ABSTRACT
The gelling ability of whey proteins provides important textural and water holding properties in many foods. However, because many products cannot be heated to the temperature needed for thermal gelation of whey proteins, cold-set gelation of whey proteins could be very advantageous to the food industry. A derivatization procedure for the production of a cold gelling whey protein isolate (WPI) consisting of protein hydration, pH adjustment, thermal gelation, freeze drying, and milling was applied to three commercial whey protein concentrates (WPC). The resulting derivatized WPC powders were reconstituted in water and evaluated through a range of rheological and physical property studies. The effects of temperature, concentration, and shear on viscosity as well as water holding capacity and intrinsic viscosity were assessed. Although the composition of the starting materials influenced the functionality of the final derivatized powders, all samples exhibited a dramatic increase in thickening and water holding ability. All samples were able to form cold-set weak gel structures suitable for contributing viscosity and texture to a wide range of food systems.
INTRODUCTION
The unique functional and nutritional properties of whey proteins make them useful food ingredients for a wide array of applications. The nutritional quality of whey proteins is high, evidenced by a higher biological value and a higher essential amino acid score than egg, milk, and soy proteins.Citation[1] The emulsification, foaming, and gelation properties of whey protein are the basis of its wide use as a functional ingredient in foods.Citation[2] In particular, the ability of whey proteins to form gels capable of holding water, lipids, and other components while providing textural properties is very important to the consumer acceptability of many foods such as processed meat, dairy, and bakery products.Citation[3]
Heat-induced whey protein gels have been extensively studied and reported.Citation4-6 However, in many food applications, heating the product to the high temperatures necessary for heat-induced gelation is undesirable or impossible. Therefore, the induction of gelation at ambient temperatures, termed cold-set gelation, greatly extends the possible utility of whey proteins. Successful cold-set gelation of whey proteins has been described by several authors.Citation7-10 These cold-set gels were prepared by first heating whey protein solutions to achieve unfolding and aggregation, followed by quickly cooling the solutions to room temperature, and then producing gelation with the addition of NaCl or CaCl2.
Cold-gelling whey proteins in a dried form have also been documented. ThomsenCitation[11] described a commercially available modified whey protein concentrate powder with potential applications in foods such as surimi, comminuted meats, dressings, and bakery products. This whey protein texturizer is produced by heat treatment during homogenization of a whey protein concentrate at slightly alkaline pH followed by immediate drying and will gel upon reconstitution in a salt solution.Citation[12] Although the gelling ability of whey proteins plays an important function in many foods, this property can also limit the application in foods where weak or non-gelling characteristics are needed such as beverages, baby-formula, and salad dressings.Citation[13] Hudson et al.Citation[14] developed a derivatized whey protein isolate capable of forming cold-set weak gel structures without the addition of salts or heat. This modification was accomplished through a process consisting of acid hydrolysis, thermal gelation, freezing, freeze-drying, and milling.
The performance of thickening and gelling ingredients is often evaluated with rheological techniques. For viscosifying agents, it is important to not only examine the level of viscosity achieved, but the dependence of this viscosity on conditions that may be commonly experienced by the system. For this reason, thickened systems are often subjected to rotational viscosity measurements over a range of shear rates, concentrations, and temperatures and when applicable, rheological models are developed to describe and predict the flow behavior. Dilute solution viscometry is another valuable tool that is employed to probe the molecular properties of the thickening agent. Measurement of dilute solutions with a capillary viscometer can be done to determine the intrinsic viscosity. Because the intrinsic viscosity is related to the hydrodynamic volume occupied by the molecule, the molecular weight, and the radius of gyration, inferences about important molecular characteristics of the food macromolecule can be made.Citation[15] Foods that achieve functionality by formation of a viscoelastic network are often investigated with small amplitude oscillatory testing. The response of a sample subjected to harmonically varying stress or strain is used to construct a mechanical spectrum that can provide information about the viscous and elastic nature of the sample behavior over a range of frequencies.Citation[16]
Because extensive processing is required to produce a whey protein isolate with a protein content greater than 90%, commercial applications may be cost prohibitive. Therefore, the objectives of this study were to apply the technique of Hudson et al.Citation[14] to several whey protein concentrates, creating more economical cold-set thickening agents, and to characterize the functionality and properties of these ingredients over a wide range of environmental conditions. Solutions of commercial WPC were manipulated to produce heat-induced gels, which upon further processing rendered derivatized protein thickeners, the rheological and physical properties of which are assessed herein.
