Abstract
Depression increasingly affects a wide range and a large number of people worldwide, both physically and psychologically, which makes it a social problem requiring prompt attention and management. Accumulating clinical and animal studies have provided us with substantial insights of disease pathogenesis, especially central monoamine deficiency, which considerably promotes antidepressant research and clinical treatment. The first-line antidepressants mainly target the monoamine system, whose drawbacks mainly include slow action and treatment resistant. The novel antidepressant esketamine, targeting on central glutamatergic system, rapidly and robustly alleviates depression (including treatment-resistant depression), whose efficiency is shadowed by potential addictive and psychotomimetic side effects. Thus, exploring novel depression pathogenesis is necessary, for seeking more safe and effective therapeutic methods. Emerging evidence has revealed vital involvement of oxidative stress (OS) in depression, which inspires us to pursue antioxidant pathway for depression prevention and treatment. Fully uncovering the underlying mechanisms of OS-induced depression is the first step towards the avenue, thus we summarize and expound possible downstream pathways of OS, including mitochondrial impairment and related ATP deficiency, neuroinflammation, central glutamate excitotoxicity, brain-derived neurotrophic factor/tyrosine receptor kinase B dysfunction and serotonin deficiency, the microbiota-gut-brain axis disturbance and hypothalamic-pituitary-adrenocortical axis dysregulation. We also elaborate on the intricate interactions between the multiple aspects, and molecular mechanisms mediating the interplay. Through reviewing the related research progress in the field, we hope to depict an integral overview of how OS induces depression, in order to provide fresh ideas and novel targets for the final goal of efficient treatment of the disease.
Depression
Depression is a category of mental disorder, characterized by core symptoms of depressed mood (for at least two weeks) and anhedonia, which influences a wide range and a large number of people worldwide. The global prevalence of the disease is approximately 6% and the lifetime risk is about 18%, which means about one out of six people would experience at least one episode in the lifetime (CitationMalhi & Mann, 2018; CitationOtte et al., 2016). According to the statistics from the World Health Organization and related report, over 280 million people have been suffering from the disease globally (CitationDamian et al., 2021), among which is over 95 million Chinese people, including PhD students (CitationWoolston & O’Meara, 2019; CitationY. Huang et al., 2019). Furthermore, the influence of global COVID-19 epidemic in recent years on the disease has started to emerge (CitationDamian et al., 2021; CitationRacine et al., 2021). Additionally, a considerable part of patients has not been properly diagnosed and attended, due to lack of awareness, limited medical resources, etc. For instance, the disease situation in children and adolescents remains unclarified, which receives increasing attention both locally and globally (CitationKong et al., 2022; Theo et al., 2020). The data indicate the disorder as an urgent social problem requiring prompt attention and management, and highlight the necessity to elucidate depression pathogenesis. The accumulating clinical and animal studies over years have provided us with in-depth understanding of the disease, and considerably promoted antidepressant research. Diversified disease hypotheses have been proposed, such as the classical hypothalamic-pituitary-adrenocortical (HPA) axis dysregulation and central serotonin (5-HT) deficiency, and the novel glutamate excitotoxicity, brain-derived neurotrophic factor/tyrosine receptor kinase B (BDNF/TrkB) dysfunction and neuroinflammation in the brain, and microbiota-gut-brain (MGB) axis disturbance (CitationKrishnan & Nestler, 2008; CitationMalhi & Mann, 2018).
The Vital Role of Oxidative Stress in Depression
Oxidation and reduction are the basic reactions of cell metabolism. Oxidation produces a variety of free radicals, mainly divided into reactive oxygen species (ROS) and reactive nitrogen species. The antioxidant system in the body is responsible for removing free radicals, including antioxidant enzymes and small molecules, such as superoxide dismutase (SOD), catalase (CAT), glutathione peroxidase (GPx), glutathione (GSH), etc. For example, SOD can catalyze superoxide (O2−) to hydrogen peroxide, which could then be catalyzed by CAT and GPx into oxygen and water (CitationAli et al., 2020). However, excessive oxidation or insufficient antioxidation would cause substantial free radical accumulation, which would result in disrupted homeostasis referred as oxidative stress (OS). Take ROS, for example, which are chemical molecules that contain one oxygen atom thus are more reactive than oxygen, such as superoxide anion (O2•−), and primarily generated by mitochondria’s electron transport chain and NADPH oxidase (NOX) activity (CitationTauffenberger & Magistretti, 2021). Physiological levels of ROS have prominent roles in the modulation of a variety of cell signals, among which extracellular signal-related kinases 1/2 (ERK1/2), mammalian target of rapamycin, phosphatidylinositide 3-kinase (PI3K) and protein kinase B are critically involved in cell survival and growth, while nuclear factor kappa-B (NF-κB) and nuclear factor erythroid 2-related factor 2 are closely related to expressions of antioxidant enzymes and molecules, and metabolism-related transcription factors (CitationBallard & Towarnicki, 2020; CitationTauffenberger & Magistretti, 2021). However, excess ROS under OS condition would disturb normal physiological signals, induce peroxidation of nucleic acids, lipids and proteins, eventually causing cell damage and pathological reactions (CitationBallard & Towarnicki, 2020; CitationTauffenberger & Magistretti, 2021). In particular, the damage of cell membrane lipid bilayers will lead to abnormal transmembrane exchanges of a variety of molecules, ions, etc., which would disrupt physiological and biochemical homeostasis within the cell, and consequentially cause cell damage. The brain neurons, astrocytes and microglia are rich in mitochondria and NOX, thus capable of generating abundant ROS (CitationCulmsee et al., 2018; CitationNayernia et al., 2014). Meanwhile, brain is a high oxygen consuming organ, with high cortical surface area, enriched with unsaturated fatty acids but lack of antioxidant enzymes, which result in low antioxidant capacity and high vulnerability to OS (CitationCobley et al., 2018; CitationPatel, 2016).
Studies have revealed the pivotal role of OS in neurodegeneration diseases, such as the Alzheimer’s disease and Parkinson’s disease (CitationChen & Zhong, 2014; CitationSubramaniam & Chesselet, 2013). Its emerging role in depression has been identified in recent years, which receives increasing attention (CitationBhatt et al., 2020; CitationWang et al., 2023). As supporting evidence, studies found significantly reduced antioxidants (e.g., SOD, GSH) and increased peroxidation biomarkers, such as carbonyl and malondialdehyde (MDA), in depressed patients and animals (CitationJuszczyk et al., 2021; CitationLiu et al., 2015; CitationVisentin et al., 2020). Clinical studies found increased peroxidation biomarkers (MDA, 8-OHdG and F2-isoprostanes) and reduced antioxidants (e.g., SOD), in the plasma and serum of major depressive disorder (MDD) patients (CitationCamkurt et al., 2016; CitationHerken et al., 2007; CitationLindqvist et al., 2017; CitationYager et al., 2010; , oxidative stress section). Animal studies further specified stress-induced abnormalities across brain subregions, such as the reduced GSH and SOD activity, and the increased levels of ROS, MDA and carbonyl, in the prefrontal cortex (PFC) and hippocampus of depressed rats, caused by chronic unpredictable mild stress (CUMS), chronic restraint stress (CRS) and chronic social isolation, respectively (CitationParul et al., 2021; CitationZafir & Banu, 2007; CitationZlatković et al., 2014; , oxidative stress section). Moreover, the antioxidant effect of antidepressants, and antidepressant effect of antioxidants have been revealed in depressed patients and rodents, respectively. For example, antidepressants fluoxetine and citalopram increased the SOD activity, and reduced MDA and carbonyl levels in MDD patients (CitationHerken et al., 2007; CitationKhanzode et al., 2003) and CRS treated rats (CitationZafir & Banu, 2007), while antioxidant diallyl disulfide reversed lipopolysaccharide (LPS)-induced depressive-like behaviors in mice (CitationWei et al., 2021). The results indicate OS as an important depression pathogenesis, and further suggest enhancing antioxidant activity as a promising therapeutic avenue. To expound possible underlying mechanisms mediating the OS influence, the following sections describe mitochondrial impairment and related adenosine triphosphate (ATP) deficiency, neuroinflammation, glutamate excitotoxicity, BDNF/TrkB dysfunction and 5-HT deficiency in the brain, and disturbed MGB and HPA axes. We also elaborate the intricate interactions between the above aspects, and the molecular mechanisms mediating the interplay.
Table 1 The Underlying Mechanisms of OS Induced Depression, as Revealed by Clinical Studies.
Table 2 The Underlying Mechanisms of OS Induced Depression, as Revealed by Rodent Studies.
The Underlying Mechanisms of OS-Induced Depression
Mitochondrial Impairment and ATP Deficiency in the Brain
Mitochondria are organelles that are mainly responsible for oxidative metabolism of energy substances (e.g., carbohydrates, proteins, lipids), to provide ATPs for organisms. They are one primary source of ROS generation, and in turn are the most vulnerable organelles of excess ROS. Brain neurons, astrocytes and microglia are enriched with mitochondria thus could generate abundant ATPs, which meanwhile consume a large quantity of ATPs for executing complicated brain functions, rationalizing the extensively affected brain functions caused by mitochondrial impairment and ATP deficiency. Clinical studies have found morphological and functional abnormalities of brain mitochondria in MDD patients, such as reduced ATP generation, electron transport chain and enzyme activity (CitationAllen et al., 2018; CitationBansal & Kuhad, 2016; , mitochondrial impairment section). Animal studies found maternal separation (MS) and corticosterone impaired central mitochondrial metabolism, and induced depressive-like behaviors in rats and mice, which were reversed by physical exercise and nicotinamide mononucleotide administration (CitationPark et al., 2019; CitationXie, Shen et al., 2020; CitationXie, Yu et al., 2020; , mitochondrial impairment section). Impaired mitochondrial metabolism would lead to reduced ATP generation, in a way that mitochondrial ROS (mtROS) induces cardiolipin peroxidation, which reduces the activity of complex V (ATP synthase) of mitochondrial electron transport chain (Citationde Oliveira et al., 2021; CitationPointer & Klegeris, 2017; ). Rodent studies found central ATP deficiency, astrocytes derived and medial prefrontal cortex (mPFC) localized, resulted in depressive-like behaviors in mice, which were ameliorated by both exogenous ATP administration and stimulated endogenous ATP release, indicating the pivotal role of central ATPs in depression onset and remedy, respectively (CitationCao et al., 2013; CitationLin et al., 2022). Furthermore, astrocytic epoxyeicosatrienoic acid signaling in the mPFC modulated depressive-like behaviors in mice, through regulating the astrocytic ATP release that was required for antidepressant effect (CitationXiong et al., 2019). The above evidence suggests critical involvement of central mitochondrial impairment and related ATP deficiency in depression.
Figure 1 Os causes depression through inducing mitochondrial impairment (A), neuroinflammation (B), glutamate excitotoxicity (C), BDNF/TrkB dysfunction (D) and 5-HT deficiency (E) in the brain, and the MGB disturbance (F) and the HPA axis dysregulation (G). A. Mitochondrial impairment induces central ATP deficiency, through elevating mtROS and reducing cardiolipin and ATP synthase, with astrocytes derived and mPFC localized ATP deficiency closely associated with depression. OS and mitochondria have mutual influence through mtROS, neuroinflammation and excess glutamate induced Ca2+ influx (A-OS). B. Neuroinflammation is characterized by microglia activation, pro-inflammatory cytokine expressions, etc. OS/Mitochondria-neuroinflammation interaction (AB, B-OS): Excess ROS increases pro-inflammatory cytokines, through elevating histone acetylation and activating NLRP3/caspase-1, AP-1 and NF-κB, and aberrant astrocytic OXPHOS activity; neuroinflammation promotes IDO/TDO/KMO to generate QUIN that activates NMDA, increases glutamate, triggers Ca2+ influx, which causes cytochrome C release and reduces SIRT3 activity and ATP generation, or depolarizes mitochondria membrane and generates excess ROS. C. Glutamate excitotoxicity is characterized by central synaptic accumulation. Glutamate-OS interaction (C-OS): Excess ROS results in excitotoxicity through affecting Ca2+, GS and GLT-1 expression; excess glutamate impairs mitochondria through NMDA activation and Ca2+ influx. Neuroinflammation-glutamate interaction (BC): Neuroinflammation promotes excitotoxicity through increasing microglial release or blocking astrocytic reuptake, by downregulating GLT-1 or elevating GLS and QUIN; excess glutamate might increase pro-inflammatory cytokines through NMDA/AMPA receptors, but reduce the cytokines through mGluRs. D. BDNF/TrkB dysfunction is characterized by inhibited BDNF expression and TrkB activation (PFC/hippocampus). BDNF-OS interaction (D-OS): Excess ROS inhibits BDNF expression, through reducing CREB and elevating NF-κB activity; BDNF owns antioxidant property through TrkB, ERK1/2 and NF-κB/sestrin2, thus, the dysfunction promotes OS. Neuroinflammation-BDNF/TrkB interaction (BD): NF-κB mediates LPS-reduced BDNF expression through interacting with BDNF/TrkB; BDNF promotes TNF-α and IL-1β release by activating astrocytes and microglia. Glutamate-BDNF/TrkB interaction (CD): Excess glutamate might inhibit BDNF expression by activating extra-synaptic NMDA and eEF2; BDNF promotes the release or protects neurons against the excitotoxicity of glutamate, through PI3K, PLC-γ, ERK, etc., whose dysfunction weakens the influence correspondingly. E. Central 5-HT interacts with multiple aspects, whose deficiency attenuates the influence correspondingly: 5-HT presents antioxidant properties, while OS inhibits 5-HT through neuroinflammation and MGB disturbance (E-OS); Neuroinflammation inhibits 5-HT by promoting KP and modulating 5-HTT (BE); 5-HT inhibits glutamate transmission through 5-HT 1A/B receptor, which in turn regulates 5-HT function through VGLUT1 (CE); 5-HT promotes BDNF expression and TrkB activation, while BDNF affects 5-HTT function (DE). F. MGB disturbance is characterized by disturbed microbiota composition/metabolism. MGB-OS/mitochondria interaction (AF, F-OS): Microbiota affects ROS generation by modulating activities of NOX, SCFAs and antioxidants, and mitochondria metabolism; Mitochondrial genotype/mutation affects microbiota through mtROS. Neuroinflammation-MGB interaction (BF): MGB disturbance leads to gut inflammation, increases QUIN that damages BBB and results in neuroinflammation; Neuroinflammation disturbs microbiota homeostasis, through pro-inflammatory cytokines and NLRP3. BDNF-MGB interaction (DF): BDNF modulates intestinal barrier integrity; MGB disturbance reduces hippocampal BDNF expression. 5-HT-MGB interaction (EF): Microbiota disturbance inhibits 5-HT generation, by promoting intestinal/central KP, affecting 5-HT and TPH 1 gene expressions; 5-HTT genotype modulates microbiota composition. G. HPA axis interacts with multiple aspects, whose dysregulation attenuates the influence correspondingly: HPA affects ROS generation, mitochondria gene expression and metabolism through GRs, while NO mediates IL-1β and cholinergic stimulation of HPA (G-OS); HPA interacts with neuroinflammation through cytokines and GR/NF-κB signaling (BG); HPA affects glutamate release (through GR-TrkB interaction) and related gene expression (CG); GR activation reduces BDNF expression, while BDNF affects GR phosphorylation and rewrote glucocorticoid transcriptome (DG); HPA hormones increase intestinal barrier permeability and disturb microbiota composition, while MGB affects HPA through GABAergic activity (FG). AB, BC, CD, DE, EF and AF have indirect interaction through OS. AP-1 = activating protein-1; AMPA = α-amino-3-hydroxy-5-methyl-4-isoxazolepropionic; ATP = adenosine triphosphate; BDNF = brain-derived neurotrophic factor; CREB = cAMP responsive element binding protein; EAATs = excitatory amino acid transporters; eEF2 = eukaryotic elongation factor 2; ERK = extracellular signal-related kinases; GS = glutamine synthetase; GLS = glutaminase; Glu = glutamate; GLT-1 = glutamate transporter 1; IDO = indoleamine 2,3-dioxygenase; IL-1β = interleukin-1β; IL-6 = interleukin-6; KMO = kynurenine 3-monooxygenase; KP = kynurenine pathway; MGB = microbial-gut-brain; mPFC = medial prefrontal cortex; mtROS = mitochondrial ROS; NF-κB = nuclear factor kappa-B; NLRP3 = NOD-like receptor thermal protein domain associated protein 3; NMDA = N-methyl-D-aspartic acid; NOX = NADPH oxidase; OS = oxidative stress; OXPHOS = oxidative phosphorylation; PLC-γ = phospholipase C-γ; PKC = protein kinase C; PI3K = phosphatidylinositide 3-kinase; PVN = paraventricular nucleus; QUIN = quinolinic acid; RNS = reactive nitrogen species; ROS = reactive oxygen species; SCFAs = short-chain fatty acids; SIRT3 = sirtuin 3; SRC =sparse representation-based classification; TDO =tryptophan-2,3-dioxygenase; TPH = tryptophan hydroxylase; TNF-α = tumor necrosis factor-α; TrkB = tyrosine kinase B; TRYCATs = tryptophan catabolites; VGLUT1 = vesicular glutamate transporter 1; 5-HTT = serotonin transporter.
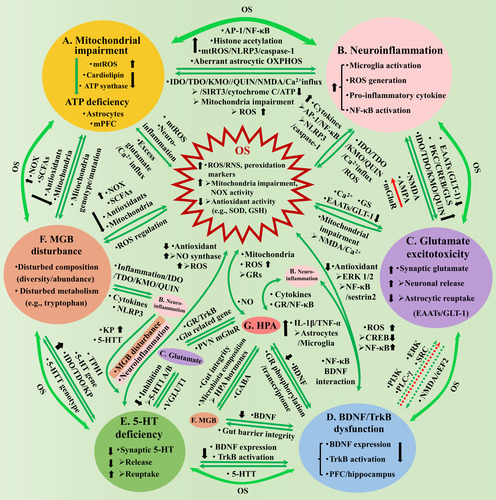
Studies have revealed mutual interaction between OS and mitochondrial impairment, through mtROS, neuroinflammation, excess glutamate-induced Ca2+ influx, etc. (Citationde Oliveira et al., 2021; CitationMoncrieff et al., 2022; CitationVisentin et al., 2020; -OS). The mutual influence between mitochondrial impairment and neuroinflammation is critically involved in depression (CitationHollis et al., 2022; CitationVisentin et al., 2020; ). Impaired mitochondria leads to increased mtROS generation, which promotes pro-inflammatory cytokine gene expression through promoting protein-1 (AP-1) and NF-κB, and increasing histone acetylation (CitationAdcock et al., 2005; CitationRahman et al., 2004), or activating caspase-1 and inflammasome NOD-like receptor thermal protein domain associated protein 3 (NLRP3) (CitationVisentin et al., 2020; CitationZhou et al., 2011), or affecting astrocytic mitochondrial oxidative phosphorylation activity (CitationMi et al., 2023). In turn, neuroinflammation promotes mtROS generation, for instance, interleukin-1β (IL-1β) and IL-18 promote kynurenine pathway (KP) of tryptophan metabolism, through activating the enzymes of indoleamine 2,3-dioxygenase (IDO), tryptophan-2,3-dioxygenase (TDO) and kynurenine 3-monooxygenase (KMO), and generating tryptophan catabolites (TRYCATs) among which quinolinic acid (QUIN) directly activates N-methyl-D-aspartic acid (NMDA) receptors, increases synaptic glutamate, triggers Ca2+ influx that depolarizes mitochondrial membrane, which finally increases mtROS generation and reduces antioxidant activity (Citationde Oliveira et al., 2021; CitationSchwarcz et al., 2012; CitationVisentin et al., 2020). Furthermore, increased intracellular Ca2+ activates neural nitric oxide synthase (NOS) and elevates nitric oxide level, decreases the activity of sirtuin 3 (SIRT3, an important deacetylase), causes mitochondrial permeability transition pores open and cytochrome C release, and finally reduces ATP generation (CitationVisentin et al., 2020). In addition, tumor necrosis factor-α (TNF-α) phosphorylates tyrosine 304 in subunit I of cytochrome C oxidase in complex IV, to impair mitochondria and reduce ATP generation (CitationVisentin et al., 2020).
