based system for marker-free gene deletion in Corynebacterium glutamicum. Plasmid. 67:44–52.
- Tsuchida T, Momose H. 1975. Genetic changes of regulatory mechanisms occurred in leucine and valine producing mutants derived from Brevibacterium lactofermentum. Agric Biol Chem. 39:2193–2198.
- Tsuchida T, Momose H. 1986. Improvement of an L-leucine-producing mutant of Brevibacterium lactofermentum 2256 by genetically desensitizing it to α-acetohydroxy acid synthetase. Appl Environ Microbiol. 51:1024–1027.
- Tsuchida T, Yoshinaga F, Kubota K, Momose H, Okumura S. 1974. Studies on fermentative production of branched-chain amino acids. I. Production of L-leucine by a mutant of Brevibacterium lactofermentum 2256. Agric Biol Chem. 38:1907–1911.
- Vigueras-Meneses LG, Escalera-Fanjul X, El-Hafidi M, Montalvo-Arredondo J, Gómez-Hernández N, Colón M, Granados E, Campero-Basaldua C, Riego-Ruiz L, Scazzocchio C, et al. 2022. Two alpha isopropylmalate synthase isozymes with similar kinetic properties are extant in the yeast Lachancea kluyveri. FEMS Yeast Res. 22:1–10.
- Vogt M, Haas S, Klaffl S, Polen T, Eggeling L, Van O J, Bott M. 2013. Pushing product formation to its limit: metabolic engineering of Corynebacterium glutamicum for L-leucine overproduction. Metab Eng. 22:40–52.
- Vogt M, Haas S, Klaffl S, Polen T, Eggeling L, Van OJ, Bott M. 2014. Pushing product formation to its limit: metabolic engineering of Corynebacterium glutamicum for L-leucine overproduction. Metab Eng. 22:40–52.
- Vogt M, Krumbach K, Bang WG, Van OJ, Noack S, Klein B, Bott M, Eggeling L. 2015. The contest for precursors: channelling L-isoleucine synthesis in Corynebacterium glutamicum without byproduct formation. Appl Microbiol Biotechnol. 99(2):791–800.
- Wandee Y, Supaporn L, Palittapongarnpim P. 2009. Characterization of α-isopropylmalate synthases containing different copy numbers of tandem repeats in Mycobacterium tuberculosis. BMC Microbiol. 9:122.
- Zhang C, Kang Z, Zhang J, Du G, Chen J, Yu X. 2014. Construction and application of novel feedback-resistant 3-deoxy-d-arabino-heptulosonate-7-phosphate synthases by engineering the N-terminal domain for L-phenylalanine synthesis. FEMS Microbiol Lett. 353:11–18.
- Zhang C, Zhang J, Kang Z, Du G, Yu X, Wang T, Chen J. 2013. Enhanced production of L-phenylalanine in Corynebacterium glutamicum due to the introduction of Escherichia coli wild-type gene aroH. J Ind Microbiol Biotechnol. 40:643–651.