MATERIALS
Three commercial whey protein concentrate powders were obtained from Davisco Foods International, Inc. (Le Sueur, MN). The compositions of the three WPC samples (lot numbers LE 001-7-685, LE 036-9-280, and LE 012-9-665) are compiled in Table . Protein contents were determined by micro-Kjeldahl,Citation[17] mineral composition was determined by inductively coupled plasma atomic emission spectroscopy, and lipid and lactose contents were provided by the supplier. All chemicals were purchased from Fisher Scientific Company (Springfield, NJ).
Table 1. Composition of Commercial Whey Protein Concentrate Samples
METHODS
Preparation of Protein Dispersions and Gels
Solutions were prepared at 12% w/v protein from each WPC powder in deionized (DI) water. Each solution was gently stirred for 1 h at room temperature, and pH was adjusted to 3.35 with 6 N HCl or 6 N NaOH. WPC gels were produced by placing the solutions in aluminum freeze-drier pans (13.5 cm×13.5 cm×4.8 cm) and heating in a water bath at 80°C according to the procedure of Hudson et al.Citation[14] for 3 h.
Derivatized Whey Protein Concentrate (dWPC) Powder Production
After thermal gelation, gels were placed at −5°C for at least 24 h. Frozen gels were dried in a 10-145-MR-TR Mechanically Refrigerated Freeze-Mobile freeze dryer with tray drying chamber (Virtis Research Equipment, Gardiner, NY). The dryer operated at a condenser temperature of −55°C and a pressure of 15 microns while the shelf temperature was slowly increased from −30°C to 20°C over a drying period of about 96 h. Gels were dried until moisture content was below 5% and removed from the freeze drier pans. Dried gels were crushed by hand, then ground with a ZM-1 Brinkman Centrifugal Grinding Mill equipped with a 24-tooth stainless-steel rotor (Brinkman Instruments, Inc., Westbury, NY) at 10,000 rpm for 60 sec. Protein gel powders were stored at room temperature in airtight bags inside a desiccator until testing.
Rheological Analyses
The protein powders were reconstituted in DI water for rheological testing. Shear rate ramps, temperature ramps, and frequency sweeps were conducted on a StressTech Controlled Stress Rheometer (ReoLogica Instruments AB, Lund, Sweden) using a concentric cylinder geometry (CC25). All rheological measurements were performed in triplicate.
Shear Rate Ramps
The derivatized protein samples were reconstituted at 8% w/w protein in DI water and allowed to hydrate for 24 h. All samples were exposed to a 30 sec pre-shear at 15 s−1. Apparent viscosity and shear rates were recorded as shear stresses were ramped from 0.05 to 25 Pa. Fresh samples were sheared at temperatures of 25, 50 and 75°C.
Flow behavior was described by the cross model
To investigate the protein concentration effects, the dWPC86 sample was also prepared at concentrations of 5.5, 6.0, 6.5, 7.0 and 7.5% w/w protein. These samples were allowed to hydrate for 24 h, subjected to the same shear rate ramps at 25°C, and described by the same Cross model methodology. For the solutions with concentrations greater than 7.0%, there was no evidence of an infinite viscosity plateau, so η∞ was again assumed to be zero for the purposes of modeling data over the experimental shear rate range. The solutions with concentrations less than or equal to 6.5% exhibited infinite viscosity plateaus, therefore η∞ was incorporated into the model.
Temperature Ramps
Solutions of 8.6% w/w protein were sheared at a constant shear rate of 50 s−1 while temperatures were ramped from 10 to 90°C and back to 10°C for 2400 s while apparent viscosity was noted. A solvent trap was used, and a thin layer of mineral oil was applied to the surface of the sample to prevent moisture loss during the experiment.
Frequency Sweeps
The derivatized samples were prepared in DI water to contain 10% w/w protein and allowed to hydrate for 24 h. Storage and loss moduli (G′ and G′′) were recorded while stress amplitude was held at 0.7 Pa and frequency was increased from 0.01 to 40.0 Hz at 25°C. All frequency sweeps were performed within the identified linear viscoelastic region for each material tested. The frequency dependence of G′ and G′′ was modeled with the power law parameters, a, b, c, and d, as described by Eqs. Equation2 and Equation3.