Neuroinflammation
Neuroinflammation is the immune response of the brain to disturbed internal homeostasis, which involves microglia activation, pro-inflammatory cytokine release, ROS generation, NF-κB and NLRP3 expression and activation, etc. (CitationRansohoff et al., 2015). Since the early clinical findings of depressive symptoms in hepatitis C patients treated with interferon-α (CitationBonaccorso et al., 2002; CitationKeefe, 2007), accumulating studies have revealed the prominent role of neuroinflammation in depression (CitationBeurel et al., 2020; CitationTroubat et al., 2021; , –, neuroinflammation section). Several pro-inflammatory pathways in the pathogenesis, treatment and remission of MDD have been addressed, including the caspase 1, inducible NOS (iNOS) and interferon-gamma (IFN-γ) (CitationInserra et al., 2019). Clinical studies found significantly elevated levels of IL-1β,IL-6 and TNF-α in the serum (CitationLindqvist et al., 2017; CitationZou et al., 2018), and increased expressions of IL-6, TNF-α and neuroinflammation biomarker 18-kda translocator protein, in the cerebrospinal fluid (CSF) and brain subregions, including the anterior cingulate cortex (ACC), PFC and hippocampus (CitationEnache et al., 2019; CitationGritti et al., 2021), in MDD patients (, neuroinflammation section). Rodent studies demonstrated early life stress perturbed microglial gene expression, morphology and function in the developing rodent hippocampus (CitationJohnson & Kaffman, 2018). MS increased microglial activation and expressions of pro-inflammatory cytokines IL-6, INF-γ and TNF-α in rat PFC (CitationGiridharan et al., 2019). Similarly, later life social isolation stress upregulated the NF-κB activation and iNOS expressions in rat PFC (CitationZlatković et al., 2014; CitationZlatković & Filipović, 2013), while CUMS increased the expressions of TNF-α and IL-1β, NLRP3 and transcription regulator NF-κB, in the PFC and hippocampus of rats (CitationPan et al., 2014; CitationParul et al., 2021; , neuroinflammation section).
Furthermore, clinical and animal studies found anti-inflammatory effect of antidepressants (CitationDionisie et al., 2021; CitationWiędłocha et al., 2018), and antidepressant effect of anti-inflammatory drugs (CitationSimon et al., 2023). For instance, clinical studies found behavioral therapy reduced peripheral IL-6 level (CitationMa et al., 2022), while antidepressant fluoxetine reduced the levels of IL-1β, IL-6 and TNF-α (CitationGarcia-Garcia et al., 2022), in MDD patients. Similarly, rodent studies found fluoxetine reduced the CUMS elevated IL-1β in rat PFC (CitationPan et al., 2014), and the LPS elevated IL-1β, IL-6 and TNF-α in mouse hippocampus (CitationDuda et al., 2017). On the other hand, drugs with anti-inflammatory properties, such as the Geraniol, Sinomenine, Patchouli alcohol, Baicalin and Berberine, alleviated the the CUMS and corticosterone-induced depressive-like behaviors in mice, respectively (CitationBai et al., 2023; CitationDeng et al., 2015; CitationHe et al., 2023; CitationL. T. Guo et al., 2019; CitationLiu et al., 2018; CitationQin et al., 2023). Patchouli alcohol and Berberine take effect through inhibiting the NLRP3-mediated neuroinflammation (CitationHe et al., 2023; CitationQin et al., 2023), while Baicalin takes effect through inhibiting the toll-like receptor 4 expression via the PI3K/AKT/FoxO1 pathway (CitationL. T. Guo et al., 2019). A recent study further showed Saikosaponin C alleviated the chronic social defeated stress (CSDS)-induced depressive-like behaviors in mice, through inhibiting the IL-6 methylation, which indicates the prominent role of epigenetic modulation of pro-inflammatory cytokines in depression (CitationBai et al., 2023). The above evidence indicates neuroinflammation as an important pathogenesis of depression, and suggests anti-inflammation as a potentially effective remedy avenue.
Studies have revealed the important role of OS-neuroinflammation interaction in depression (CitationLindqvist et al., 2017; CitationRawdin et al., 2013; -OS). Excess ROS promotes pro-inflammatory cytokine expressions, through activating AP-1 and NF-κB, and increasing histone acetylation (CitationAdcock et al., 2005; CitationRahman et al., 2004), or activating caspase-1 and NLRP3 (Citationde Oliveira et al., 2021; CitationVisentin et al., 2020). In turn, neuroinflammation induces mitochondrial impairment and excess ROS generation, through activating IDO/TDO/KMO to promote KP and generate QUIN, which activates NMDA, increases synaptic glutamate, triggers Ca2+ influx that depolarizes the mitochondrial membrane (CitationCatena-Dell’Osso et al., 2013; Citationde Oliveira et al., 2021; CitationMoncrieff et al., 2022; CitationSchwarcz et al., 2012; CitationVisentin et al., 2020). As supporting evidence, studies showed both peripheral and central inflammation-promoted KP and elevated QUIN in the brain, through increasing the IDO/TDO/KMO activities (CitationConnor et al., 2008; CitationFu et al., 2010; CitationLawson et al., 2013; CitationRaison et al., 2010), which was inhibited by antidepressants (CitationEskelund et al., 2017). QUIN could act as NMDA receptor agonist and elevate synaptic glutamate (CitationTavares et al., 2002; CitationVisentin et al., 2020), and promote OS through increasing the ROS generation and lipid peroxidation (CitationRios & Santamaria, 1991; CitationStone et al., 2000).
Neuroinflammation could induce 5-HT deficiency in the brain, through promoting KP by activating IDO/TDO, which has been recognized as a classic depression pathogenesis (CitationCatena-Dell’Osso et al., 2013; CitationMoncrieff et al., 2022; ). Tryptophan, the precursor of 5-HT, is an essential amino acid obtained from the diet, which could pass blood-brain barrier (BBB) into the brain and be metabolized to 5-HT, catalyzed by tryptophan hydroxylase (TPH) 2 and aromatic amino acid decarboxylase (AAAD), or converted into kynurenine, catalyzed by IDO (CitationGheorghe et al., 2019; CitationKrautkramer et al., 2021; CitationSchwarcz et al., 2012). Neuroinflammation-promoted KP would reduce tryptophan available for the 5-HT pathway, resulting in reduced 5-HT generation in the brain. A study indeed found inflammation and CRS enhanced IDO activity, promoted KP, and reduced 5-HT concentration in mouse hippocampus, which were reversed by the IDO inhibitor 1-methyl-tryptophan (CitationDeng et al., 2021). Kynurenine could either be catalyzed by kynurenine aminotransferases to kynurenic acid in astrocytes, which acts as NMDA antagonist and exerts neuroprotection effect, or catalyzed by KMO to QUIN in microglia (CitationGheorghe et al., 2019; CitationKrautkramer et al., 2021; CitationSchwarcz et al., 2012). QUIN could act as NMDA receptor agonist and cause synaptic glutamate accumulation, through both stimulating the release and inhibiting the uptake, thus is neurotoxic (CitationTavares et al., 2002; CitationVisentin et al., 2020). Neuroinflammation could also directly increase microglial glutamate release through increasing glutaminase (GLS), via protein kinase C and cAMP responsive element binding protein (CREB) (CitationChen et al., 2012; CitationTakeuchi et al., 2006), or inhibiting astrocytic reuptake through downregulating excitatory amino acid transporters (EAATs), especially glutamate transporter 1 (GLT-1) (CitationHaroon & Miller, 2017; CitationTilleux & Hermans, 2007; ). The above mechanisms might contribute to neuroinflammation elevated glutamate in the brain (e.g., ACC and basal ganglia) of MDD patients, assessed by magnetic resonance spectroscopy, which was closely correlation with anhedonia phenotype and increased inflammation markers (e.g., C-reactive protein) (CitationHaroon & Miller, 2017). Alternatively, neuroinflammation might regulate glutamate signaling indirectly through OS (CitationMiller & Raison, 2016), as studies found IL-1β inhibited glutamate reuptake and LPS stimulated glutamate release were blocked by oxide synthase and NOX inhibitors, respectively (CitationBarger et al., 2007; CitationHu et al., 2000). Glutamate excitotoxicity would be achieved once excess glutamate accumulated in the synaptic space of the brain, which also serves as an important downstream pathway of OS.
Central Glutamate Excitotoxicity
Glutamate is the major excitatory neurotransmitter in the brain, produced de novo from the mitochondrial tricarboxylic acid cycle intermediate metabolite α-Ketoglutarate. The intracellular glutamate concentration is approximate 10 mmol/L, much higher than the levels in synaptic cleft (1 mmol/L), extracellular fluid, CSF and plasma (0.5–4, 10, 150 μmol/L, respectively) (CitationMontanari et al., 2022; CitationPittenger et al., 2008). Glutamatergic neurons and astrocytes form glutamate-glutamine cycle to maintain synaptic homeostasis, mainly through reuptaking extracellular glutamate by astrocytic EAATs, which are the primary terminating mechanism for the actions of synaptic glutamate, as the synaptic space is short of glutamate degrading enzymes (CitationHertz & Zielke, 2004). Five subtypes of EAATs (referred as EAAT1-5) have been found so far, among which EAAT 1 and 2 (referred as GLAST and GLT-1 in rodents, respectively) are mainly expressed in astrocytes and primarily responsible for astrocytic reuptake of synaptic glutamate (CitationHertz & Zielke, 2004; CitationMurrough et al., 2017). Studies have found GLT-1 is mainly distributed in hippocampus and cerebral cortex, while GLAST is mainly distributed in cerebellum and retina (CitationTodd & Hardingham, 2020; CitationZhou et al., 2019). Glutamate cannot cross the BBB, thus excessive accumulation in the synaptic space of the brain, caused by promoted neuronal release or EAAT/GLT-1 inhibition reduced astrocytic reuptake, leads to glutamate excitotoxicity, which would damage central neurons and glial cells, and cause neurological and psychiatric disorders (CitationLau & Tymianski, 2010; CitationPajarillo et al., 2019).
Considerable studies have revealed the prominent role of glutamate excitotoxicity in depression (CitationMurrough et al., 2017; CitationSanacora et al., 2008; , –, glutamate excitotoxicity section). Clinical studies found significantly increased glutamate levels in the CSF and brain subregions of MDD patients (CitationHashimoto et al., 2007; CitationMadeira et al., 2018; , glutamate excitotoxicity section). In consistent, rodent studies demonstrated inducement of depressive-like behaviors in mice and rats, by injections of GLT-1 inhibitors WAY-213613 into the lateral habenula (LHb) (CitationLyu et al., 2021), and dihydrokainic acid into multiple brain subregions, including the LHb (CitationCui et al., 2014), lateral ventricle (CitationBechtholt-Gompf et al., 2010), infralimbic cortex (CitationGasull-Camós et al., 2017), central amygdala (CitationJohn et al., 2015) and PFC (CitationJohn et al., 2012). Moreover, CUMS exposure reduced astrocytic GLT-1 expression in the PFC and hippocampus, and induced depressive-like behaviors in rats and mice (CitationLiu et al., 2019; CitationYang et al., 2020; , glutamate excitotoxicity section). Furthermore, antidepressants fluoxetine and Chinese herbal formula Xiao-Yao-San reversed depressive-like behaviors, through upregulating the CUMS inhibited GLT-1 expression in mice (CitationDing et al., 2017; CitationLiu et al., 2019). In addition, the glutamatergic signal inhibitory drugs alleviated depressive-like behaviors, such as the GLT-1 promoting drug Riluzole (CitationBanasr et al., 2010), and a variety of NMDA receptor antagonists (CitationAutry et al., 2011). However, NMDA receptor antagonists, such as phencyclidine and dizocilpine, could impair cognitive function, which precludes its utilization as antidepressants (CitationB. Zhang et al., 2019; CitationZhang et al., 2017). Meanwhile, elevated O-linked-N-acetylglucosaminylation (O-GlcNAcylation), in the mPFC of mice, had antidepressant effect (CitationCho et al., 2020). However, a recent study found the mRNA of O-GlcNAc transferase (OGT), a key enzyme in protein O-GlcNAcylation, was increased in MDD patients and in mPFC astrocytes of CSDS susceptible mice (CitationFan et al., 2023). Moreover, the OGT overexpression increased CSDS susceptibility of mice, whereas the OGT knockout reduced the astrocytic GLT-1 GlcNAcylation, and resulted in antidepressant effect (CitationFan et al., 2023). The results indicate the prominent role of mPFC O-GlcNAcylation in the modulation of depression, however, the detailed underlying mechanisms and the potential differential roles of neurons and astrocytes (e.g., astrocytic GLT-1) require further in-depth investigations for clarification (CitationFan et al., 2023; CitationPaton & Menard, 2023). The above evidence indicates central glutamate excitotoxicity, caused by GLT-1 inhibition disrupted astrocytic reuptake of synaptic glutamate, as an important pathogenesis of depression. The results further suggest GLT-1 modulation as a potentially promising antidepressant pathway.
Studies have found mutual interaction between glutamate excitotoxicity and OS (-OS). Excess ROS promotes glutamate excitotoxicity, through inhibiting the astrocytic GLT-1 expression and affecting Ca2+ and glutamine synthetase (CitationDallas et al., 2007; CitationVolterra et al., 1994). In turn, excess glutamate exacerbates OS by impairing mitochondria and promoting the ROS generation, through activating NMDA and triggering Ca2+ influx (CitationAtlante et al., 2001). As supporting evidence, in vitro studies showed ROS generation was produced upon NMDA receptor activation (CitationLafon-Cazal et al., 1993; CitationReynolds & Hastings, 1995), which was dependent on mitochondria polarization, induced by glutamate caused Ca2+ influx that also reduced ATP generation (CitationCastilho et al., 1999; CitationPereira & Oliveira, 2000; CitationReynolds & Hastings, 1995). Furthermore, interaction between OS and neuroinflammation might be required for the promoting effect, as oxide synthase and NOX inhibitors can block the process (CitationBarger et al., 2007; CitationHu et al., 2000; CitationTilleux & Hermans, 2007), and hypoxia affected GLT-1 function required the present of TNF, which could cause glutamate excitotoxicity by itself (CitationBoycott et al., 2008; CitationClark & Vissel, 2016). For the interaction between neuroinflammation and glutamate signaling (), neuroinflammation increases microglial glutamate release as mentioned above, through affecting the expression and activity of several regulatory proteins, such as downregulating the GLT-1 (CitationHaroon & Miller, 2017; CitationTilleux & Hermans, 2007), increasing the GLS (CitationChen et al., 2012; CitationTakeuchi et al., 2006), or promoting the QUIN generation (Citationde Oliveira et al., 2021; CitationVisentin et al., 2020). In turn, glutamate might regulate the microglial release of pro-inflammatory cytokines, in a receptor subtype-dependent manner (CitationMurugan et al., 2013). In vitro studies, performed in the primary microglial cultures, demonstrated that glutamate promoted the TNF-α and IL-1β expressions through α-amino-3-hydroxy-5-methyl-4-isoxazolepropionic (AMPA) receptor activation (CitationSivakumar et al., 2010), while the NMDA inhibition reduced the hypoxia-induced TNF-α and IL-1β expression (CitationMurugan et al., 2011), and the metabotropic glutamate receptor 5 (mGluR5) activation significantly reduced TNF-α generation (CitationByrnes et al., 2009). The findings might suggest glutamate upregulates pro-inflammatory cytokine expressions in activated microglia through ionotropic NMDA/AMPA receptors, whereas downregulates the expression through mGluRs.
Central BDNF/TrkB Dysfunction
Studies found glutamate quickly promoted BDNF expression in vitro, as reflected by increased levels of BDNF mRNA and protein (CitationH. Wu et al., 2004; CitationJean et al., 2008). BDNF is a major type of neurotrophic factor in the brain, released by different types of cells (e.g., endothelial cells, microglia and megakaryocytes), which is critically involved in diversified functions of the brain, such as maintaining neuronal plasticity and regulating mental state (CitationLin & Huang, 2020). BDNF concentration is significantly higher in the serum than in the plasma and CSF, as over 90% is stored in blood platelets (CitationFujimura et al., 2002; CitationNaegelin et al., 2018). Early clinical studies found significantly reduced BDNF in the serum and plasma of MDD patients (CitationKarege et al., 2005; CitationLee & Kim, 2008; , BDNF/TrkB dysfunction section), probably caused by reduced platelet release (CitationSerra-Millàs, 2016). In the brain, the gene expression and protein synthesis of BDNF and TrkB receptors have been found in multiple subregions, especially those closely related to depression (e.g., the PFC, hippocampus, hypothalamus) (CitationBrigadski & Leßmann, 2020). BDNF evaluation in the human brain could only be acquired by analyzing postmortem brain tissues, which demonstrates subregion specific BDNF alternations in MDD patients, with the findings of reduced BDNF mRNA and protein in the PFC and hippocampus, but increased BDNF expressions in the nucleus accumbens (NAc) and amygdala (CitationAutry & Monteggia, 2012; CitationBrigadski & Leßmann, 2020; CitationZhang et al., 2016). Animal studies further showed BDNF concentrations in mouse serum and plasma were too low to be detected, which probably attributes to the lack of BDNF gene in mouse megakaryocytes, and the consequential insufficient BDNF protein synthesis (CitationChacón-Fernández et al., 2016). Consistent with the clinical findings, rodent studies found CUMS and CSDS impaired the BDNF signaling in the PFC and hippocampus, and induced depressive-like behaviors in rats and mice, respectively (CitationMao, Ip et al., 2009; CitationMao, Xian et al., 2010; CitationZhang et al., 2015; , BDNF/TrkB dysfunction section). Recent studies further found microglial BDNF depletion in the PFC increased the mouse susceptibility to CUMS-induced depressive-like behaviors (CitationWoodburn et al., 2023), and the BDNF-TrkB signaling was critically involved in thalamic-primary auditory cortex circuit mediated resilience to CSDS in mice (CitationLi et al., 2023). Furthermore, rodent studies showed multiple antidepressants take effect through increasing the BDNF expression, or directly binding to TrkB receptors (CitationP. C. Casarotto et al., 2021; CitationP. Casarotto et al., 2022). For instance, ketamine elevated the BDNF expression in the mPFC and hippocampus, which effect was suggested to be required for the behavioral actions of ketamine and its metabolite (2R, 6R)-hydroxynorketamine (CitationFan et al., 2021; CitationFukumoto et al., 2019; CitationLepack et al., 2014; , BDNF section). The downstream pathways of TrkB receptors mainly involve the PI3K, phospholipase C-γ (PLC-γ) and ERK signals (CitationKozisek et al., 2008). The above evidence indicates the critical contribution of BDNF/TrkB dysfunction to depression onset, and the requirement of BDNF expression and TrkB activation for antidepressant effect (CitationCasarotto et al., 2022; CitationCastren & Monteggia, 2021; , –, BDNF/TrkB dysfunction section).
Studies found mutual interaction between BDNF/TrkB signaling and OS, and neuroinflammation, respectively (-OS, BD). For BDNF-OS interaction, excess ROS inhibits the BDNF gene expression and protein synthesis, through reducing the CREB activity and increasing the NF-κB binding activity (CitationA. Wu et al., 2004; CitationKapczinski et al., 2008; CitationZou & Crews, 2006). In turn, BDNF has antioxidant effect and protects neurons against OS, through TrkB, ERK 1/2 and NF-κB/sestrin2, thus BDNF deficiency might aggravate OS (CitationS. D. Chen et al., 2017). For the interaction with neuroinflammation, BDNF promotes neuroinflammation through activating astrocytes and microglia to release TNF-α and IL-1β (CitationDing et al., 2020). In turn, LPS significantly reduced the BDNF protein level in mouse PFC and hippocampus, whereas increased the expression in the NAc (CitationGuan & Fang, 2006; CitationZhang et al., 2014), probably through activating microglial NF-κB that interacts with the BDNF/TrkB signaling (CitationLi et al., 2022). The prominent role of interaction between BDNF/TrkB and NF-κB in depression has been previously described (CitationCaviedes et al., 2017). With regard to the BDNF-glutamate signaling interaction (), in vitro studies found glutamate (100 μM and 100 mM) promoted the BDNF expression and secretion (CitationH. Wu et al., 2004; CitationJean et al., 2008). Moreover, the higher glutamate dosage (1.0 mM) induced more BDNF release than the lower dose (0.1 mM), which suggests dose-dependent promoting effect (CitationTaylor et al., 2003). However, the effect of toxic dosage glutamate on BDNF expression, in vitro and in vivo, remains unclear and requires further clarification. Excess glutamate might bind to the extra-synaptic NMDA receptors and activate eukaryotic elongation factor 2 (eEF2) kinase, which phosphorylates eEF2 that inhibits the BDNF expression, functioning in the opposite direction of NMDA antagonists, considering the prodepressant role of glutamate excitotoxicity, antidepressant role of NMDA blockage, and enhanced BDNF in the PFC and hippocampus (CitationAutry et al., 2011; CitationBjorkholm & Monteggia, 2016; CitationMurrough et al., 2017). In turn, BDNF protected neurons and glia cells against glutamate excitotoxicity in vitro, which effect involved Ca2+ homeostasis, cytokine expressions and related sparse representation-based classification (SRC) signals (CitationGaidin et al., 2020). The protection effect partially attributes to the BDNF upregulated astrocytic GLT-1 expression, via p42/p44 mitogen-activated protein kinase signals (ERK1/2) utilizing NF-κB (CitationRodriguez-Kern et al., 2003). In addition, the protection effect was blocked by inhibitors of ERK, PI3K and PLC-γ, which indicated vital involvement of the signals in the process (CitationAlmeida et al., 2005; CitationKume et al., 2000; CitationMelo et al., 2013). In contrast, BDNF also rapidly and temporarily increased glutamate release in cultured neurons and astrocytes, which process was blocked by K252a and PP2 (specific inhibitors of TrkB and SRC, respectively), indicating the requirement of TrkB/SRC/PLC-γ1 pathway activation for the effect (CitationPascual et al., 2001; CitationZhang et al., 2013). Studies also demonstrated BDNF-promoted glutamate release, through activating the PLC-γ/IP3 pathway and inducing transient Ca2+ increase (CitationNumakawa et al., 2001), and moreover, antidepressants imipramine and fluvoxamine potentiated the BDNF-promoted PLC-γ/IP3/Ca2+ signaling and glutamate release via sigma-1 receptors (CitationYagasaki et al., 2006). Additionally, a recent study found microglial BDNF promoted the NMDA receptor expression in the PFC of mice, further indicating the BDNF-promoted glutamatergic signaling (CitationWoodburn et al., 2023). The above evidence might suggest BDNF modulates glutamate homeostasis in the brain, in a way that it promotes glutamate release under physiological condition (but only to limited extent), however, protects neurons and glia cells against glutamate excitotoxicity. The BDNF dysregulation would attenuate the promoted glutamate release or the excitotoxicity, correspondingly.
Despite of the well-recognized role of BDNF, the direct involvement of TrkB receptors in depression still requires further clarification. Limited research so far found TrkB inhibition caused resistant to antidepressants in TrkB.T1-overexpressing transgenic mice (CitationSaarelainen et al., 2003), while K252a inhibited TrkB activation blocked ketamine’s antidepressant effect in CUMS exposed rats (CitationLiu et al., 2016). Moreover, TrkB activation, by administration of the agonist 7,8-Dihydroxyflavone in the PFC and hippocampus, had antidepressant effect through increasing the p-TrkB level, while the TrkB inhibitor ANA-12 administration in NAc exerted antidepressant effect by reducing the p-TrkB level, in depressed rats and mice caused by learned helplessness, CSDS and LPS administration, respectively (CitationShirayama et al., 2015; CitationZhang, Wu et al., 2014; CitationZhang, Yao et al., 2015). The brain subregion specific p-TrkB alternation is consistent with the regional BDNF abnormalities. More importantly, together with the findings that antidepressants can directly bind to TrkB receptors to produce antidepressant effect, results suggest the possibility of modulating depression phenotype through directly regulating TrkB expression and activation (CitationP. C. Casarotto et al., 2021; CitationP. Casarotto et al., 2022). The direct influence of OS, neuroinflammation and glutamate excitotoxicity on TrkB expression and activation is yet to be determined. The evidence in the present section indicates BDNF/TrkB dysfunction as a vital pathogenesis of depression, and moreover, suggests the BDNF/TrkB signaling physiological homeostasis restoration as a promising therapeutic avenue of the disease.
Central 5-HT Deficiency
The central monoamine deficiency, especially the 5-HT deficiency, is the classical depression hypothesis, based on which most first-line clinical antidepressants have been developed and widely utilized, such as tricyclic antidepressants (TCAs), monoamine oxidase inhibitors (MAOIs), and selective serotonin reuptake inhibitors (SSRIs), which take effects through upregulating the synaptic concentrations of monoamines (CitationMalhi & Mann, 2018; CitationMoncrieff et al., 2022). Over 90% of systematic 5-HT is synthesized by intestinal chromaffin cells, which can not cross the BBB under physiological condition, whereas its primary precursor tryptophan can (CitationCorreia & Vale, 2022; CitationGheorghe et al., 2019). Tryptophan could be metabolized to 5-HT, catalyzed by TPH1/AAAD in the gut and TPH2/AAAD in the brain, or alternatively, metabolized to kynurenine, catalyzed by IDO1/TDO in the gut and IDO in the brain (CitationGheorghe et al., 2019; CitationKrautkramer et al., 2021). Thus, the enhanced IDO1/TDO activity would promote KP and inhibit 5-HT pathway, resulting in increased kynurenine and reduced 5-HT concentration, respectively. Gut inflammation would activate the IDO1/TDO activity and promote peripheral KP, which reduces tryptophan available to cross the BBB for central 5-HT production, while neuroinflammation enhanced IDO activity promotes central KP and directly inhibits 5-HT generation in the brain (CitationChen et al., 2023; CitationDeng et al., 2021; CitationWang et al., 2022).
The central 5-HT could interact with OS directly, or indirectly through neuroinflammation, central glutamate and BDNF/TrkB signaling, and the MGB axis. For the interaction between 5-HT and OS (-OS), studies found 5-HT presented antioxidant properties in vitro, as indicted by the inhibited generations of ROS and iNOS (CitationVašíček et al., 2020), while N-acetyl 5-HT showed antioxidant and anti-apoptosis actions, by activating TrkB/CREB/BDNF pathway and antioxidant enzyme expression, in vitro and in vivo (CitationYoo et al., 2017). Recent studies demonstrated the possibility of designing fast-onset antidepressant, by dissociating 5-HTT from neuronal NOS in the DRN of mice, which indicates the critical involvement of 5-HT and OS interaction in depression pathogenesis (CitationGuan & Pang, 2023; CitationKingwell, 2022; CitationSun et al., 2022; CitationYe et al., 2023). In turn, OS could cause central 5-HT deficiency through inducing neuroinflammation (CitationCatena-Dell’Osso et al., 2013) and MGB disturbance (CitationStasi et al., 2019), by activating IDO/TDO enzymes and promoting KP of tryptophan metabolism, as expounded in the above sections correspondingly. For 5-HT and neuroinflammation interaction (), pro-inflammatory cytokines, such as TNF-α and IL-1β, modulated the expression and function of serotonin transporter (SERT/5-HTT) (CitationMalynn et al., 2013; CitationZhu et al., 2006). With regard to 5-HT and glutamate interaction (), studies showed 5-HT receptors mediated the serotonergic inhibition of glutamate transmission in multiple brain subregions, through the 5-HT1B receptor inhibited presynaptic glutamate release, or the 5-HT1A receptor reduced postsynaptic glutamate receptor sensitivity (CitationGuo & Rainnie, 2010; CitationNishijo et al., 2022). In turn, vesicular glutamate transporter 1 (VGLUT1)-dependent glutamate innervation of dorsal raphe nucleus (DRN) modulated the 5-HT function, as the VGLUT1+/− mice presented decreased 5-HT neuronal activity and hippocampal 5-HT turnover (CitationGarcía-García et al., 2013). The glutamatergic drive of the DRN, which contains majority of 5-HT neurons in the brain, has been previously addressed (CitationSoiza-Reilly & Commons, 2011). The involvement of 5-HT and BDNF/TrkB interaction () in depression has been previously addressed (CitationPopova & Naumenko, 2019). 5-HT receptor activation with agonists promoted the BDNF expression and TrkB phosphorylation, in vivo and in vitro, therefore, the central 5-HT deficiency would lead to reduced BDNF expression and TrkB activation (Citationde Foubert et al., 2007; CitationPascual-Brazo et al., 2012; CitationSamarajeewa et al., 2014). In turn, BDNF dose-dependently reduced 5-HT reuptake through affecting the 5-HTT function, with the higher doses presenting more severe reuptake inhibition, in vitro (CitationMössner et al., 2000). However, intrahippocampal BDNF injection reduced extracellular 5-HT concentration in mice, which effect was blocked by the TrkB antagonist K252a, suggesting TrkB activation-dependent BDNF-promoted 5-HTT function (CitationBenmansour et al., 2008). BDNF had no significant influence on the 5-HTT density in rat hippocampus (CitationBenmansour et al., 2008), which was consistent with the finding of BDNF-dependent 5-HTT function, but not expression, in mice (CitationDaws et al., 2007). Furthermore, loss of BDNF gene allele increased stress abnormalities and exacerbated monoamine deficiencies in the 5-HTT knockout mice (CitationRen-Patterson et al., 2005), and down-regulated the 5-HTT function in mouse hippocampus (CitationDaws et al., 2007; CitationDeltheil et al., 2008). The results suggest BDNF affected 5-HTT function, rather than the expression, at least in the hippocampus of mice. For the 5-HT and MGB interaction (), microbiota disturbance could induce central 5-HT deficiency, through causing gut inflammation that activates IDO1/TDO and promotes peripheral KP, which reduces tryptophan available for central 5-HT production (CitationChen et al., 2023; CitationWang et al., 2022), or affecting the expressions of TPH1 (CitationReigstad et al., 2015) and 5-HT-related genes (CitationBorrelli et al., 2016). In turn, the 5-HTT genotype could modulate the microbiota composition in rats (CitationEl Aidy et al., 2017). Moreover, the gene expression pattern of MS exposed rats resembled the pattern of rats with reduced 5-HTT gene expression, in the PFC and hippocampus, which indicates the prominent role of 5-HTT in stress response (CitationComasco et al., 2019). The evidence in present section indicates close relationship between the classical central 5-HT deficiency and OS, neuroinflammation, central glutamate and BDNF/TrkB dysregulation, and the MGB disturbance. Further exploring the connections between the classical and novel pathogenesis would definitely benefit the incoming studies, by providing deep insights of the intricate underlying mechanisms, and novel targets and fresh ideas for depression treatment.
The MGB Axis Disturbance
The MGB axis refers to the connection and mutual influence between the brain and gastrointestinal system, with intestinal microbiota composition (abundance and diversity) and metabolism as the key component. Microbiota affect brain function through tryptophan metabolism, vagus nerve, immune regulation, etc., while brain in turn influences microbiota through immune modulation, neuroendocrine regulation, etc. (CitationKundu et al., 2017). The close relationship between the MGB disturbance and depression has been extensively attended in recent years (CitationMcGuinness et al., 2022; CitationWinter et al., 2018) (, –, MGB disturbance section). As supporting evidence, studies found disturbed microbiota composition and metabolism in depressed patients and animals, however, with both increased and reduced abundance and diversity been revealed across microbiota species/genera (mainly Firmicutes and Bacteroidetes) (CitationBarandouzi et al., 2020; CitationDe Palma et al., 2015; CitationGao et al., 2022; CitationQu et al., 2017; CitationSanada et al., 2020). Clinical studies found reduced abundance of Bifidobacteria and Lactobacilli, but increased abundance of Bacteroidea, Proteus and Actinobact eria, in MDD patients (CitationBarandouzi et al., 2020; CitationSanada et al., 2020; , MGB axis disturbance section). Rodent studies revealed significantly reduced abundance of Bacteroidales and Clostridiales in CSDS and social disruption treated mice (CitationBailey et al., 2011; CitationQu et al., 2017), and reduced abundance of Lactobacillus in chronic variable stress exposed rats (CitationM. Yu et al., 2017) and CSDS susceptible mice (CitationX. Zhu et al., 2023). In contrast, the abundance of bacteria in the genus Clostridium and Lactobacillus were increased in social disruption and MS treated mice, respectively (CitationBailey et al., 2011; CitationDe Palma et al., 2015) (, MGB axis disturbance section). The inconsistency could attribute to diversified microbiota species/genera and individual genetic diversity, influenced by variables of subject gender and age, stress subtype, dynamic microbiota changes across development, constant influence of daily diet, etc. On the other hand, microbiota disturbance, induced by antibiotic treatment or fecal microbiota transplantation (FMT) from CUMS exposed subjects to control recipients, resulted in depressive-live behaviors in rats and mice, respectively (CitationHoban et al., 2016; CitationKelly et al., 2016; CitationLi et al., 2019). Furthermore, microbiota homeostasis restoration and depressive-live behavior amelioration have been achieved in mice, through antidepressant treatments (e.g., ketamine, fluoxetine and Chinese medicine formula Kai-Xin-San) (CitationCao et al., 2020; CitationLukic et al., 2019; CitationQu et al., 2017), probiotic administrations (Bifidobacterium, Lactobacillus rhamnosus and Lactococcus lactis) (CitationBravo et al., 2011; CitationGao et al., 2022; CitationYang et al., 2017), dechlorogenic sunflower seeds and tryptophan supplementation (CitationLu et al., 2022; CitationWang et al., 2022), and FMT from NLRP3 KO mice to CUMS treated mice (CitationY. Zhang et al., 2019). The above evidence indicates the vital role of microbiota disturbance in depression onset, and suggests microbiota homeostasis restoration as an appealing antidepressant approach.
Studies have found close interplay between microbiota and OS/mitochondria, which might play prominent roles in depression (CitationShandilya et al., 2022) (). Microbiota regulates ROS level through affecting the function and activities of mitochondria, short chain fatty acids (SCFAs), antioxidants and NOX, which are enriched in the intestinal tract (CitationAviello & Knaus, 2018; CitationBallard & Towarnicki, 2020; CitationJones & Neish, 2017). For example, lactic acid derived from Lactobacillus plantarum activated the intestinal NOX to promote ROS generation (CitationIatsenko et al., 2018), while molecules containing indole and SCFAs improved bacterial metabolism and reduced the ROS production, through restoring mitochondrial function and activating antioxidant activity, respectively (CitationBallard & Towarnicki, 2020). Furthermore, studies indicated gut microbiota as the vital mediator of OS, whose disturbance impaired mitochondria, reduced ATP production and increased ROS generation, partially through the metabolite N6-carboxymethyllysine in central microglia of mice (CitationBrunt et al., 2019; CitationMossad et al., 2022). In addition, the probiotic Bifidobacterium infantis increased plasma tryptophan in rats, and tryptophan supplementation improved central mitochondrial metabolism and attenuated depressive-like behaviors in CUMS exposed mice, which results indicate critical involvement of tryptophan in microbiota affected mitochondrial function and depressive symptoms (CitationDesbonnet et al., 2008; CitationWang et al., 2022). In turn, mitochondrial genotype and mutation significantly influenced microbiota composition through ROS regulation (CitationYardeni et al., 2019).
Studies have revealed mutual influence between microbiota and peripheral/gut and central/neuron inflammation, respectively. The interaction between microbiota and gut inflammation involves multiple molecular mechanisms, such as the microbiota-shaped T cell subsets and generated SCFAs, immune-related dendritic cells and immunoglobulin A, etc. (CitationBelkaid & Hand, 2014; CitationHooper et al., 2012; CitationRatajczak et al., 2019). For microbiota-neuroinflammation interaction (), microbiota disturbance leads to systemic inflammation, reduces SCFAs, and increases peripheral IL-6 and IFN-γ, which activate microglia and promote the enzymes of IDO/TDO/KMO to generate QUIN that damages BBB and elevates IL-6, IL-1β, and NLRP3 in the brain, finally resulting in neuroinflammation (CitationCarlessi et al., 2021). On the other hand, probiotics have anti-inflammatory and antidepressant effects. Probiotic inulin reversed age-related neuroinflammation in mice, mainly including monocyte infiltration and microglial activation (CitationBoehme et al., 2020). Bifidobacterium infantis significantly increased tryptophan concentrations, and reduced forced swim stress enhanced IL-6, IFN-γ and TNF-α levels in rat plasma (CitationDesbonnet et al., 2008), while Bifidobacterium adolescentis and Parabacteroides reduced the CRS enhanced IDO activity and increased levels of IL-1β, TNF-α and NF-κB in mouse hippocampus (CitationDeng et al., 2021; CitationY. Guo et al., 2019), which all reversed the rodent depressive-like behaviors. Additionally, studies suggested the antidepressant and anti-inflammatory effects of ketamine was mediated via its interaction with a variety of gut microbiota, such as the Lactobacillus, Actinobacteria, etc. (CitationGetachew et al., 2018; CitationN. Huang et al., 2019). In turn, neuroinflammation could disturb microbiota homeostasis, primarily through the NLRP3, pro-inflammatory cytokines and ROS, whose underlying mechanisms need to be further uncovered (CitationCarlessi et al., 2021; CitationInserra et al., 2018). Furthermore, recent studies have revealed the crucial roles of microbiota-gut-immune-glia axis and glia-neuron communication in depression, which could potentially integrate the gut microbiota, immune signals, and central glia-neuron communication into a network, responsible for depression onset and remedy (CitationRudzki & Maes, 2020, Citation2021).