Intrinsic Viscosity
Intrinsic viscosity was measured using Cannon-Fenske capillary viscometers (Cannon Instrument Co., State College, PA) immersed in a constant-temperature bath at 25±0.1°C. In accordance with the recommended viscosity ranges for each instrument, the derivatized protein samples were measured with a size 50 viscometer, and the commercial WPC samples were assessed with a size 25 viscometer. Stock solutions for each sample were prepared with DI water to contain 5 mg protein/mL. The stock solutions were diluted with DI water to produce a series of five concentrations between 0.5 and 5.0 mg protein/mL. The specific viscosity was calculated by
Water Holding Capacity
The water holding capacity was determined by a modification of the centrifugation procedure of Fleming et al.Citation[18] in which 7% w/w protein solutions were prepared in DI water and centrifuged at 2500 rpm for 15 min. Upon removal from the centrifuge, the supernatant was carefully decanted and the water held per gram of protein was calculated by weight difference.
Electrophoresis
The approximate contents of the individual whey proteins in each commercial WPC sample were analyzed by sodium dodecyl sulfate-polyacrylamide gel electrophoresis (SDS-PAGE) with a Novex Midget electrophoresis unit (Novex, Novel Experiment Technology, San Diego, CA). A Tris-Tricine gel with dithiothreitol (DTT) serving as the denaturant was utilized. A 0.1% protein solution was prepared for each sample by 1:1 dilution of a 0.2% protein solution with sample buffer. A 25 mL aliquot of the 0.1% protein sample was injected into each well of the 10–20% gradient polyacrylamide gel (Novex, Novel Experiment Technology, San Diego, CA). The gel was stained with a colloidal Coomassie solution (Pierce Chemical Company, Rockford, IL), and the protein bands were quantified with a Molecular Dynamics personal densitometer (Molecular Dynamics, Sunnyvale, CA).
RESULTS AND DISCUSSION
Rheological Analyses
Shear Rate Ramps
Shear rate ramps were performed at 25, 50, and 75°C to investigate the shear and temperature effects on the apparent viscosity of the three derivatized whey protein concentrate (dWPC) powders. Table summarizes the Cross model parameters as well as the apparent viscosities at shear rates of 10 and 50 s−1 (η10 and η50) for each of the shear rate ramps. The dWPC86 sample produced the greatest viscosity at each temperature. At the three temperatures evaluated, the dWPC74 and dWPC64 samples exhibited similar viscosities that were significantly lower than the dWPC86 sample. All samples showed a decrease in viscosity from 25 to 50°C due to the increased thermal energy of the system. A viscosity increase was observed in each sample from 50 to 75°C, with the dWPC64 sample exhibiting the greatest viscosity change. This increase in viscosity can be attributed to additional protein unfolding and the formation of physical crosslinks. Hudson et al.Citation[14] demonstrated that an increased concentration of salts had a stabilizing effect on the protein, resulting in a greater percentage of native protein remaining after a thermal treatment at pH values near 3.35. Harwalkar and KalabCitation[19] also reported a protective effect from denaturation when ionic strength was increased from 0 to 0.1. The protein solutions used in this research were within the ionic strength range of 0 to 0.1. Therefore, the dWPC64 sample, which was derived from the WPC sample containing the highest level of salts (Table ), likely had a greater amount of undenatured protein available to unfold and produce the greatest increase in solution viscosity during temperature studies.
Table 2. Cross Model Parameters of 8% (w/w Protein) Derivatized Whey Protein Concentrate Solutions at 25, 50, and 75°C
The dWPC86 sample was prepared at a range of concentrations and subjected to shear rate ramps to examine the concentration effects. Solutions of less than 5% protein were very thin and would offer little viscosifying power. Solutions with concentrations from 5.5 to 8.0% exhibited steadily increasing thickening ability (Table ). These solutions manifested typical rheological characteristics of a thickened fluid: a zero shear viscosity plateau at low shear rates, a shear-thinning regime, and an infinite shear plateau at high shear rates for the thinner solutions (Fig. ).