The MGB disturbance affects neurotransmitter homeostasis in the brain, especially inducing the BDNF/TrkB dysfunction and 5-HT deficiency (). For the MGB-BDNF interaction (), studies found chronic stress (CUMS and CRS)-induced microbiota disturbance and depressive-like behaviors in mice, through reducing the hippocampal BDNF expression, which were ameliorated by the restored BDNF expression, caused by tryptophan supplementation and probiotic treatments (e.g., Parabacteroides and Bifidobacterium adolescentis) (CitationBercik et al., 2011; CitationDing et al., 2021; CitationWang et al., 2022; CitationY. Guo et al., 2019). The direct influence of MGB disturbance on TrkB expression and activation remains unclear. In turn, BDNF modulates the intestinal barrier integrity, through regulating tight junction protein expression and inhibiting intestinal epithelial cell apoptosis, whose dysfunction would impair the integrity and further disturbs the microbiota homeostasis (CitationY. B. Yu et al., 2017; CitationZhao et al., 2018). The MGB-BDNF/TrkB interaction might play more important roles in depression than we expected, however, more extensive and in-depth investigations are required to further validate their critical involvement, and reveal the detailed underlying mechanisms. With regard to the central 5-HT deficiency, considerable evidence has demonstrated its pivotal role in depression, as described in the above section (CitationMoncrieff et al., 2022; CitationVahid-Ansari & Albert, 2021). Studies have found interactions between the 5-HT, gut microbiota and the MGB axis (CitationStasi et al., 2019) (). Microbiota metabolites SCFAs increased the TPH1 mRNAs in vitro (CitationReigstad et al., 2015), while Bifidobacterium infantis reduced the concentration of 5-HIAA (the primary metabolite of 5-HT) in the PFC of rats (CitationDesbonnet et al., 2008), and Lactobacillus rhamnosus increased the expression of 5-HT-related genes (e.g., TPH1a, TPH2, and slc6a4a) in the brain of zebrafish (CitationBorrelli et al., 2016). For the involvement of the interaction in depression, studies found microbiota disturbance induces gut and central inflammation, activates the IDO/TDO enzymes and promotes peripheral and central KP, which reduces peripheral tryptophan available to cross the BBB and directly inhibits central 5-HT production, respectively. Those alternations were reversed by tryptophan supplementation and IDO/TDO inhibition, along with the depressive-like behaviors in rodents. As supporting evidence, CUMS damaged gut barrier integrity, promoted peripheral IDO activity and KP, reduced 5-HT in the serum, and induced depressive-like behaviors in mice, which were reversed by tryptophan supplementation and lentivirus-mediated IDO knockdown, respectively (CitationChen et al., 2023; CitationWang et al., 2022). In addition, CUMS and CRS-promoted neuroinflammation, reduced hippocampal 5-HT, and induced depressive-like behaviors in mice, which were reversed by the IDO inhibitor 1-methyl-tryptophan and tryptophan supplementation (CitationDeng et al., 2021; CitationWang et al., 2022). The results indicate the vital role of tryptophan metabolism in the interplay between microbiota and peripheral/central inflammation. In turn, 5-HTT genotype modulated microbiota composition in rats (CitationEl Aidy et al., 2017). The evidence in the present section indicates the vital role of MGB disturbance in depression, which involves the mutual interaction with OS and mitochondrial impairment, neuroinflammation, central BDNF/TrkB dysregulation and 5-HT deficiency, respectively. The evidence also suggests MGB homeostasis restoration as a promising antidepressant avenue.
The HPA Axis Dysregulation
The HPA axis dysregulation is another classical depression hypothesis being extensively attended, and both clinical and animal studies have found depression,related HPA hyperactivity or hypoactivity, and the consequential hypercortisolism and hypocortisolism, respectively (CitationMenke, 2019; CitationZhang, 2017). To simply describe the axis activity, the hypothalamus secretes corticotropin-releasing factor (CRF) that promotes the pituitary release of adrenocorticotropic hormone (ACTH), which activates the adrenal cortex release of glucocorticoids. Glucocorticoid receptors (GRs) are widely distributed in in the brain, especially depression-related subregions (e.g., PFC, hippocampus, and hypothalamus), whose activation is responsible for feedback inhibition of the CRH/ACTH release, and restoration of the HPA axis homeostasis. The dysfunction of GRs, especially the reduced expression and desensitization, would disrupt the feedback loop and result in hypercortisolism that is commonly and critically involved in depression (CitationMenke, 2019).
The HPA axis interacts with OS directly, or indirectly through neuroinflammation, central glutamate and BDNF/TrkB signaling, and the MGB axis. For the HPA-OS interaction (-OS), studies found NO mediated the cholinergic stimulation of HPA axis, and the IL-1β-induced HPA response in social crowding stress exposed rats (CitationBugajski et al., 2006; CitationGadek-Michalska & Bugajski, 2005). In turn, cortisol-induced ROS generation through membrane GRs (CitationEspinoza et al., 2017), which probably attributes to translocation of the activated GRs to mitochondria that results in enhanced calcium buffering capacity (CitationDu et al., 2009; CitationPsarra & Sekeris, 2009). Furthermore, mitochondrial GR activation could directly regulate mitochondrial energy metabolism and oxidative phosphorylation gene expression (CitationKokkinopoulou & Moutsatsou, 2021). With regard to the HPA-neuroinflammation interaction (), studies have found activated GRs could translocate to nucleus, to directly inhibit pro-inflammatory cytokine gene expressions (CitationAdcock et al., 2005; CitationCain & Cidlowski, 2017). The pro-inflammatory gene expression was increased in the GR depleted hippocampal microglia in mice reared under standard condition, but was reduced in the CUMS exposed mice, which suggests differential regulating effect of GRs under normal and stressful conditions (CitationPicard et al., 2021). Another study further revealed the prominent role of interaction between GR and NF-κB in regulating hippocampal inflammatory responses, in the CUMS exposed rats (CitationLi et al., 2021). Alternatively, GRs could indirectly take effect through OS, as GR agonist compound K regulated IL-6 and TNF-α expressions, through inhibiting the ROS generation (CitationCuong et al., 2009). In turn, cytokine affected GR function is closed related to glucocorticoid resistance in depression (CitationPace et al., 2007), and pro-inflammatory cytokines target at hypothalamus to promote glucocorticoid secretion, which in turn activates GRs that inhibit cytokine expressions, which loop links neuroinflammation to the axis activity (CitationCain & Cidlowski, 2017). For HPA-glutamate interaction (), in vitro studies showed activated GRs interacted with TrkB to facilitate the BDNF-promoted glutamate release via PLC-γ signaling (CitationNumakawa et al., 2009), while glucocorticoids attenuated the BDNF-dependent glutamate receptor upregulation (CitationKawashima et al., 2010). Moreover, chronic HPA axis disruption with corticosterone altered glutamate signaling-related gene expressions in mice, in a way that corticosterone increased mRNA expression of ionotropic glutamate receptor subunits GluR1 and GluR2 in the mPFC, reduced mRNA expression of the NMDA receptor subunits (NR1, NR2B) and mGluR5 in the hippocampus, and decreased mRNA expression the AMPA receptor subunit GluR1 in the amygdala (CitationKinlein et al., 2022). In turn, glutamate signaling plays a complex role in affecting the HPA responses, probably through driving acute HPA response via the ionotropic receptors postsynaptically, and inhibiting CRH neurons via group I mGluR presynaptically (CitationEvanson & Herman, 2015b). For instance, paraventricular nucleus injection of group I mGluR agonist blunted, whereas the antagonist potentiated, the HPA response to restraint stress in rats (CitationEvanson & Herman, 2015a). For the HPA-BDNF interaction (), studies showed that GR activation down-regulated the BDNF expression in vitro (CitationChen et al., 2019; CitationH. Chen et al., 2017), while BDNF polymorphism disrupted the GR phosphorylation, through which central BDNF signaling rewrote the glucocorticoid transcriptome (CitationArango-Lievano et al., 2019; CitationLambert et al., 2013). The critical involvement of HPA-MGB interaction in depression has been previously indicated (CitationFreimer et al., 2022) (). For example, stress activated HPA axis and consequentially generated hormones (e.g., glucocorticoids and catecholamines) increased the intestinal barrier permeability (CitationZheng et al., 2013) and disturbed microbiota composition (CitationMoussaoui et al., 2017). In turn, microbiota homeostasis restoration with the probiotic L. farciminis attenuated the LPS-induced gut leakiness, HPA response and neuroinflammation in rats (CitationAit-Belgnaoui et al., 2012). Moreover, Lactobacillus rhamnosus restores central GABAergic activity to attenuate the HPA hyperactivity and exert antidepressant effect (CitationTette et al., 2022). The evidence in the present section indicates close association between the classical HPA axis dysregulation and novel depression pathogenesis, including OS, neuroinflammation, central glutamate and BDNF/TrkB dysregulation, and the MGB disturbance. The integration of the classical and novel pathogenesis surely would provide us with an integral overview, and supply us with more in-depth insights, of the intricate pathogenesis of the disease.
Concluding Remarks
Depression increasingly and seriously affects the mental and physical health of people worldwide. To uncover the intricate disease pathogenesis, several animal models (especially in rodents) have been established, through acute and chronic stress exposures, olfactory bulbectomy, drug administration (e.g., LPS and corticosterone), genetic manipulation, etc. (CitationCzéh et al., 2016; CitationZhang, 2017). Through years of clinical and animal investigations, a variety of disease hypotheses have been proposed, such as the classical central 5-HT deficiency (CitationMoncrieff et al., 2022) and HPA axis dysregulation (CitationMenke, 2019), and the relative novel neuroinflammation (CitationTroubat et al., 2021), glutamate excitotoxicity (CitationMurrough et al., 2017), BDNF dysfunction (CitationCastren & Monteggia, 2021) and MGB axis disturbance (CitationWinter et al., 2018). With regard to the existing first-line antidepressants, over a third of MDD patients do not have proper response, referred as treatment-resistant depression (TRD). Classical antidepressants usually target on the monoamine system and elevate their synaptic concentration in the brain, such as the TCAs, MAOIs and SSRIs, which generally require at least two weeks of continuous treatment, and moreover, have a variety of side effects (CitationCipriani et al., 2018; CitationMalhi & Mann, 2018). However, recent studies demonstrated the possibility of designing fast-onset monoamine-based antidepressants, by dissociating 5-HTT from neuronal NOS in the DRN of mice (CitationGuan & Pang, 2023; CitationKingwell, 2022; CitationSun et al., 2022; CitationYe et al., 2023). Another study further showed Propofol rapidly relieved the core depressive symptom anhedonia in mice, through inhibiting dopamine transporter (but not the 5-HTT) and evoking long-lasting dopamine accumulation in the NAc (CitationX. N. Zhu et al., 2023). There exist several other antidepressants targeting beyond the monoamine signaling, among which ketamine could rapidly and robustly alleviate depression (including TRD) with subanaesthetic doses, mainly through modulating central glutamatergic system, whose efficiency, however, has been shadowed by the addictive and psychotomimetic side effects (CitationBerton & Nestler, 2006; CitationRiggs & Gould, 2021). Thus, exploring novel pathogenesis of depression is necessary, for seeking more safe and efficient therapeutic treatments. In recent years, emerging evidence has revealed the vital involvement of OS in depression, however, the detailed underlying mechanisms remain unclarified. Considering the natural advantages of antioxidant treatments, such as antioxidant diet and exercise, which could avoid the side effect of drugs, it is appealing to pursue the antioxidant avenue of depression prevention and treatment. Fully uncovering how OS induces depression is the first step towards the avenue, thus we summarize and elaborate the possible downstream pathways mediating the OS influence, from the perspectives of mitochondrial impairment and related ATP deficiency, neuroinflammation, glutamate excitotoxicity, BDNF/TrkB dysfunction and 5-HT deficiency in the brain, the MGB disturbance and HPA dysregulation. We also expound the intricate interactions between the multiple aspects, and the molecular mechanisms mediating the interplay. Despite of what we have learned so far, more in-depth investigations are required to fully depict a comprehensive overview of how OS induces depression, in order to further provide fresh ideas and novel targets for the final goal of effective prevention and treatment of the disease.
Author Contributions
J. N., L. M. Z. and C. Y. T. wrote the draft manuscript and prepared the illustrations. T. S. W., L. C. Y. and Z. B. conceived and edited the manuscript and illustrations. All authors read and approved the final version of the manuscript.
Declaration of Conflicting Interests
The authors declared no potential conflicts of interest with respect to the research, authorship, and/or publication of this article.
ORCID iD
Funding
The authors disclosed receipt of the following financial support for the research, authorship, and/or publication of this article: This work was supported by the Natural Science Foundation of Guangxi Province (2020GXNSFAA297134), the Guangxi science and technology base and talent special project (GuiKe-AD20238023), the National Natural Science Foundation of China (32260200, 32160197), and the Guangxi Key Laboratory of Brain and Cognitive Neuroscience (GKLBCN-20190101).
References
- Adcock I. M., Cosio B., Tsaprouni L., Barnes P. J., Ito K. (2005). Redox regulation of histone deacetylases and glucocorticoid-mediated inhibition of the inflammatory response. Antioxidants & Redox Signaling, 7(1-2), 144–152. https://doi.org/10.1089/ars.2005.7.144
- Ait-Belgnaoui A., Durand H., Cartier C., Chaumaz G., Eutamene H., Ferrier L., Houdeau E., Fioramonti J., Bueno L., Theodorou V. (2012). Prevention of gut leakiness by a probiotic treatment leads to attenuated HPA response to an acute psychological stress in rats. Psychoneuroendocrinology, 37(11), 1885–1895. https://doi.org/10.1016/j.psyneuen.2012.03.024
- Ali S. S., Ahsan H., Zia M. K., Siddiqui T., Khan F. H. (2020). Understanding oxidants and antioxidants: Classical team with new players. Journal of Food Biochemistry, 44(3), e13145. https://doi.org/10.1111/jfbc.13145
- Allen J., Romay-Tallon R., Brymer K. J., Caruncho H. J., Kalynchuk L. E. (2018). Mitochondria and mood: Mitochondrial dysfunction as a key player in the manifestation of depression. Frontiers in Neuroscience, 12, 386. https://doi.org/10.3389/fnins.2018.00386
- Almeida R. D., Manadas B. J., Melo C. V., Gomes J. R., Mendes C. S., Grãos M. M., Carvalho R. F., Carvalho A. P., Duarte C. B. (2005). Neuroprotection by BDNF against glutamate-induced apoptotic cell death is mediated by ERK and PI3-kinase pathways. Cell Death & Differentiation, 12(10), 1329–1343. https://doi.org/10.1038/sj.cdd.4401662
- Arango-Lievano M., Borie A. M., Dromard Y., Murat M., Desarmenien M. G., Garabedian M. J., Jeanneteau F. (2019). Persistence of learning-induced synapses depends on neurotrophic priming of glucocorticoid receptors. Proceedings of the National Academy of Sciences, 116(26), 13097–13106. https://doi.org/10.1073/pnas.1903203116
- Atlante A., Calissano P., Bobba A., Giannattasio S., Marra E., Passarella S. (2001). Glutamate neurotoxicity, oxidative stress and mitochondria. FEBS Letters, 497(1), 1–5. https://doi.org/10.1016/s0014-5793(01)02437-1
- Autry A. E., Adachi M., Nosyreva E., Na E. S., Los M. F., Cheng P. F., Kavalali E. T., Monteggia L. M. (2011). NMDA receptor blockade at rest triggers rapid behavioural antidepressant responses. Nature, 475(7354), 91–95. https://doi.org/10.1038/nature10130
- Autry A. E., Monteggia L. M. (2012). Brain-derived neurotrophic factor and neuropsychiatric disorders. Pharmacological Reviews, 64(2), 238–258. https://doi.org/10.1124/pr.111.005108
- Aviello G., Knaus U. G. (2018). NADPH oxidases and ROS signaling in the gastrointestinal tract. Mucosal Immunology, 11(4), 1011–1023. https://doi.org/10.1038/s41385-018-0021-8
- Bai Z., Gao T., Zhang R., Lu Y., Tian J., Wang T., Zhao K., Wang H. (2023). Inhibition of IL-6 methylation by Saikosaponin C regulates neuroinflammation to alleviate depression. International Immunopharmacology, 118, 110043. https://doi.org/10.1016/j.intimp.2023.110043
- Bailey M. T., Dowd S. E., Galley J. D., Hufnagle A. R., Allen R. G., Lyte M. (2011). Exposure to a social stressor alters the structure of the intestinal microbiota: Implications for stressor-induced immunomodulation. Brain, Behavior, and Immunity, 25(3), 397–407. https://doi.org/10.1016/j.bbi.2010.10.023
- Ballard J. W. O., Towarnicki S. G. (2020). Mitochondria, the gut microbiome and ROS. Cellular Signalling, 75, 109737. https://doi.org/10.1016/j.cellsig.2020.109737
- Banasr M., Chowdhury G. M., Terwilliger R., Newton S. S., Duman R. S., Behar K. L., Sanacora G. (2010). Glial pathology in an animal model of depression: Reversal of stress-induced cellular, metabolic and behavioral deficits by the glutamate-modulating drug riluzole. Molecular Psychiatry, 15(5), 501–511. https://doi.org/10.1038/mp.2008.106
- Bansal Y., Kuhad A. (2016). Mitochondrial dysfunction in depression. Current Neuropharmacology, 14(6), 610–618. https://doi.org/10.2174/1570159x14666160229114755
- Barandouzi Z. A., Starkweather A. R., Henderson W. A., Gyamfi A., Cong X. S. (2020). Altered composition of gut microbiota in depression: A systematic review. Frontiers in Psychiatry, 11, 541. https://doi.org/10.3389/fpsyt.2020.00541
- Barger S. W., Goodwin M. E., Porter M. M., Beggs M. L. (2007). Glutamate release from activated microglia requires the oxidative burst and lipid peroxidation. Journal of Neurochemistry, 101(5), 1205–1213. https://doi.org/10.1111/j.1471-4159.2007.04487.x
- Bechtholt-Gompf A. J., Walther H. V., Adams M. A., Carlezon W. A.Jr., Ongur D., Cohen B. M. (2010). Blockade of astrocytic glutamate uptake in rats induces signs of anhedonia and impaired spatial memory. Neuropsychopharmacology, 35(10), 2049–2059. https://doi.org/10.1038/npp.2010.74
- Belkaid Y., Hand T. W. (2014). Role of the microbiota in immunity and inflammation. Cell, 157(1), 121–141. https://doi.org/10.1016/j.cell.2014.03.011
- Benmansour S., Deltheil T., Piotrowski J., Nicolas L., Reperant C., Gardier A. M., Frazer A., David D. J. (2008). Influence of brain-derived neurotrophic factor (BDNF) on serotonin neurotransmission in the hippocampus of adult rodents. European Journal of Pharmacology, 587(1-3), 90–98. https://doi.org/10.1016/j.ejphar.2008.03.048
- Bercik P., Denou E., Collins J., Jackson W., Lu J., Jury J., Deng Y., Blennerhassett P., Macri J., McCoy K. D., Verdu E. F., Collins S. M. (2011). The intestinal microbiota affect central levels of brain-derived neurotropic factor and behavior in mice. Gastroenterology, 141(2), 599–609.e3. https://doi.org/10.1053/j.gastro.2011.04.052
- Berton O., Nestler E. J. (2006). New approaches to antidepressant drug discovery: Beyond monoamines. Nature Reviews Neuroscience, 7(2), 137–151. https://doi.org/10.1038/nrn1846
- Beurel E., Toups M., Nemeroff C. B. (2020). The bidirectional relationship of depression and inflammation: Double trouble. Neuron, 107(2), 234–256. https://doi.org/10.1016/j.neuron.2020.06.002
- Bhatt S., Nagappa A. N., Patil C. R. (2020). Role of oxidative stress in depression. Drug Discovery Today, 25(7), 1270–1276. https://doi.org/10.1016/j.drudis.2020.05.001
- Bjorkholm C., Monteggia L. M. (2016). BDNF-a key transducer of antidepressant effects. Neuropharmacology, 102, 72–79. https://doi.org/10.1016/j.neuropharm.2015.10.034
- Boehme M., van de Wouw M., Bastiaanssen T. F. S., Olavarría-Ramírez L., Lyons K., Fouhy F., Golubeva A. V., Moloney G. M., Minuto C., Sandhu K. V., Scott K. A., Clarke G., Stanton C., Dinan T. G., Schellekens H., Cryan J. F. (2020). Mid-life microbiota crises: Middle age is associated with pervasive neuroimmune alterations that are reversed by targeting the gut microbiome. Molecular Psychiatry, 25(10), 2567–2583. https://doi.org/10.1038/s41380-019-0425-1
- Bonaccorso S., Marino V., Biondi M., Grimaldi F., Ippoliti F., Maes M. (2002). Depression induced by treatment with interferon-alpha in patients affected by hepatitis C virus. Journal of Affective Disorders, 72(3), 237–241. https://doi.org/10.1016/s0165-0327(02)00264-1
- Borrelli L., Aceto S., Agnisola C., De Paolo S., Dipineto L., Stilling R. M., Dinan T. G., Cryan J. F., Menna L. F., Fioretti A. (2016). Probiotic modulation of the microbiota-gut-brain axis and behaviour in zebrafish. Scientific Reports, 6, 30046. https://doi.org/10.1038/srep30046
- Boycott H. E., Wilkinson J. A., Boyle J. P., Pearson H. A., Peers C. (2008). Differential involvement of TNF alpha in hypoxic suppression of astrocyte glutamate transporters. Glia, 56(9), 998–1004. https://doi.org/10.1002/glia.20673
- Bravo J. A., Forsythe P., Chew M. V., Escaravage E., Savignac H. M., Dinan T. G., Bienenstock J., Cryan J. F. (2011). Ingestion of Lactobacillus strain regulates emotional behavior and central GABA receptor expression in a mouse via the vagus nerve. Proceedings of the National Academy of Sciences, 108(38), 16050–16055. https://doi.org/10.1073/pnas.1102999108
- Brigadski T., Leßmann V. (2020). The physiology of regulated BDNF release. Cell and Tissue Research, 382(1), 15–45. https://doi.org/10.1007/s00441-020-03253-2
- Brunt V. E., Gioscia-Ryan R. A., Richey J. J., Zigler M. C., Cuevas L. M., Gonzalez A., Vázquez-Baeza Y., Battson M. L., Smithson A. T., Gilley A. D., Ackermann G., Neilson A. P., Weir T., Davy K. P., Knight R., Seals D. R. (2019). Suppression of the gut microbiome ameliorates age-related arterial dysfunction and oxidative stress in mice. The Journal of Physiology, 597(9), 2361–2378. https://doi.org/10.1113/jp277336
- Bugajski A. J., Gadek-Michalska A., Bugajski J. (2006). The involvement of nitric oxide and prostaglandins in the cholinergic stimulation of hypothalamie-pituitary-adrenal response during crowding stress. Journal of Physiology and Pharmacology, 57(3), 463–477.