Table 3. Cross Model Parameters for dWPC86 Solutions with Concentrations Between 5.5 and 8.0% (w/w Protein) at 25°C
Temperature Ramps
Subjecting the dWPC86 sample to a temperature ramp under a shear rate of 50 s−1 produced little variation in apparent viscosity (Fig. ). As the temperature was increased from 10 to 60°C, the viscosity gradually decreased from 1.35 to 0.52 Pa s. From 60 to 90°C, there was a subsequent 0.10 Pa s increase in viscosity, possibly attributed to additional denaturation of native or renatured proteins.Citation[14] This protein unfolding and increased hydrophobic interactions were responsible for the higher viscosity during the 90 to 10°C temperature ramp. The dWPC74 and dWPC64 samples also displayed a similar temperature dependency over this range.
Frequency Sweeps
Small amplitude oscillatory rheology was used to determine the mechanical spectrum of each dWPC powder. The power law parameters relating the storage modulus (G′) and loss modulus (G′′) to frequency for each sample are presented in Table . The power law coefficients, a and c, represent the magnitude of G′ and G′′ respectively at a given frequency. The dWPC86 sample had the greatest values of a and c, while the dWPC74 and dWPC64 samples had lower, but similar magnitudes for their moduli. In all three samples, the magnitude of a was much greater than c. The existence of such a pronounced region where the storage modulus is considerably greater than the loss modulus, as represented graphically in Fig. , indicates gel behavior.Citation[20] The power law exponents b and d represent the slope of the relationships between the moduli and frequency. An exponent value of zero would indicate the modulus is not changing with frequency, a characteristic of a fully cured gel. The dWPC86 sample exhibited the lowest values of b and d, while the dWPC74 and dWPC64 samples had similar, higher slope values. The dependence of G′ and G′′ on frequency illustrates that the derivatized WPC samples form weak gels under these conditions.
Table 4. Viscoelastic Power Law Constants for 10% (w/w Protein) dWPC Solutions at 25°C
Intrinsic Viscosity
Intrinsic viscosity, [η], is a characteristic property of a polymer in a specific solvent that measures the hydrodynamic volume occupied by a polymer molecule. The largest intrinsic viscosity was produced by dWPC86 (Table ), suggesting the derivatization process produced particles capable of occupying the greatest hydrodynamic volume once hydrated in solution. The dWPC74 and dWPC64 samples displayed intrinsic viscosities of 56.4 and 49.5 mL/g. This response is indicative of smaller and/or more spherical particles than those of dWPC86. The intrinsic viscosities of the derivatized samples were much greater than those of the commercial WPC samples from which they were derived. The commercial WPC samples had very low intrinsic viscosities ranging from 6–8 mL/g. These values agree with the findings of Vardhanabhuti and FoegedingCitation[21] who reported a value of 5.0 mL/g for a native whey protein isolate sample. The plots of ηsp/c vs. c for each dWPC powder had negative slopes (Fig. ). A possible explanation is that the dWPC powders behaved as polyelectrolytes. Dilution decreases the ionic strength and leads to an increase in the repulsive forces, resulting in the expansion of the molecule and an increase in the reduced viscosity (ηsp/c).Citation[21]
Table 5. Intrinsic Viscosity and Water Holding Capacity of Commercial and Derivatized Whey Protein Concentrate Powders at 25°C
Water Holding Capacity
The derivatized protein powders possessed a much greater capacity to hold water than the commercial WPCs (Table ). With the underivatized commercial WPC samples, nearly all of the powder remained in solution after centrifugation. Because there was no pellet created by centrifugation capable of retaining water, the water holding capacity was deemed to be zero. Conversely, upon centrifugation of the derivatized protein solutions, the derivatized powders were observed to hold greater than seven times their weight in water.
Electrophoresis
In the aforementioned experiments, the dWPC86 sample consistently demonstrated superior thickening and water holding abilities, while the dWPC74 and dWPC64 samples exhibited levels of functionality that were similar to one another, but lower than that of the dWPC86 sample. To attempt to explain these differences in performance, the compositions of the commercial WPC samples were examined by electrophoresis and mineral content analysis. Because the protein component in each sample is ultimately responsible for the thickening functionality, SDS-PAGE was employed to determine if differences existed in the amounts of the specific whey proteins in each commercial WPC. Variations in the original whey composition and the whey protein concentrate production conditions could result in WPCs with different protein constituents and functionalities. However, Fig. clearly shows that the three commercial WPCs had very similar protein profiles. Quantification of the protein bands by densitometry revealed no significant differences in the quantities of individual proteins.