- Byrnes K. R., Stoica B., Loane D. J., Riccio A., Davis M. I., Faden A. I. (2009). Metabotropic glutamate receptor 5 activation inhibits microglial associated inflammation and neurotoxicity. Glia, 57(5), 550–560. https://doi.org/10.1002/glia.20783
- Cain D. W., Cidlowski J. A. (2017). Immune regulation by glucocorticoids. Nature Reviews Immunology, 17(4), 233–247. https://doi.org/10.1038/nri.2017.1
- Camkurt M. A., Fındıklı E., İzci F., Kurutaş E. B., Tuman T. C. (2016). Evaluation of malondialdehyde, superoxide dismutase and catalase activity and their diagnostic value in drug naïve, first episode, non-smoker major depression patients and healthy controls. Psychiatry Research, 238, 81–85. https://doi.org/10.1016/j.psychres.2016.01.075
- Cao C., Liu M., Qu S., Huang R., Qi M., Zhu Z., Zheng J., Chen Z., Wang Z., Han Z., Zhu Y., Huang F., Duan J. A. (2020). Chinese medicine formula Kai-Xin-San ameliorates depression-like behaviours in chronic unpredictable mild stressed mice by regulating gut microbiota-inflammation-stress system. Journal of Ethnopharmacology, 261, 113055. https://doi.org/10.1016/j.jep.2020.113055
- Cao X., Li L. P., Wang Q., Wu Q., Hu H. H., Zhang M., Fang Y. Y., Zhang J., Li S. J., Xiong W. C., Yan H. C., Gao Y. B., Liu J. H., Li X. W., Sun L. R., Zeng Y. N., Zhu X. H., Gao T. M. (2013). Astrocyte-derived ATP modulates depressive-like behaviors. Nature Medicine, 19(6), 773–777. https://doi.org/10.1038/nm.3162
- Carlessi A. S., Borba L. A., Zugno A. I., Quevedo J., Réus G. Z. (2021). Gut microbiota-brain axis in depression: The role of neuroinflammation. European Journal of Neuroscience, 53(1), 222–235. https://doi.org/10.1111/ejn.14631
- Casarotto P., Umemori J., Castren E. (2022). BDNF receptor TrkB as the mediator of the antidepressant drug action. Frontiers in Molecular Neuroscience, 15, 1032224. https://doi.org/10.3389/fnmol.2022.1032224
- Casarotto P. C., Girych M., Fred S. M., Kovaleva V., Moliner R., Enkavi G., Biojone C., Cannarozzo C., Sahu M. P., Kaurinkoski K., Brunello C. A., Steinzeig A., Winkel F., Patil S., Vestring S., Serchov T., Diniz C., Laukkanen L., Cardon I., Antila H., Rog T., Piepponen T. P., Bramham C. R., Normann C., Lauri S. E., Saarma M., Vattulainen I., Castren E. (2021). Antidepressant drugs act by directly binding to TrkB neurotrophin receptors. Cell, 184(5), 1299–1313.e19. https://doi.org/10.1016/j.cell.2021.01.034
- Castilho R. F., Ward M. W., Nicholls D. G. (1999). Oxidative stress, mitochondrial function, and acute glutamate excitotoxicity in cultured cerebellar granule cells. Journal of Neurochemistry, 72(4), 1394–1401. https://doi.org/10.1046/j.1471-4159.1999.721394.x
- Castren E., Monteggia L. M. (2021). Brain-derived neurotrophic factor signaling in depression and antidepressant action. Biological Psychiatry, 90(2), 128–136. https://doi.org/10.1016/j.biopsych.2021.05.008
- Catena-Dell’Osso M., Rotella F., Dell’Osso A., Fagiolini A., Marazziti D. (2013). Inflammation, serotonin and major depression. Current Drug Targets, 14(5), 571–577. https://doi.org/10.2174/13894501113149990154
- Caviedes A., Lafourcade C., Soto C., Wyneken U. (2017). BDNF/NF-κB signaling in the neurobiology of depression. Current Pharmaceutical Design, 23(21), 3154–3163. https://doi.org/10.2174/1381612823666170111141915
- Chacón-Fernández P., Säuberli K., Colzani M., Moreau T., Ghevaert C., Barde Y. A. (2016). Brain-derived neurotrophic factor in megakaryocytes. Journal of Biological Chemistry, 291(19), 9872–9881. https://doi.org/10.1074/jbc.M116.720029
- Chen C. J., Ou Y. C., Chang C. Y., Pan H. C., Liao S. L., Chen S. Y., Raung S. L., Lai C. Y. (2012). Glutamate released by Japanese encephalitis virus-infected microglia involves TNF-alpha signaling and contributes to neuronal death. Glia, 60(3), 487–501. https://doi.org/10.1002/glia.22282
- Chen H., Amazit L., Lombès M., Le Menuet D. (2019). Crosstalk between glucocorticoid receptor and early-growth response protein 1 accounts for repression of brain-derived neurotrophic factor transcript 4 expression. Neuroscience, 399, 12–27. https://doi.org/10.1016/j.neuroscience.2018.12.012
- Chen H., Lombès M., Le Menuet D. (2017). Glucocorticoid receptor represses brain-derived neurotrophic factor expression in neuron-like cells. Molecular Brain, 10(1), 12. https://doi.org/10.1186/s13041-017-0295-x
- Chen J., Li J., Qiao H., Hu R., Li C. (2023). Disruption of IDO signaling pathway alleviates chronic unpredictable mild stress-induced depression-like behaviors and tumor progression in mice with breast cancer. Cytokine, 162, 156115. https://doi.org/10.1016/j.cyto.2022.156115
- Chen S. D., Wu C. L., Hwang W. C., Yang D. I. (2017). More insight into BDNF against neurodegeneration: Anti-apoptosis, anti-oxidation, and suppression of autophagy. International Journal of Molecular Sciences, 18(3), 545. https://doi.org/10.3390/ijms18030545
- Chen Z., Zhong C. (2014). Oxidative stress in Alzheimer’s disease. Neuroscience Bulletin, 30(2), 271–281. https://doi.org/10.1007/s12264-013-1423-y
- Cho Y., Hwang H., Rahman M. A., Chung C., Rhim H. (2020). Elevated O-GlcNAcylation induces an antidepressant-like phenotype and decreased inhibitory transmission in medial prefrontal cortex. Scientific Reports, 10(1), 6924. https://doi.org/10.1038/s41598-020-63819-6
- Cipriani A., Furukawa T. A., Salanti G., Chaimani A., Atkinson L. Z., Ogawa Y., Leucht S., Ruhe H. G., Turner E. H., Higgins J. P. T., Egger M., Takeshima N., Hayasaka Y., Imai H., Shinohara K., Tajika A., Ioannidis J. P. A., Geddes J. R. (2018). Comparative efficacy and acceptability of 21 antidepressant drugs for the acute treatment of adults with major depressive disorder: A systematic review and network meta-analysis. The Lancet, 391(10128), 1357–1366. https://doi.org/10.1016/s0140-6736(17)32802-7
- Clark I. A., Vissel B. (2016). Excess cerebral TNF causing glutamate excitotoxicity rationalizes treatment of neurodegenerative diseases and neurogenic pain by anti-TNF agents. Journal of Neuroinflammation, 13(1), 236. https://doi.org/10.1186/s12974-016-0708-2
- Cobley J. N., Fiorello M. L., Bailey D. M. (2018). 13 reasons why the brain is susceptible to oxidative stress. Redox Biology, 15, 490–503. https://doi.org/10.1016/j.redox.2018.01.008
- Comasco E., Schijven D., de Maeyer H., Vrettou M., Nylander I., Sundström-Poromaa I., Olivier J. D. A. (2019). Constitutive serotonin transporter reduction resembles maternal separation with regard to stress-related gene expression. ACS Chemical Neuroscience, 10(7), 3132–3142. https://doi.org/10.1021/acschemneuro.8b00595
- Connor T. J., Starr N., O’Sullivan J. B., Harkin A. (2008). Induction of indolamine 2,3-dioxygenase and kynurenine 3-monooxygenase in rat brain following a systemic inflammatory challenge: A role for IFN-gamma? Neuroscience Letters, 441(1), 29–34. https://doi.org/10.1016/j.neulet.2008.06.007
- Correia A. S., Vale N. (2022). Tryptophan metabolism in depression: A narrative review with a focus on serotonin and kynurenine pathways. International Journal of Molecular Sciences, 23(15), 8493. https://doi.org/10.3390/ijms23158493
- Cui W., Mizukami H., Yanagisawa M., Aida T., Nomura M., Isomura Y., Takayanagi R., Ozawa K., Tanaka K., Aizawa H. (2014). Glial dysfunction in the mouse habenula causes depressive-like behaviors and sleep disturbance. The Journal of Neuroscience, 34(49), 16273–16285. https://doi.org/10.1523/JNEUROSCI.1465-14.2014
- Culmsee C., Michels S., Scheu S., Arolt V., Dannlowski U., Alferink J. (2018). Mitochondria, microglia, and the immune system-how are they linked in affective disorders? Frontiers in Psychiatry, 9, 739. https://doi.org/10.3389/fpsyt.2018.00739
- Cuong T. T., Yang C. S., Yuk J. M., Lee H. M., Ko S. R., Cho B. G., Jo E. K. (2009). Glucocorticoid receptor agonist compound K regulates dectin-1-dependent inflammatory signaling through inhibition of reactive oxygen species. Life Sciences, 85(17-18), 625–633. https://doi.org/10.1016/j.lfs.2009.08.014
- Czéh B., Fuchs E., Wiborg O., Simon M. (2016). Animal models of major depression and their clinical implications. Progress in Neuro-Psychopharmacology and Biological Psychiatry, 64, 293–310. https://doi.org/10.1016/j.pnpbp.2015.04.004
- Dallas M., Boycott H. E., Atkinson L., Miller A., Boyle J. P., Pearson H. A., Peers C. (2007). Hypoxia suppresses glutamate transport in astrocytes. The Journal of Neuroscience, 27(15), 3946–3955. https://doi.org/10.1523/JNEUROSCI.5030-06.2007
- Damian F. S., Ana M. H., Jamileh S. (2021). Global prevalence and burden of depressive and anxiety disorders in 204 countries and territories in 2020 due to the COVID-19 pandemic. The Lancet, 398(10312), 1700–1712. https://doi.org/10.1016/s0140-6736(21)02143-7
- Daws L. C., Munn J. L., Valdez M. F., Frosto-Burke T., Hensler J. G. (2007). Serotonin transporter function, but not expression, is dependent on brain-derived neurotrophic factor (BDNF): In vivo studies in BDNF-deficient mice. Journal of Neurochemistry, 101(3), 641–651. https://doi.org/10.1111/j.1471-4159.2006.04392.x
- de Foubert G., O’Neill M. J., Zetterström T. S. (2007). Acute onset by 5-HT(6)-receptor activation on rat brain brain-derived neurotrophic factor and activity-regulated cytoskeletal-associated protein mRNA expression. Neuroscience, 147(3), 778–785. https://doi.org/10.1016/j.neuroscience.2007.04.045
- Deltheil T., Guiard B. P., Cerdan J., David D. J., Tanaka K. F., Repérant C., Guilloux J. P., Coudoré F., Hen R., Gardier A. M. (2008). Behavioral and serotonergic consequences of decreasing or increasing hippocampus brain-derived neurotrophic factor protein levels in mice. Neuropharmacology, 55(6), 1006–1014. https://doi.org/10.1016/j.neuropharm.2008.08.001
- Deng X. Y., Xue J. S., Li H. Y., Ma Z. Q., Fu Q., Qu R., Ma S. P. (2015). Geraniol produces antidepressant-like effects in a chronic unpredictable mild stress mice model. Physiology & Behavior, 152(Pt A), 264–271. https://doi.org/10.1016/j.physbeh.2015.10.008
- Deng Y., Zhou M., Wang J., Yao J., Yu J., Liu W., Wu L., Wang J., Gao R. (2021). Involvement of the microbiota-gut-brain axis in chronic restraint stress: Disturbances of the kynurenine metabolic pathway in both the gut and brain. Gut Microbes, 13(1), 1–16. https://doi.org/10.1080/19490976.2020.1869501
- de Oliveira L. G., Angelo Y. S., Iglesias A. H., Peron J. P. S. (2021). Unraveling the link between mitochondrial dynamics and neuroinflammation. Frontiers in Immunology, 12, 624919. https://doi.org/10.3389/fimmu.2021.624919
- De Palma G., Blennerhassett P., Lu J., Deng Y., Park A. J., Green W., Denou E., Silva M. A., Santacruz A., Sanz Y., Surette M. G., Verdu E. F., Collins S. M., Bercik P. (2015). Microbiota and host determinants of behavioural phenotype in maternally separated mice. Nature Communications, 6, 7735. https://doi.org/10.1038/ncomms8735
- Desbonnet L., Garrett L., Clarke G., Bienenstock J., Dinan T. G. (2008). The probiotic Bifidobacteria infantis: An assessment of potential antidepressant properties in the rat. Journal of Psychiatric Research, 43(2), 164–174. https://doi.org/10.1016/j.jpsychires.2008.03.009
- Ding H., Chen J., Su M., Lin Z., Zhan H., Yang F., Li W., Xie J., Huang Y., Liu X., Liu B., Zhou X. (2020). BDNF promotes activation of astrocytes and microglia contributing to neuroinflammation and mechanical allodynia in cyclophosphamide-induced cystitis. Journal of Neuroinflammation, 17(1), 19. https://doi.org/10.1186/s12974-020-1704-0
- Ding X. F., Li Y. H., Chen J. X., Sun L. J., Jiao H. Y., Wang X. X., Zhou Y. (2017). Involvement of the glutamate/glutamine cycle and glutamate transporter GLT-1 in antidepressant-like effects of Xiao Yao san on chronically stressed mice. BMC Complementary and Alternative Medicine, 17(1), 326. https://doi.org/10.1186/s12906-017-1830-0
- Ding Y., Bu F., Chen T., Shi G., Yuan X., Feng Z., Duan Z., Wang R., Zhang S., Wang Q., Zhou J., Chen Y. (2021). A next-generation probiotic: Akkermansia muciniphila ameliorates chronic stress-induced depressive-like behavior in mice by regulating gut microbiota and metabolites. Applied Microbiology and Biotechnology, 105(21-22), 8411–8426. https://doi.org/10.1007/s00253-021-11622-2
- Dionisie V., Filip G. A., Manea M. C., Manea M., Riga S. (2021). The anti-inflammatory role of SSRI and SNRI in the treatment of depression: A review of human and rodent research studies. Inflammopharmacology, 29(1), 75–90. https://doi.org/10.1007/s10787-020-00777-5
- Du J., McEwen B., Manji H. K. (2009). Glucocorticoid receptors modulate mitochondrial function: A novel mechanism for neuroprotection. Communicative & Integrative Biology, 2(4), 350–352. https://doi.org/10.4161/cib.2.4.8554
- Duda W., Kubera M., Kreiner G., Curzytek K., Detka J., Glombik K., Slusarczyk J., Basta-Kaim A., Budziszewska B., Lason W., Regulska M., Leskiewicz M., Roman A., Zelek-Molik A., Nalepa I. (2017). Suppression of pro-inflammatory cytokine expression and lack of anti-depressant-like effect of fluoxetine in lipopolysaccharide-treated old female mice. International Immunopharmacology, 48, 35–42. https://doi.org/10.1016/j.intimp.2017.04.021
- El Aidy S., Ramsteijn A. S., Dini-Andreote F., van Eijk R., Houwing D. J., Salles J. F., Olivier J. D. A. (2017). Serotonin transporter genotype modulates the gut microbiota composition in young rats, an effect augmented by early life stress. Frontiers in Cellular Neuroscience, 11, 222. https://doi.org/10.3389/fncel.2017.00222
- Enache D., Pariante C. M., Mondelli V. (2019). Markers of central inflammation in major depressive disorder: A systematic review and meta-analysis of studies examining cerebrospinal fluid, positron emission tomography and post-mortem brain tissue. Brain, Behavior, and Immunity, 81, 24–40. https://doi.org/10.1016/j.bbi.2019.06.015
- Eskelund A., Li Y., Budac D. P., Müller H. K., Gulinello M., Sanchez C., Wegener G. (2017). Drugs with antidepressant properties affect tryptophan metabolites differently in rodent models with depression-like behavior. Journal of Neurochemistry, 142(1), 118–131. https://doi.org/10.1111/jnc.14043
- Espinoza M. B., Aedo J. E., Zuloaga R., Valenzuela C., Molina A., Valdés J. A. (2017). Cortisol induces reactive oxygen species through a membrane glucocorticoid receptor in rainbow trout myotubes. Journal of Cellular Biochemistry, 118(4), 718–725. https://doi.org/10.1002/jcb.25676
- Evanson N. K., Herman J. P. ( 2015a). Metabotropic glutamate receptor-mediated signaling dampens the HPA axis response to restraint stress. Physiology & Behavior, 150, 2–7. https://doi.org/10.1016/j.physbeh.2015.02.027
- Evanson N. K., Herman J. P. ( 2015b). Role of paraventricular nucleus glutamate signaling in regulation of HPA axis stress responses. Interdiscip Inf Sci, 21(3), 253–260. https://doi.org/10.4036/iis.2015.B.10
- Fan J., Guo F., Mo R., Chen L. Y., Mo J., Lu C. L., Ren J., Zhong Q., Kuang X., Wen Y., Gu T. T., Liu J., Li S., Fang Y., Zhao C., Gao T. M., Cao X. (2023). O-GlcNAc transferase in astrocytes modulates depression-related stress susceptibility through glutamatergic synaptic transmission. Journal of Clinical Investigation, 133(7). https://doi.org/10.1172/jci160016
- Fan J. F., Tang Z. H., Wang S. Y., Lei S., Zhang B., Tian S. W. (2021). Ketamine enhances novel object recognition memory reconsolidation via the BDNF/TrkB pathway in mice. Physiology & Behavior, 242, 113626. https://doi.org/10.1016/j.physbeh.2021.113626
- Freimer D., Yang T. T., Ho T. C., Tymofiyeva O., Leung C. (2022). The gut microbiota, HPA axis, and brain in adolescent-onset depression: Probiotics as a novel treatment. Brain, Behavior, & Immunity - Health, 26, 100541. https://doi.org/10.1016/j.bbih.2022.100541
- Fu X., Zunich S. M., O’Connor J. C., Kavelaars A., Dantzer R., Kelley K. W. (2010). Central administration of lipopolysaccharide induces depressive-like behavior in vivo and activates brain indoleamine 2,3 dioxygenase in murine organotypic hippocampal slice cultures. Journal of Neuroinflammation, 7(1), 43. https://doi.org/10.1186/1742-2094-7-43
- Fujimura H., Altar C. A., Chen R., Nakamura T., Nakahashi T., Kambayashi J., Sun B., Tandon N. N. (2002). Brain-derived neurotrophic factor is stored in human platelets and released by agonist stimulation. Thrombosis and Haemostasis, 87(4), 728–734. https://doi.org/10.1055/s-0037-1613072
- Fukumoto K., Fogaça M. V., Liu R. J., Duman C., Kato T., Li X. Y., Duman R. S. (2019). Activity-dependent brain-derived neurotrophic factor signaling is required for the antidepressant actions of (2R,6R)-hydroxynorketamine. Proceedings of the National Academy of Sciences, 116(1), 297–302. https://doi.org/10.1073/pnas.1814709116
- Gadek-Michalska A., Bugajski J. (2005). Nitric oxide mediates the interleukin-1beta- and nicotine-induced hypothalamic-pituitary-adrenocortical response during social stress. Journal of Physiology and Pharmacology, 56(3), 491–503.
- Gaidin S. G., Turovskaya M. V., Gavrish M. S., Babaev A. A., Mal’tseva V. N., Blinova E. V., Turovsky E. A. (2020). The selective BDNF overexpression in neurons protects neuroglial networks against OGD and glutamate-induced excitotoxicity. International Journal of Neuroscience, 130(4), 363–383. https://doi.org/10.1080/00207454.2019.1691205
- Gao K., Farzi A., Ke X., Yu Y., Chen C., Chen S., Yu T., Wang H., Li Y. (2022). Oral administration of Lactococcus lactis WHH2078 alleviates depressive and anxiety symptoms in mice with induced chronic stress. Food & Function, 13(2), 957–969. https://doi.org/10.1039/d1fo03723d
- García-García A. L., Venzala E., Elizalde N., Ramírez M. J., Urbiola A., Del Rio J., Lanfumey L., Tordera R. M. (2013). Regulation of serotonin (5-HT) function by a VGLUT1 dependent glutamate pathway. Neuropharmacology, 70, 190–199. https://doi.org/10.1016/j.neuropharm.2012.11.005
- Garcia-Garcia M. L., Tovilla-Zarate C. A., Villar-Soto M., Juarez-Rojop I. E., Gonzalez-Castro T. B., Genis-Mendoza A. D., Ramos-Mendez M. A., Lopez-Narvaez M. L., Saucedo-Osti A. S., Ruiz-Quinones J. A., Martinez-Magana J. J. (2022). Fluoxetine modulates the pro-inflammatory process of IL-6, IL-1beta and TNF-alpha levels in individuals with depression: A systematic review and meta-analysis. Psychiatry Research, 307, 114317. https://doi.org/10.1016/j.psychres.2021.114317
- Gasull-Camós J., Tarrés-Gatius M., Artigas F., Castañé A. (2017). Glial GLT-1 blockade in infralimbic cortex as a new strategy to evoke rapid antidepressant-like effects in rats. Translational Psychiatry, 7(2), e1038. https://doi.org/10.1038/tp.2017.7
- Getachew B., Aubee J. I., Schottenfeld R. S., Csoka A. B., Thompson K. M., Tizabi Y. (2018). Ketamine interactions with gut-microbiota in rats: Relevance to its antidepressant and anti-inflammatory properties. BMC Microbiology, 18(1), 222. https://doi.org/10.1186/s12866-018-1373-7
- Gheorghe C. E., Martin J. A., Manriquez F. V., Dinan T. G., Cryan J. F., Clarke G. (2019). Focus on the essentials: Tryptophan metabolism and the microbiome-gut-brain axis. Current Opinion in Pharmacology, 48, 137–145. https://doi.org/10.1016/j.coph.2019.08.004
- Giridharan V. V., Reus G. Z., Selvaraj S., Scaini G., Barichello T., Quevedo J. (2019). Maternal deprivation increases microglial activation and neuroinflammatory markers in the prefrontal cortex and hippocampus of infant rats. Journal of Psychiatric Research, 115, 13–20. https://doi.org/10.1016/j.jpsychires.2019.05.001
- Gritti D., Delvecchio G., Ferro A., Bressi C., Brambilla P. (2021). Neuroinflammation in major depressive disorder: A review of PET imaging studies examining the 18-kDa translocator protein. Journal of Affective Disorders, 292, 642–651. https://doi.org/10.1016/j.jad.2021.06.001
- Guan X., Pang T. (2023). Fast-onset antidepressant targeting the nNOS-SERT interaction in the DRN. Chinese Journal of Natural Medicines, 21(1), 1–2. https://doi.org/10.1016/s1875-5364(23)60380-2.
- Guan Z., Fang J. (2006). Peripheral immune activation by lipopolysaccharide decreases neurotrophins in the cortex and hippocampus in rats. Brain, Behavior, and Immunity, 20(1), 64–71. https://doi.org/10.1016/j.bbi.2005.04.005
- Guo J. D., Rainnie D. G. (2010). Presynaptic 5-HT(1B) receptor-mediated serotonergic inhibition of glutamate transmission in the bed nucleus of the stria terminalis. Neuroscience, 165(4), 1390–1401. https://doi.org/10.1016/j.neuroscience.2009.11.071
- Guo L. T., Wang S. Q., Su J., Xu L. X., Ji Z. Y., Zhang R. Y., Zhao Q. W., Ma Z. Q., Deng X. Y., Ma S. P. (2019). Baicalin ameliorates neuroinflammation-induced depressive-like behavior through inhibition of toll-like receptor 4 expression via the PI3K/AKT/FoxO1 pathway. Journal of Neuroinflammation, 16(1), 95. https://doi.org/10.1186/s12974-019-1474-8
- Guo Y., Xie J. P., Deng K., Li X., Yuan Y., Xuan Q., Xie J., He X. M., Wang Q., Li J. J., Luo H. R. (2019). Prophylactic effects of Bifidobacterium adolescentis on anxiety and depression-like phenotypes after chronic stress: A role of the gut microbiota-inflammation axis. Frontiers in Behavioral Neuroscience, 13, 126. https://doi.org/10.3389/fnbeh.2019.00126
- Haroon E., Miller A. H. (2017). Inflammation effects on brain glutamate in depression: Mechanistic considerations and treatment implications. Current Topics in Behavioral Neurosciences, 31, 173–198. https://doi.org/10.1007/7854_2016_40
- Hashimoto K., Sawa A., Iyo M. (2007). Increased levels of glutamate in brains from patients with mood disorders. Biological Psychiatry, 62(11), 1310–1316. https://doi.org/10.1016/j.biopsych.2007.03.017
- He H., Xie X., Zhang J., Mo L., Kang X., Zhang Y., Wang L., Hu N., Xie L., Peng C., You Z. (2023). Patchouli alcohol ameliorates depression-like behaviors through inhibiting NLRP3-mediated neuroinflammation in male stress-exposed mice. Journal of Affective Disorders, 326, 120–131. https://doi.org/10.1016/j.jad.2023.01.065
- Herken H., Gurel A., Selek S., Armutcu F., Ozen M. E., Bulut M., Kap O., Yumru M., Savas H. A., Akyol O. (2007). Adenosine deaminase, nitric oxide, superoxide dismutase, and xanthine oxidase in patients with major depression: Impact of antidepressant treatment. Archives of Medical Research, 38(2), 247–252. https://doi.org/10.1016/j.arcmed.2006.10.005
- Hertz L., Zielke H. R. (2004). Astrocytic control of glutamatergic activity: Astrocytes as stars of the show. Trends in Neurosciences, 27(12), 735–743. https://doi.org/10.1016/j.tins.2004.10.008
- Hoban A. E., Moloney R. D., Golubeva A. V., McVey Neufeld K. A., O’Sullivan O., Patterson E., Stanton C., Dinan T. G., Clarke G., Cryan J. F. (2016). Behavioural and neurochemical consequences of chronic gut microbiota depletion during adulthood in the rat. Neuroscience, 339, 463–477. https://doi.org/10.1016/j.neuroscience.2016.10.003
- Hollis F., Pope B. S., Gorman-Sandler E., Wood S. K. (2022). Neuroinflammation and mitochondrial dysfunction link social stress to depression. Curr Top Behav Neurosci, 54, 59–93. https://doi.org/10.1007/7854_2021_300
- Hooper L. V., Littman D. R., Macpherson A. J. (2012). Interactions between the microbiota and the immune system. Science, 336(6086), 1268–1273. https://doi.org/10.1126/science.1223490
- Hu S., Sheng W. S., Ehrlich L. C., Peterson P. K., Chao C. C. (2000). Cytokine effects on glutamate uptake by human astrocytes. Neuroimmunomodulation, 7(3), 153–159. https://doi.org/10.1159/000026433
- Huang N., Hua D., Zhan G., Li S., Zhu B., Jiang R., Yang L., Bi J., Xu H., Hashimoto K., Luo A., Yang C. (2019). Role of Actinobacteria and Coriobacteriia in the antidepressant effects of ketamine in an inflammation model of depression. Pharmacology Biochemistry and Behavior, 176, 93–100. https://doi.org/10.1016/j.pbb.2018.12.001
- Huang Y., Wang Y., Wang H., Liu Z., Yu X., Yan J., Yu Y., Kou C., Xu X., Lu J., Wang Z., He S., Xu Y., He Y., Li T., Guo W., Tian H., Xu G., Xu X., Ma Y., Wang L., Wang L., Yan Y., Wang B., Xiao S., Zhou L., Li L., Tan L., Zhang T., Ma C., Li Q., Ding H., Geng H., Jia F., Shi J., Wang S., Zhang N., Du X., Du X., Wu Y. (2019). Prevalence of mental disorders in China: A cross-sectional epidemiological study. The Lancet Psychiatry, 6(3), 211–224. https://doi.org/10.1016/S2215-0366(18)30511-X
- Iatsenko I., Boquete J. P., Lemaitre B. (2018). Microbiota-derived lactate activates production of reactive oxygen species by the Intestinal NADPH oxidase NOX and shortens Drosophila lifespan. Immunity, 49(5), 929–942.e5. https://doi.org/10.1016/j.immuni.2018.09.017
- Inserra A., Mastronardi C. A., Rogers G., Licinio J., Wong M. L. (2019). Neuroimmunomodulation in major depressive disorder: Focus on caspase 1, inducible nitric oxide synthase, and interferon-gamma. Molecular Neurobiology, 56(6), 4288–4305. https://doi.org/10.1007/s12035-018-1359-3
- Inserra A., Rogers G. B., Licinio J., Wong M. L. (2018). The Microbiota-inflammasome hypothesis of major depression. Bioessays, 40(9), e1800027. https://doi.org/10.1002/bies.201800027
- Jean Y. Y., Lercher L. D., Dreyfus C. F. (2008). Glutamate elicits release of BDNF from basal forebrain astrocytes in a process dependent on metabotropic receptors and the PLC pathway. Neuron Glia Biology, 4(1), 35–42. https://doi.org/10.1017/S1740925X09000052
- John C. S., Smith K. L., Van’t Veer A., Gompf H. S., Carlezon W. A.Jr., Cohen B. M., Öngür D., Bechtholt-Gompf A. J. (2012). Blockade of astrocytic glutamate uptake in the prefrontal cortex induces anhedonia. Neuropsychopharmacology, 37(11), 2467–2475. https://doi.org/10.1038/npp.2012.105
- John C. S., Sypek E. I., Carlezon W. A., Cohen B. M., Öngür D., Bechtholt A. J. (2015). Blockade of the GLT-1 transporter in the central nucleus of the amygdala induces both anxiety and depressive-like symptoms. Neuropsychopharmacology, 40(7), 1700–1708. https://doi.org/10.1038/npp.2015.16
- Johnson F. K., Kaffman A. (2018). Early life stress perturbs the function of microglia in the developing rodent brain: New insights and future challenges. Brain, Behavior, and Immunity, 69, 18–27. https://doi.org/10.1016/j.bbi.2017.06.008
- Jones R. M., Neish A. S. (2017). Redox signaling mediated by the gut microbiota. Free Radical Biology and Medicine, 105, 41–47. https://doi.org/10.1016/j.freeradbiomed.2016.10.495
- Juszczyk G., Mikulska J., Kasperek K., Pietrzak D., Mrozek W., Herbet M. (2021). Chronic stress and oxidative stress as common factors of the pathogenesis of depression and Alzheimer’s disease: The role of antioxidants in prevention and treatment. Antioxidants, 10(9), 1439. https://doi.org/10.3390/antiox10091439
- Kapczinski F., Frey B. N., Andreazza A. C., Kauer-Sant’Anna M., Cunha A. B., Post R. M. (2008). Increased oxidative stress as a mechanism for decreased BDNF levels in acute manic episodes. Revista Brasileira de Psiquiatria, 30(3), 243–245. https://doi.org/10.1590/s1516-44462008000300011
- Karege F., Bondolfi G., Gervasoni N., Schwald M., Aubry J. M., Bertschy G. (2005). Low brain-derived neurotrophic factor (BDNF) levels in serum of depressed patients probably results from lowered platelet BDNF release unrelated to platelet reactivity. Biological Psychiatry, 57(9), 1068–1072. https://doi.org/10.1016/j.biopsych.2005.01.008
- Kawashima H., Numakawa T., Kumamaru E., Adachi N., Mizuno H., Ninomiya M., Kunugi H., Hashido K. (2010). Glucocorticoid attenuates brain-derived neurotrophic factor-dependent upregulation of glutamate receptors via the suppression of microRNA-132 expression. Neuroscience, 165(4), 1301–1311. https://doi.org/10.1016/j.neuroscience.2009.11.057
- Keefe B. (2007). Interferon-induced depression in hepatitis C: An update. Current Psychiatry Reports, 9(3), 255–261. https://doi.org/10.1007/s11920-007-0028-4
- Kelly J. R., Borre Y., O’Brien C., Patterson E., El Aidy S., Deane J., Kennedy P. J., Beers S., Scott K., Moloney G., Hoban A. E., Scott L., Fitzgerald P., Ross P., Stanton C., Clarke G., Cryan J. F., Dinan T. G. (2016). Transferring the blues: Depression-associated gut microbiota induces neurobehavioural changes in the rat. Journal of Psychiatric Research, 82, 109–118. https://doi.org/10.1016/j.jpsychires.2016.07.019
- Khanzode S. D., Dakhale G. N., Khanzode S. S., Saoji A., Palasodkar R. (2003). Oxidative damage and major depression: The potential antioxidant action of selective serotonin re-uptake inhibitors. Redox Report, 8(6), 365–370. https://doi.org/10.1179/135100003225003393
- Kingwell K. (2022). A faster route to antidepressant activity. Nature Reviews Drug Discovery, 21(12), 877. https://doi.org/10.1038/d41573-022-00188-x
- Kinlein S. A., Wallace N. K., Savenkova M. I., Karatsoreos I. N. (2022). Chronic hypothalamic-pituitary-adrenal axis disruption alters glutamate homeostasis and neural responses to stress in male C57Bl6/N mice. Neurobiology of Stress, 19, 100466. https://doi.org/10.1016/j.ynstr.2022.100466
- Kokkinopoulou I., Moutsatsou P. (2021). Mitochondrial glucocorticoid receptors and their actions. International Journal of Molecular Sciences, 22(11), 6054. https://doi.org/10.3390/ijms22116054
- Kong L. Z., Lai J. B., Hu S. H. (2022). China Initiates depression screening in children and adolescents. The Lancet Psychiatry, 9(2), 107–108. https://doi.org/10.1016/S2215-0366(21)00479-X
- Kozisek M. E., Middlemas D., Bylund D. B. (2008). Brain-derived neurotrophic factor and its receptor tropomyosin-related kinase B in the mechanism of action of antidepressant therapies. Pharmacology & Therapeutics, 117(1), 30–51. https://doi.org/10.1016/j.pharmthera.2007.07.001
- Krautkramer K. A., Fan J., Bäckhed F. (2021). Gut microbial metabolites as multi-kingdom intermediates. Nature Reviews Microbiology, 19(2), 77–94. https://doi.org/10.1038/s41579-020-0438-4
- Krishnan V., Nestler E. J. (2008). The molecular neurobiology of depression. Nature, 455(7215), 894–902. https://doi.org/10.1038/nature07455
- Kume T., Nishikawa H., Tomioka H., Katsuki H., Akaike A., Kaneko S., Maeda T., Kihara T., Shimohama S. (2000). p75-mediated neuroprotection by NGF against glutamate cytotoxicity in cortical cultures. Brain Research, 852(2), 279–289. https://doi.org/10.1016/s0006-8993(99)02226-x
- Kundu P., Blacher E., Elinav E., Pettersson S. (2017). Our gut microbiome: The evolving inner self. Cell, 171(7), 1481–1493. https://doi.org/10.1016/j.cell.2017.11.024
- Lafon-Cazal M., Pietri S., Culcasi M., Bockaert J. (1993). NMDA-dependent superoxide production and neurotoxicity. Nature, 364(6437), 535–537. https://doi.org/10.1038/364535a0
- Lambert W. M., Xu C. F., Neubert T. A., Chao M. V., Garabedian M. J., Jeanneteau F. D. (2013). Brain-derived neurotrophic factor signaling rewrites the glucocorticoid transcriptome via glucocorticoid receptor phosphorylation. Molecular and Cellular Biology, 33(18), 3700–3714. https://doi.org/10.1128/mcb.00150-13
- Lau A., Tymianski M. (2010). Glutamate receptors, neurotoxicity and neurodegeneration. Pflügers Archiv - European Journal of Physiology, 460(2), 525–542. https://doi.org/10.1007/s00424-010-0809-1
- Lawson M. A., Parrott J. M., McCusker R. H., Dantzer R., Kelley K. W., O’Connor J. C. (2013). Intracerebroventricular administration of lipopolysaccharide induces indoleamine-2,3-dioxygenase-dependent depression-like behaviors. Journal of Neuroinflammation, 10(1), 87. https://doi.org/10.1186/1742-2094-10-87
- Lee H. Y., Kim Y. K. (2008). Plasma brain-derived neurotrophic factor as a peripheral marker for the action mechanism of antidepressants. Neuropsychobiology, 57(4), 194–199. https://doi.org/10.1159/000149817
- Lepack A. E., Fuchikami M., Dwyer J. M., Banasr M., Duman R. S. (2014). BDNF release is required for the behavioral actions of ketamine. International Journal of Neuropsychopharmacology, 18(1), pyu033–pyu033. https://doi.org/10.1093/ijnp/pyu033.