Figure 5. SDS-PAGE gel of commercial whey concentrate powders. Lane 1 is a molecular weight marker, lanes 2–4 are WPC86, lanes 5–7 are WPC74, and lanes 8–10 are WPC64.
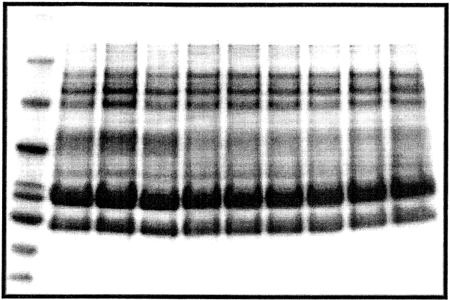
The WPC compositions do offer possible explanations for the results of the dWPC functionality tests. Table shows that the WPC86 sample contained the lowest quantities of the major minerals, especially calcium, while the WPC74 and WPC64 samples had similar mineral contents. The level of salts influences the type of gel structure formed during the 3 h gelation period at 80°C. At low salt concentrations the electrostatic repulsions between proteins are only partially shielded and a translucent fine-stranded filament gel is formed. As the salt concentration is increased, the electrostatic repulsions are completely shielded and attractive forces dominate, resulting in protein aggregation and the formation of a particulate gel.Citation[22] Hudson et al.Citation[14] reported that in the production of derivatized WPI powders, solutions with higher salt concentrations permitted aggregation and formation of more “particulate-type” fine-stranded gel structures. Upon freeze-drying and milling, the particulate gel structures were less effective in producing cold-set thickening functionality than the fine stranded gel structures. Based on these previous findings, the lower level of salts in the WPC86 sample may have limited protein aggregation more than in the WPC74 and WPC64 samples, creating a more fine-stranded gel which upon further processing rendered a more functional thickener. Furthermore, the similarity in the mineral contents of the WPC74 and WPC64 samples may have been responsible for the similarities in the rheological and physical properties displayed by the dWPC74 and dWPC64 samples.
The lactose and lipid contents of each WPC sample listed in Table may also provide rationale for the results of the dWPC characterization. The WPC86 sample had significantly lower contents of both lactose and lipid. The increased sugar content in the WPC74 and WPC64 protected the proteins from denaturation during the heating and tended to produce a more particulate-type gel structure. The higher lipid content may have also influenced the gel structure, as interactions between lipids and whey proteins, particularly β-lactoglobulin, are well established.Citation[23] However, additional research is needed to further delineate the precise role played by minerals, lipids, and lactose in the performance of derivatized whey protein concentrate powders.
CONCLUSIONS
A procedure for production of cold-set gelling derivatized whey protein isolate was successfully applied to three commercial whey protein concentrates. The derivatization procedure greatly increased the ability of the powders to impart viscosity and hold water. Weak gel structures were formed by all three derivatized powders at ambient temperatures without addition of salts. The performance differences among the derivatized powders were explained by the influence of the mineral content on the thermal gelation whey proteins during the production of the derivatized powders. The cold-set gelation capability gives rise to a wide range of applications such as sports drinks, dairy beverages, sauces, fillings, and infant and enteral formulations.
ABBREVIATIONS
WPI, whey protein isolate; WPC, whey protein concentrate; WPC86, whey protein concentrate −86% protein; dWPC, derivatized whey protein concentrate; DI, deionized; SDS-PAGE, sodium dodecyl sulfate-polyacrylamide gel electrophoresis
AKNOWLEDGMENTS
The authors would like to thank Tim Seaboch for his freeze drying assistance, Davisco Foods International, Inc. for providing the whey protein concentrate samples, and the Southeast Dairy Foods Research Center for supporting this study.
Paper No. FSR01-12 of the Journal Series of the North Carolina Agricultural Research Service, Raleigh, NC 27695-7643. The use of trade names does not imply endorsement by the North Carolina Agricultural Research Service of products named, nor criticisms of similar ones not mentioned.
REFERENCES
- Harper , W.J. 2000 . Biological Properties of Whey Components: A Review Chicago, IL : American Dairy Products Institute .
- Kinsella , J.E. and Whitehead , D.M. 1989 . Proteins in Whey: Chemical, Physical, and Functional Properties . Advances in Food and Nutrition Research , 33 : 343 – 438 .