- Li H. Y., Zhu M. Z., Yuan X. R., Guo Z. X., Pan Y. D., Li Y. Q., Zhu X. H. (2023). A thalamic-primary auditory cortex circuit mediates resilience to stress. Cell, 186(7), 1352–1368.e18. https://doi.org/10.1016/j.cell.2023.02.036
- Li N., Wang Q., Wang Y., Sun A., Lin Y., Jin Y., Li X. (2019). Fecal microbiota transplantation from chronic unpredictable mild stress mice donors affects anxiety-like and depression-like behavior in recipient mice via the gut microbiota-inflammation-brain axis. Stress, 22(5), 592–602. https://doi.org/10.1080/10253890.2019.1617267
- Li W., Ali T., Zheng C., He K., Liu Z., Shah F. A., Li N., Yu Z. J., Li S. (2022). Anti-depressive-like behaviors of APN KO mice involve Trkb/BDNF signaling related neuroinflammatory changes. Molecular Psychiatry, 27(2), 1047–1058. https://doi.org/10.1038/s41380-021-01327-3
- Li Z. R., Han Y. S., Liu Z., Zhao H. Q., Liu J., Yang H., Wang Y. H. (2021). GR/NF-κB signaling pathway regulates hippocampal inflammatory responses in diabetic rats with chronic unpredictable mild stress. European Journal of Pharmacology, 895, 173861. https://doi.org/10.1016/j.ejphar.2021.173861
- Lin C. C., Huang T. L. (2020). Brain-derived neurotrophic factor and mental disorders. Biomedical Journal, 43(2), 134–142. https://doi.org/10.1016/j.bj.2020.01.001
- Lin S., Huang L., Luo Z. C., Li X., Jin S. Y., Du Z. J., Wu D. Y., Xiong W. C., Huang L., Luo Z. Y., Song Y. L., Wang Q., Liu X. W., Ma R. J., Wang M. L., Ren C. R., Yang J. M., Gao T. M. (2022). The ATP level in the medial prefrontal cortex regulates depressive-like behavior via the medial prefrontal cortex-lateral habenula pathway. Biological Psychiatry, 92(3), 179–192. https://doi.org/10.1016/j.biopsych.2022.02.014
- Lindqvist D., Dhabhar F. S., James S. J., Hough C. M., Jain F. A., Bersani F. S., Reus V. I., Verhoeven J. E., Epel E. S., Mahan L., Rosser R., Wolkowitz O. M., Mellon S. H. (2017). Oxidative stress, inflammation and treatment response in major depression. Psychoneuroendocrinology, 76, 197–205. https://doi.org/10.1016/j.psyneuen.2016.11.031
- Liu S., Xu S., Wang Z., Guo Y., Pan W., Shen Z. (2018). Anti-depressant-like effect of sinomenine on chronic unpredictable mild stress-induced depression in a mouse model. Medical Science Monitor, 24, 7646–7653. https://doi.org/10.12659/MSM.908422
- Liu T., Zhong S., Liao X., Chen J., He T., Lai S., Jia Y. (2015). A meta-analysis of oxidative stress markers in depression. PLoS One, 10(10), e0138904. https://doi.org/10.1371/journal.pone.0138904.
- Liu W. X., Wang J., Xie Z. M., Xu N., Zhang G. F., Jia M., Zhou Z. Q., Hashimoto K., Yang J. J. (2016). Regulation of glutamate transporter 1 via BDNF-TrkB signaling plays a role in the anti-apoptotic and antidepressant effects of ketamine in chronic unpredictable stress model of depression. Psychopharmacology, 233(3), 405–415. https://doi.org/10.1007/s00213-015-4128-2
- Liu Y., Ding X. F., Wang X. X., Zou X. J., Li X. J., Liu Y. Y., Li J., Qian X. Y., Chen J. X. (2019). Xiaoyaosan exerts antidepressant-like effects by regulating the functions of astrocytes and EAATs in the prefrontal cortex of mice. BMC Complementary and Alternative Medicine, 19(1), 215. https://doi.org/10.1186/s12906-019-2613-6
- Lu X., Qi C., Zheng J., Sun M., Jin L., Sun J. (2022). The antidepressant effect of deoiled sunflower seeds on chronic unpredictable mild stress in mice through regulation of microbiota-gut-brain axis. Frontiers in Nutrition, 9, 908297. https://doi.org/10.3389/fnut.2022.908297
- Lukic I., Getselter D., Ziv O., Oron O., Reuveni E., Koren O., Elliott E. (2019). Antidepressants affect gut microbiota and Ruminococcus flavefaciens is able to abolish their effects on depressive-like behavior. Translational Psychiatry, 9(1), 133. https://doi.org/10.1038/s41398-019-0466-x
- Lyu S., Guo Y., Zhang L., Tang G., Li R., Yang J., Gao S., Li W., Liu J. (2021). Downregulation of astroglial glutamate transporter GLT-1 in the lateral habenula is associated with depressive-like behaviors in a rat model of Parkinson’s disease. Neuropharmacology, 196, 108691. https://doi.org/10.1016/j.neuropharm.2021.108691
- Ma H., Xu J., Li R., McIntyre R. S., Teopiz K. M., Cao B., Yang F. (2022). The impact of cognitive behavioral therapy on peripheral interleukin-6 levels in depression: A systematic review and meta-analysis. Frontiers in Psychiatry, 13, 844176. https://doi.org/10.3389/fpsyt.2022.844176
- Madeira C., Vargas-Lopes C., Brandao C. O., Reis T., Laks J., Panizzutti R., Ferreira S. T. (2018). Elevated glutamate and glutamine levels in the cerebrospinal fluid of patients with probable Alzheimer’s disease and depression. Frontiers in Psychiatry, 9, 561. https://doi.org/10.3389/fpsyt.2018.00561
- Malhi G. S., Mann J. J. (2018). Depression. The Lancet, 392(10161), 2299–2312. https://doi.org/10.1016/S0140-6736(18)31948-2
- Malynn S., Campos-Torres A., Moynagh P., Haase J. (2013). The pro-inflammatory cytokine TNF-α regulates the activity and expression of the serotonin transporter (SERT) in astrocytes. Neurochemical Research, 38(4), 694–704. https://doi.org/10.1007/s11064-012-0967-y
- Mao Q. Q., Ip S. P., Ko K. M., Tsai S. H., Che C. T. (2009). Peony glycosides produce antidepressant-like action in mice exposed to chronic unpredictable mild stress: Effects on hypothalamic-pituitary-adrenal function and brain-derived neurotrophic factor. Progress in Neuro-Psychopharmacology and Biological Psychiatry, 33(7), 1211–1216. https://doi.org/10.1016/j.pnpbp.2009.07.002
- Mao Q. Q., Xian Y. F., Ip S. P., Tsai S. H., Che C. T. (2010). Long-term treatment with peony glycosides reverses chronic unpredictable mild stress-induced depressive-like behavior via increasing expression of neurotrophins in rat brain. Behavioural Brain Research, 210(2), 171–177. https://doi.org/10.1016/j.bbr.2010.02.026
- McGuinness A. J., Davis J. A., Dawson S. L., Loughman A., Collier F., O’Hely M., Simpson C. A., Green J., Marx W., Hair C., Guest G., Mohebbi M., Berk M., Stupart D., Watters D., Jacka F. N. (2022). A systematic review of gut microbiota composition in observational studies of major depressive disorder, bipolar disorder and schizophrenia. Molecular Psychiatry, 27(4), 1920–1935. https://doi.org/10.1038/s41380-022-01456-3
- Melo C. V., Okumoto S., Gomes J. R., Baptista M. S., Bahr B. A., Frommer W. B., Duarte C. B. (2013). Spatiotemporal resolution of BDNF neuroprotection against glutamate excitotoxicity in cultured hippocampal neurons. Neuroscience, 237, 66–86. https://doi.org/10.1016/j.neuroscience.2013.01.054
- Menke A. (2019). Is the HPA axis as target for depression outdated, or is there a new hope? Frontiers in Psychiatry, 10, 101. https://doi.org/10.3389/fpsyt.2019.00101
- Mi Y., Qi G., Vitali F., Shang Y., Raikes A. C., Wang T., Jin Y., Brinton R. D., Gu H., Yin F. (2023). Loss of fatty acid degradation by astrocytic mitochondria triggers neuroinflammation and neurodegeneration. Nature Metabolism, 5(3), 445–465. https://doi.org/10.1038/s42255-023-00756-4
- Miller A. H., Raison C. L. (2016). The role of inflammation in depression: From evolutionary imperative to modern treatment target. Nature Reviews Immunology, 16(1), 22–34. https://doi.org/10.1038/nri.2015.5
- Moncrieff J., Cooper R. E., Stockmann T., Amendola S., Hengartner M. P., Horowitz M. A. (2022). The serotonin theory of depression: a systematic umbrella review of the evidence. Molecular Psychiatry. https://doi.org/10.1038/s41380-022-01661-0
- Montanari M., Martella G., Bonsi P., Meringolo M. (2022). Autism spectrum disorder: focus on glutamatergic neurotransmission. International Journal of Molecular Sciences, 23(7), 3861. https://doi.org/10.3390/ijms23073861
- Mossad O., Batut B., Yilmaz B., Dokalis N., Mezö C., Nent E., Nabavi L. S., Mayer M., Maron F. J. M., Buescher J. M., de Agüero M. G., Szalay A., Lämmermann T., Macpherson A. J., Ganal-Vonarburg S. C., Backofen R., Erny D., Prinz M., Blank T. (2022). Gut microbiota drives age-related oxidative stress and mitochondrial damage in microglia via the metabolite N(6)-carboxymethyllysine. Nature Neuroscience, 25(3), 295–305. https://doi.org/10.1038/s41593-022-01027-3
- Mössner R., Daniel S., Albert D., Heils A., Okladnova O., Schmitt A., Lesch K. P. (2000). Serotonin transporter function is modulated by brain-derived neurotrophic factor (BDNF) but not nerve growth factor (NGF). Neurochemistry International, 36(3), 197–202. https://doi.org/10.1016/s0197-0186(99)00122-9
- Moussaoui N., Jacobs J. P., Larauche M., Biraud M., Million M., Mayer E., Taché Y. (2017). Chronic early-life stress in rat pups alters basal corticosterone, intestinal permeability, and fecal microbiota at weaning: Influence of sex. Journal of Neurogastroenterology and Motility, 23(1), 135–143. https://doi.org/10.5056/jnm16105
- Murrough J. W., Abdallah C. G., Mathew S. J. (2017). Targeting glutamate signalling in depression: Progress and prospects. Nature Reviews Drug Discovery, 16(7), 472–486. https://doi.org/10.1038/nrd.2017.16
- Murugan M., Ling E. A., Kaur C. (2013). Glutamate receptors in microglia. CNS & Neurological Disorders - Drug Targets, 12(6), 773–784. https://doi.org/10.2174/18715273113126660174
- Murugan M., Sivakumar V., Lu J., Ling E. A., Kaur C. (2011). Expression of N-methyl D-aspartate receptor subunits in amoeboid microglia mediates production of nitric oxide via NF-kappaB signaling pathway and oligodendrocyte cell death in hypoxic postnatal rats. Glia, 59(4), 521–539. https://doi.org/10.1002/glia.21121
- Naegelin Y., Dingsdale H., Sauberli K., Schadelin S., Kappos L., Barde Y. A. (2018). Measuring and validating the levels of brain-derived neurotrophic factor in human serum. eNeuro, 5(2), eneuro.0419-0417.2018. https://doi.org/10.1523/ENEURO.0419-17.2018
- Nayernia Z., Jaquet V., Krause K. H. (2014). New insights on NOX enzymes in the central nervous system. Antioxidants & Redox Signaling, 20(17), 2815–2837. https://doi.org/10.1089/ars.2013.5703
- Nishijo T., Suzuki E., Momiyama T. (2022). Serotonin 5-HT(1A) and 5-HT(1B) receptor-mediated inhibition of glutamatergic transmission onto rat basal forebrain cholinergic neurones. The Journal of Physiology, 600(13), 3149–3167. https://doi.org/10.1113/jp282509
- Numakawa T., Kumamaru E., Adachi N., Yagasaki Y., Izumi A., Kunugi H. (2009). Glucocorticoid receptor interaction with TrkB promotes BDNF-triggered PLC-gamma signaling for glutamate release via a glutamate transporter. Proceedings of the National Academy of Sciences, 106(2), 647–652. https://doi.org/10.1073/pnas.0800888106
- Numakawa T., Matsumoto T., Adachi N., Yokomaku D., Kojima M., Takei N., Hatanaka H. (2001). Brain-derived neurotrophic factor triggers a rapid glutamate release through increase of intracellular Ca(2+) and Na(+) in cultured cerebellar neurons. Journal of Neuroscience Research, 66(1), 96–108. https://doi.org/10.1002/jnr.1201
- Otte C., Gold S. M., Penninx B. W., Pariante C. M., Etkin A., Fava M., Mohr D. C., Schatzberg A. F. (2016). Major depressive disorder. Nature Reviews Disease Primers, 2(1), 16065. https://doi.org/10.1038/nrdp.2016.65
- Pace T. W., Hu F., Miller A. H. (2007). Cytokine-effects on glucocorticoid receptor function: Relevance to glucocorticoid resistance and the pathophysiology and treatment of major depression. Brain, Behavior, and Immunity, 21(1), 9–19. https://doi.org/10.1016/j.bbi.2006.08.009
- Pajarillo E., Rizor A., Lee J., Aschner M., Lee E. (2019). The role of astrocytic glutamate transporters GLT-1 and GLAST in neurological disorders: Potential targets for neurotherapeutics. Neuropharmacology, 161, 107559. https://doi.org/10.1016/j.neuropharm.2019.03.002
- Pan Y., Chen X. Y., Zhang Q. Y., Kong L. D. (2014). Microglial NLRP3 inflammasome activation mediates IL-1β-related inflammation in prefrontal cortex of depressive rats. Brain, Behavior, and Immunity, 41, 90–100. https://doi.org/10.1016/j.bbi.2014.04.007
- Park S. S., Park H. S., Kim C. J., Baek S. S., Kim T. W. (2019). Exercise attenuates maternal separation-induced mood disorder-like behaviors by enhancing mitochondrial functions and neuroplasticity in the dorsal raphe. Behavioural Brain Research, 372, 112049. https://doi.org/10.1016/j.bbr.2019.112049
- Parul M. A., Singh S., Singh S., Tiwari V., Chaturvedi S., Wahajuddin M., Palit G., Shukla S. (2021). Chronic unpredictable stress negatively regulates hippocampal neurogenesis and promote anxious depression-like behavior via upregulating apoptosis and inflammatory signals in adult rats. Brain Research Bulletin, 172, 164–179. https://doi.org/10.1016/j.brainresbull.2021.04.017
- Pascual M., Climent E., Guerri C. (2001). BDNF induces glutamate release in cerebrocortical nerve terminals and in cortical astrocytes. Neuroreport, 12(12), 2673–2677. https://doi.org/10.1097/00001756-200108280-00017
- Pascual-Brazo J., Castro E., Díaz A., Valdizán E. M., Pilar-Cuéllar F., Vidal R., Treceño B., Pazos A. (2012). Modulation of neuroplasticity pathways and antidepressant-like behavioural responses following the short-term (3 and 7 days) administration of the 5-HT₄ receptor agonist RS67333. The International Journal of Neuropsychopharmacology, 15(5), 631–643. https://doi.org/10.1017/s1461145711000782
- Patel M. (2016). Targeting oxidative stress in central nervous system disorders. Trends in Pharmacological Sciences, 37(9), 768–778. https://doi.org/10.1016/j.tips.2016.06.007
- Paton S. E., Menard C. (2023). Glutamate shall not pass: a mechanistic role for astrocytic O-GlcNAc transferase in stress and depression. Journal of Clinical Investigation, 133(7). https://doi.org/10.1172/jci168662
- Pereira C. F., Oliveira C. R. (2000). Oxidative glutamate toxicity involves mitochondrial dysfunction and perturbation of intracellular Ca2+ homeostasis. Neuroscience Research, 37(3), 227–236. https://doi.org/10.1016/s0168-0102(00)00124-3
- Picard K., Bisht K., Poggini S., Garofalo S., Golia M. T., Basilico B., Abdallah F., Ciano Albanese N., Amrein I., Vernoux N., Sharma K., Hui C. W., Savage J. C., Limatola C., Ragozzino D., Maggi L., Branchi I., Tremblay M. (2021). Microglial-glucocorticoid receptor depletion alters the response of hippocampal microglia and neurons in a chronic unpredictable mild stress paradigm in female mice. Brain, Behavior, and Immunity, 97, 423–439. https://doi.org/10.1016/j.bbi.2021.07.022
- Pittenger C., Coric V., Banasr M., Bloch M., Krystal J. H., Sanacora G. (2008). Riluzole in the treatment of mood and anxiety disorders. CNS Drugs, 22(9), 761–786. https://doi.org/10.2165/00023210-200822090-00004
- Pointer C. B., Klegeris A. (2017). Cardiolipin in central nervous system physiology and pathology. Cellular and Molecular Neurobiology, 37(7), 1161–1172. https://doi.org/10.1007/s10571-016-0458-9
- Popova N. K., Naumenko V. S. (2019). Neuronal and behavioral plasticity: The role of serotonin and BDNF systems tandem. Expert Opinion on Therapeutic Targets, 23(3), 227–239. https://doi.org/10.1080/14728222.2019.1572747
- Psarra A. M., Sekeris C. E. (2009). Glucocorticoid receptors and other nuclear transcription factors in mitochondria and possible functions. Biochimica et Biophysica Acta (BBA) - Bioenergetics, 1787(5), 431–436. https://doi.org/10.1016/j.bbabio.2008.11.011
- Qin Z., Shi D. D., Li W., Cheng D., Zhang Y. D., Zhang S., Tsoi B., Zhao J., Wang Z., Zhang Z. J. (2023). Berberine ameliorates depression-like behaviors in mice via inhibiting NLRP3 inflammasome-mediated neuroinflammation and preventing neuroplasticity disruption. Journal of Neuroinflammation, 20(1), 54. https://doi.org/10.1186/s12974-023-02744-7
- Qu Y., Yang C., Ren Q., Ma M., Dong C., Hashimoto K. (2017). Comparison of (R)-ketamine and lanicemine on depression-like phenotype and abnormal composition of gut microbiota in a social defeat stress model. Scientific Reports, 7(1), 15725. https://doi.org/10.1038/s41598-017-16060-7
- Racine N., McArthur B. A., Cooke J. E., Eirich R., Zhu J., Madigan S. (2021). Global prevalence of depressive and anxiety symptoms in children and adolescents during COVID-19: A meta-analysis. JAMA Pediatrics, 175(11), 1142–1150. https://doi.org/10.1001/jamapediatrics.2021.2482
- Rahman I., Marwick J., Kirkham P. (2004). Redox modulation of chromatin remodeling: Impact on histone acetylation and deacetylation, NF-kappaB and pro-inflammatory gene expression. Biochemical Pharmacology, 68(6), 1255–1267. https://doi.org/10.1016/j.bcp.2004.05.042
- Raison C. L., Dantzer R., Kelley K. W., Lawson M. A., Woolwine B. J., Vogt G., Spivey J. R., Saito K., Miller A. H. (2010). CSF Concentrations of brain tryptophan and kynurenines during immune stimulation with IFN-alpha: Relationship to CNS immune responses and depression. Molecular Psychiatry, 15(4), 393–403. https://doi.org/10.1038/mp.2009.116
- Ransohoff R. M., Schafer D., Vincent A., Blachere N. E., Bar-Or A. (2015). Neuroinflammation: Ways in which the immune system affects the brain. Neurotherapeutics, 12(4), 896–909. https://doi.org/10.1007/s13311-015-0385-3
- Ratajczak W., Rył A., Mizerski A., Walczakiewicz K., Sipak O., Laszczyńska M. (2019). Immunomodulatory potential of gut microbiome-derived short-chain fatty acids (SCFAs). Acta Biochimica Polonica, 66(1), 1–12. https://doi.org/10.18388/abp.2018_2648.