- Ju , Z.Y. and Kilara , A. 1998 . Textural Properties of Cold-set Gels Induced from Heat-denatured Whey Protein Isolates . Journal of Food Science , 63 : 288 – 292 .
- Mulvihill , D.M. and Kinsella , J.E. 1987 . Gelation Characteristics of Whey Proteins and β-Lactoglobulin . Food Technology , 41 : 102 – 111 .
- Ziegler , G.R. and Foegeding , E.A. 1990 . The Gelation of Proteins . Advances in Food and Nutrition Research , 34 : 203 – 298 .
- Mangino , M.E. 1992 . Gelation of Whey Protein Concentrates . Food Technology , 46 : 114 – 117 .
- Barbut , S. and Foegeding , E.A. 1993 . Ca 2+-induced Gelation of Pre-heated Whey Protein Isolate . Journal of Food Science , 58 : 867 – 871 .
- McClements , D.J. and Keoh , M.K. 1995 . Physical Properties of Cold-setting Gels Formed from Heat-denatured Whey Protein Isolate . Journal of the Science of Food and Agriculture , 69 : 7 – 14 .
- Nakamura , M. , Sato , K. , Koizumi , S. , Kawachi , K. , Hishiya , T. and Nakajima , I. 1995 . Preparation and Properties of Salt-induced Gel of Whey Protein . Nippon Shokuhin Kagaku Kogaku Kaishi , 42 : 1 – 6 .
- Sato , K. , Nakamura , M. , Koisumi , S. , Kawachi , K. , Nishiya , T. and Nakajima , I. 1995 . Changes in Hydrophobicity and SH Content on Salt-induced Gelation of Whey Protein . Nippon Shokuhin Kagaku Kogaku Kaishi , 42 : 1 – 6 .
- Thomsen , B. 1994 . Whey Protein Texturizer . European Food and Drink Review , : 46 – 47 .
- Elofsson , C. , Dejmek , P. , Paulsson , M. and Burling , H. 1998 . Characterization of a Cold-gelling Whey Protein Concentrate . International Journal of Dairy Science , 7 : 601 – 608 .
- Huang , X.L. , Catignani , G.L. and Swaisgood , H.E. 1999 . Modification of Rheological Properties of Whey Protein Isolates by Limited Proteolysis . Nahrung , 43 : 79 – 85 .
- Hudson , H.M. , Daubert , C.R. and Foegeding , E.A. 2000 . Rheological and Physical Properties of Derivatized Whey Protein Isolate Powders . Journal of Agricultural and Food Chemistry , 48 ( 8 ) : 3112 – 3119 .
- Rao , M.A. 1999 . Rheology of Fluid and Semisolid Foods Gaithersburg, Maryland : Aspen .
- Steffe , J.F. 1996 . Rheological Methods in Food Process Engineering East Lansing, Michigan : Freeman Press .
- 1984 . “ AOAC ” . In Official Methods of Analysis , 14 Arlington, VA : Association of Official Analytical Chemists .
- Fleming , S.E. , Sosulski , F.W. , Kilara , A. and Humbert , E.S. 1974 . Viscosity and Water Absorption Characteristics of Slurries of Sunflower and Soybean Flours, Concentrates and Isolates . Journal of Food Science , 39 : 188 – 191 .
- Harwalkar , V.R. and Kaleb , M. 1985 . Thermal Denaturation and Aggregation of β-lactoglobulin at pH 2.5. Effect of Ionic Strength and Protein Concentration . Milchwissenschaft , 40 ( 1 ) : 31 – 34 .
- Almdal , K. , Dyre , J. , Hvidt , S. and Kramer , O. 1993 . Towards a Phenomenological Definition of the Term ‘gel.’ . Polymer Gels and Networks , 1 : 5 – 17.21 .
- Vardhanabhuti , B. and Foegeding , E.A. 1999 . Rheological Properties and Characterization of Polymerized Whey Protein Isolates . Journal of Agricultural and Food Chemistry , 37 ( 9 ) : 3649 – 3655 .
- Bryant , C.M. and McClements , D.J. 1998 . Molecular Basis of Protein Functionality With Special Consideration of Cold-set Gels Derived From Heat-denatured Whey . Trends in Food Science and Technology , 9 : 143 – 151 .
- Brown , E.M. 1984 . Interactions of β-lactoglobulin and α-lactalbumin With Lipid: A Review . Journal of Dairy Science , 67 : 713 – 727 .