- Rawdin B. J., Mellon S. H., Dhabhar F. S., Epel E. S., Puterman E., Su Y., Burke H. M., Reus V. I., Rosser R., Hamilton S. P., Nelson J. C., Wolkowitz O. M. (2013). Dysregulated relationship of inflammation and oxidative stress in major depression. Brain, Behavior, and Immunity, 31, 143–152. https://doi.org/10.1016/j.bbi.2012.11.011
- Reigstad C. S., Salmonson C. E., Rainey J. F3rd, Szurszewski J. H., Linden D. R., Sonnenburg J. L., Farrugia G., Kashyap P. C. (2015). Gut microbes promote colonic serotonin production through an effect of short-chain fatty acids on enterochromaffin cells. The FASEB Journal, 29(4), 1395–1403. https://doi.org/10.1096/fj.14-259598
- Ren-Patterson R. F., Cochran L. W., Holmes A., Sherrill S., Huang S. J., Tolliver T., Lesch K. P., Lu B., Murphy D. L. (2005). Loss of brain-derived neurotrophic factor gene allele exacerbates brain monoamine deficiencies and increases stress abnormalities of serotonin transporter knockout mice. Journal of Neuroscience Research, 79(6), 756–771. https://doi.org/10.1002/jnr.20410
- Reynolds I. J., Hastings T. G. (1995). Glutamate induces the production of reactive oxygen species in cultured forebrain neurons following NMDA receptor activation. The Journal of Neuroscience, 15(5 Pt 1), 3318–3327. https://doi.org/10.1523/JNEUROSCI.15-05-03318.1995
- Riggs L. M., Gould T. D. (2021). Ketamine and the future of rapid-acting antidepressants. Annual Review of Clinical Psychology, 17, 207–231. https://doi.org/10.1146/annurev-clinpsy-072120-014126
- Rios C., Santamaria A. (1991). Quinolinic acid is a potent lipid peroxidant in rat brain homogenates. Neurochemical Research, 16(10), 1139–1143. https://doi.org/10.1007/bf00966592
- Rodriguez-Kern A., Gegelashvili M., Schousboe A., Zhang J., Sung L., Gegelashvili G. (2003). Beta-amyloid and brain-derived neurotrophic factor, BDNF, up-regulate the expression of glutamate transporter GLT-1/EAAT2 via different signaling pathways utilizing transcription factor NF-kappaB. Neurochemistry International, 43(4-5), 363–370. https://doi.org/10.1016/s0197-0186(03)00023-8
- Rudzki L., Maes M. (2020). The microbiota-gut-immune-glia (MGIG) axis in major depression. Molecular Neurobiology, 57(10), 4269–4295. https://doi.org/10.1007/s12035-020-01961-y
- Rudzki L., Maes M. (2021). From “leaky gut” to impaired glia-neuron communication in depression. Advances in Experimental Medicine and Biology, 1305, 129–155. https://doi.org/10.1007/978-981-33-6044-0_9
- Saarelainen T., Hendolin P., Lucas G., Koponen E., Sairanen M., MacDonald E., Agerman K., Haapasalo A., Nawa H., Aloyz R., Ernfors P., Castrén E. (2003). Activation of the TrkB neurotrophin receptor is induced by antidepressant drugs and is required for antidepressant-induced behavioral effects. The Journal of Neuroscience, 23(1), 349–357. https://doi.org/10.1523/jneurosci.23-01-00349.2003
- Samarajeewa A., Goldemann L., Vasefi M. S., Ahmed N., Gondora N., Khanderia C., Mielke J. G., Beazely M. A. (2014). 5-HT7 Receptor activation promotes an increase in TrkB receptor expression and phosphorylation. Frontiers in Behavioral Neuroscience, 8, 391. https://doi.org/10.3389/fnbeh.2014.00391
- Sanacora G., Zarate C. A., Krystal J. H., Manji H. K. (2008). Targeting the glutamatergic system to develop novel, improved therapeutics for mood disorders. Nature Reviews Drug Discovery, 7(5), 426–437. https://doi.org/10.1038/nrd2462
- Sanada K., Nakajima S., Kurokawa S., Barceló-Soler A., Ikuse D., Hirata A., Yoshizawa A., Tomizawa Y., Salas-Valero M., Noda Y., Mimura M., Iwanami A., Kishimoto T. (2020). Gut microbiota and major depressive disorder: A systematic review and meta-analysis. Journal of Affective Disorders, 266, 1–13. https://doi.org/10.1016/j.jad.2020.01.102
- Schwarcz R., Bruno J. P., Muchowski P. J., Wu H. Q. (2012). Kynurenines in the mammalian brain: When physiology meets pathology. Nature Reviews Neuroscience, 13(7), 465–477. https://doi.org/10.1038/nrn3257
- Serra-Millàs M. (2016). Are the changes in the peripheral brain-derived neurotrophic factor levels due to platelet activation? World Journal of Psychiatry, 6(1), 84–101. https://doi.org/10.5498/wjp.v6.i1.84
- Shandilya S., Kumar S., Kumar Jha N., Kumar Kesari K., Ruokolainen J. (2022). Interplay of gut microbiota and oxidative stress: Perspective on neurodegeneration and neuroprotection. Journal of Advanced Research, 38, 223–244. https://doi.org/10.1016/j.jare.2021.09.005
- Shirayama Y., Yang C., Zhang J. C., Ren Q., Yao W., Hashimoto K. (2015). Alterations in brain-derived neurotrophic factor (BDNF) and its precursor proBDNF in the brain regions of a learned helplessness rat model and the antidepressant effects of a TrkB agonist and antagonist. European Neuropsychopharmacology, 25(12), 2449–2458. https://doi.org/10.1016/j.euroneuro.2015.09.002
- Simon M. S., Arteaga-Henríquez G., Fouad Algendy A., Siepmann T., Illigens B. M. W. (2023). Anti-inflammatory treatment efficacy in major depressive disorder: A systematic review of meta-analyses. Neuropsychiatric Disease and Treatment, 19, 1–25. https://doi.org/10.2147/ndt.S385117
- Sivakumar V., Ling E. A., Lu J., Kaur C. (2010). Role of glutamate and its receptors and insulin-like growth factors in hypoxia induced periventricular white matter injury. Glia, 58(5), 507–523. https://doi.org/10.1002/glia.20940
- Soiza-Reilly M., Commons K. G. (2011). Glutamatergic drive of the dorsal raphe nucleus. Journal of Chemical Neuroanatomy, 41(4), 247–255. https://doi.org/10.1016/j.jchemneu.2011.04.004
- Stasi C., Sadalla S., Milani S. (2019). The relationship between the serotonin metabolism, gut-microbiota and the gut-brain axis. Current Drug Metabolism, 20(8), 646–655. https://doi.org/10.2174/1389200220666190725115503
- Stone T. W., Behan W. M., MacDonald M., Darlington L. G. (2000). Possible mediation of quinolinic acid-induced hippocampal damage by reactive oxygen species. Amino Acids, 19(1), 275–281. https://doi.org/10.1007/s007260070059
- Subramaniam S. R., Chesselet M. F. (2013). Mitochondrial dysfunction and oxidative stress in Parkinson’s disease. Progress in Neurobiology, 106-107, 17–32. https://doi.org/10.1016/j.pneurobio.2013.04.004
- Sun N., Qin Y. J., Xu C., Xia T., Du Z. W., Zheng L. P., Li A. A., Meng F., Zhang Y., Zhang J., Liu X., Li T. Y., Zhu D. Y., Zhou Q. G. (2022). Design of fast-onset antidepressant by dissociating SERT from nNOS in the DRN. Science, 378(6618), 390–398. https://doi.org/10.1126/science.abo3566
- Takeuchi H., Jin S., Wang J., Zhang G., Kawanokuchi J., Kuno R., Sonobe Y., Mizuno T., Suzumura A. (2006). Tumor necrosis factor-alpha induces neurotoxicity via glutamate release from hemichannels of activated microglia in an autocrine manner. Journal of Biological Chemistry, 281(30), 21362–21368. https://doi.org/10.1074/jbc.M600504200
- Tauffenberger A., Magistretti P. J. (2021). Reactive oxygen species: Beyond their reactive behavior. Neurochemical Research, 46(1), 77–87. https://doi.org/10.1007/s11064-020-03208-7
- Tavares R. G., Tasca C. I., Santos C. E., Alves L. B., Porciúncula L. O., Emanuelli T., Souza D. O. (2002). Quinolinic acid stimulates synaptosomal glutamate release and inhibits glutamate uptake into astrocytes. Neurochemistry International, 40(7), 621–627. https://doi.org/10.1016/s0197-0186(01)00133-4
- Taylor S., Srinivasan B., Wordinger R. J., Roque R. S. (2003). Glutamate stimulates neurotrophin expression in cultured Muller cells. Molecular Brain Research, 111(1-2), 189–197. https://doi.org/10.1016/s0169-328x(03)00030-5
- Tette F. M., Kwofie S. K., Wilson M. D. (2022). Therapeutic anti-depressant potential of microbial GABA produced by Lactobacillus rhamnosus strains for GABAergic signaling restoration and inhibition of addiction-induced HPA axis hyperactivity. Current Issues in Molecular Biology, 44(4), 1434–1451. https://doi.org/10.3390/cimb44040096
- Theo V., Stephen S. L., Cristiana A. (2020). Global burden of 369 diseases and injuries in 204 countries and territories, 1990-2019: A systematic analysis for the Global Burden Of Disease Study 2019. The Lancet, 396(10258), 1204–1222. https://doi.org/10.1016/s0140-6736(20)30925-9
- Tilleux S., Hermans E. (2007). Neuroinflammation and regulation of glial glutamate uptake in neurological disorders. Journal of Neuroscience Research, 85(10), 2059–2070. https://doi.org/10.1002/jnr.21325
- Todd A. C., Hardingham G. E. (2020). The regulation of astrocytic glutamate transporters in health and neurodegenerative diseases. International Journal of Molecular Sciences, 21(24), 9607. https://doi.org/10.3390/ijms21249607
- Troubat R., Barone P., Leman S., Desmidt T., Cressant A., Atanasova B., Brizard B., El Hage W., Surget A., Belzung C., Camus V. (2021). Neuroinflammation and depression: A review. European Journal of Neuroscience, 53(1), 151–171. https://doi.org/10.1111/ejn.14720
- Vahid-Ansari F., Albert P. R. (2021). Rewiring of the serotonin system in major depression. Frontiers in Psychiatry, 12, 802581. https://doi.org/10.3389/fpsyt.2021.802581
- Vašíček O., Lojek A., Číž M. (2020). Serotonin and its metabolites reduce oxidative stress in murine RAW264.7 macrophages and prevent inflammation. Journal of Physiology and Biochemistry, 76(1), 49–60. https://doi.org/10.1007/s13105-019-00714-3
- Visentin A. P. V., Colombo R., Scotton E., Fracasso D. S., da Rosa A. R., Branco C. S., Salvador M. (2020). Targeting inflammatory-mitochondrial response in major depression: Current evidence and further challenges. Oxidative Medicine and Cellular Longevity, 2020, 2972968. https://doi.org/10.1155/2020/2972968
- Volterra A., Trotti D., Floridi S., Racagni G. (1994). Reactive oxygen species inhibit high-affinity glutamate uptake: Molecular mechanism and neuropathological implications. Annals of the New York Academy of Sciences, 738, 153–162. https://doi.org/10.1111/j.1749-6632.1994.tb21800.x
- Wang D., Wu J., Zhu P., Xie H., Lu L., Bai W., Pan W., Shi R., Ye J., Xia B., Zhao Z., Wang Y., Liu X., Zhao B. (2022). Tryptophan-rich diet ameliorates chronic unpredictable mild stress induced depression- and anxiety-like behavior in mice: The potential involvement of gut-brain axis. Food Research International, 157, 111289. https://doi.org/10.1016/j.foodres.2022.111289
- Wang H., Jin M., Xie M., Yang Y., Xue F., Li W., Zhang M., Li Z., Li X., Jia N., Liu Y., Cui X., Hu G., Dong L., Wang G., Yu Q. (2023). Protective role of antioxidant supplementation for depression and anxiety: A meta-analysis of randomized clinical trials. Journal of Affective Disorders, 323, 264–279. https://doi.org/10.1016/j.jad.2022.11.072
- Wei X., Ma Y., Li F., He H., Huang H., Huang C., Chen Z., Chen D., Chen J., Yuan X. (2021). Acute diallyl disulfide administration prevents and reveres lipopolysaccharide-induced depression-like behaviors in mice via regulating neuroinflammation and oxido-nitrosative stress. Inflammation, 44(4), 1381–1395. https://doi.org/10.1007/s10753-021-01423-0
- Więdłocha M., Marcinowicz P., Krupa R., Janoska-Jaździk M., Janus M., Dębowska W., Mosiołek A., Waszkiewicz N., Szulc A. (2018). Effect of antidepressant treatment on peripheral inflammation markers-a meta-analysis. Progress in Neuro-Psychopharmacology and Biological Psychiatry, 80(Pt C), 217–226. https://doi.org/10.1016/j.pnpbp.2017.04.026
- Winter G., Hart R. A., Charlesworth R. P. G., Sharpley C. F. (2018). Gut microbiome and depression: What we know and what we need to know. Reviews in the Neurosciences, 29(6), 629–643. https://doi.org/10.1515/revneuro-2017-0072
- Woodburn S. C., Asrat H. S., Flurer J. K., Schwierling H. C., Bollinger J. L., Vollmer L. L., Wohleb E. S. (2023). Depletion of microglial BDNF increases susceptibility to the behavioral and synaptic effects of chronic unpredictable stress. Brain, Behavior, and Immunity, 109, 127–138. https://doi.org/10.1016/j.bbi.2023.01.014
- Woolston C., O’Meara S. (2019). Phd students in China report misery and hope. Nature, 575(7784), 711–713. https://doi.org/10.1038/d41586-019-03631-z
- Wu A., Ying Z., Gomez-Pinilla F. (2004). The interplay between oxidative stress and brain-derived neurotrophic factor modulates the outcome of a saturated fat diet on synaptic plasticity and cognition. European Journal of Neuroscience, 19(7), 1699–1707. https://doi.org/10.1111/j.1460-9568.2004.03246.x
- Wu H., Friedman W. J., Dreyfus C. F. (2004). Differential regulation of neurotrophin expression in basal forebrain astrocytes by neuronal signals. Journal of Neuroscience Research, 76(1), 76–85. https://doi.org/10.1002/jnr.20060
- Xie X., Shen Q., Yu C., Xiao Q., Zhou J., Xiong Z., Li Z., Fu Z. (2020). Depression-like behaviors are accompanied by disrupted mitochondrial energy metabolism in chronic corticosterone-induced mice. The Journal of Steroid Biochemistry and Molecular Biology, 200, 105607. https://doi.org/10.1016/j.jsbmb.2020.105607
- Xie X., Yu C., Zhou J., Xiao Q., Shen Q., Xiong Z., Li Z., Fu Z. (2020). Nicotinamide mononucleotide ameliorates the depression-like behaviors and is associated with attenuating the disruption of mitochondrial bioenergetics in depressed mice. Journal of Affective Disorders, 263, 166–174. https://doi.org/10.1016/j.jad.2019.11.147
- Xiong W., Cao X., Zeng Y., Qin X., Zhu M., Ren J., Wu Z., Huang Q., Zhang Y., Wang M., Chen L., Turecki G., Mechawar N., Chen W., Yi G., Zhu X. (2019). Astrocytic epoxyeicosatrienoic acid signaling in the medial prefrontal cortex modulates depressive-like behaviors. The Journal of Neuroscience, 39(23), 4606–4623. https://doi.org/10.1523/jneurosci.3069-18.2019
- Yagasaki Y., Numakawa T., Kumamaru E., Hayashi T., Su T. P., Kunugi H. (2006). Chronic antidepressants potentiate via sigma-1 receptors the brain-derived neurotrophic factor-induced signaling for glutamate release. Journal of Biological Chemistry, 281(18), 12941–12949. https://doi.org/10.1074/jbc.M508157200
- Yager S., Forlenza M. J., Miller G. E. (2010). Depression and oxidative damage to lipids. Psychoneuroendocrinology, 35(9), 1356–1362. https://doi.org/10.1016/j.psyneuen.2010.03.010
- Yang C., Fujita Y., Ren Q., Ma M., Dong C., Hashimoto K. (2017). Bifidobacterium in the gut microbiota confer resilience to chronic social defeat stress in mice. Scientific Reports, 7, 45942. https://doi.org/10.1038/srep45942
- Yang F., Wang H., Chen H., Ran D., Tang Q., Weng P., Sun Y., Jiang W. (2020). RAGE Signaling pathway in hippocampus dentate gyrus involved in GLT-1 decrease induced by chronic unpredictable stress in rats. Brain Research Bulletin, 163, 49–56. https://doi.org/10.1016/j.brainresbull.2020.06.020
- Yardeni T., Tanes C. E., Bittinger K., Mattei L. M., Schaefer P. M., Singh L. N., Wu G. D., Murdock D. G., Wallace D. C. (2019). Host mitochondria influence gut microbiome diversity: A role for ROS. Science Signaling, 12(588), eaaw3159. https://doi.org/10.1126/scisignal.aaw3159
- Ye Q., Lin S. S., Ulrich H., Tang Y. (2023). Decoupling SERT-nNOS interaction to generate fast-onset antidepressants. Neuroscience Bulletin. https://doi.org/10.1007/s12264-023-01049-2
- Yoo J. M., Lee B. D., Sok D. E., Ma J. Y., Kim M. R. (2017). Neuroprotective action of N-acetyl serotonin in oxidative stress-induced apoptosis through the activation of both TrkB/CREB/BDNF pathway and Akt/Nrf2/Antioxidant enzyme in neuronal cells. Redox Biology, 11, 592–599. https://doi.org/10.1016/j.redox.2016.12.034
- Yu M., Jia H., Zhou C., Yang Y., Zhao Y., Yang M., Zou Z. (2017). Variations in gut microbiota and fecal metabolic phenotype associated with depression by 16S rRNA gene sequencing and LC/MS-based metabolomics. Journal of Pharmaceutical and Biomedical Analysis, 138, 231–239. https://doi.org/10.1016/j.jpba.2017.02.008
- Yu Y. B., Zhao D. Y., Qi Q. Q., Long X., Li X., Chen F. X., Zuo X. L. (2017). BDNF modulates intestinal barrier integrity through regulating the expression of tight junction proteins. Neurogastroenterology & Motility, 29(3), e12967. https://doi.org/10.1111/nmo.12967
- Zafir A., Banu N. (2007). Antioxidant potential of fluoxetine in comparison to Curcuma longa in restraint-stressed rats. European Journal of Pharmacology, 572(1), 23–31. https://doi.org/10.1016/j.ejphar.2007.05.062
- Zhang B. (2017). Consequences of early adverse rearing experience (EARE) on development: Insights from non-human primate studies. Zool Res, 38(1), 7–35. https://doi.org/10.13918/j.issn.2095-8137.2017.002.
- Zhang B., Li C. Y., Wang X. S. (2017). The effect of hippocampal NMDA receptor blockade by MK-801 on cued fear extinction. Behavioural Brain Research, 332, 200–203. https://doi.org/10.1016/j.bbr.2017.05.067
- Zhang B., Xiong F., Ma Y., Li B., Mao Y., Zhou Z., Yu H., Li J., Li C., Fu J., Wang J., Zhao X. (2019). Chronic phencyclidine treatment impairs spatial working memory in rhesus monkeys. Psychopharmacology, 236(7), 2223–2232. https://doi.org/10.1007/s00213-019-05214-2
- Zhang J. C., Wu J., Fujita Y., Yao W., Ren Q., Yang C., Li S. X., Shirayama Y., Hashimoto K. (2014). Antidepressant effects of TrkB ligands on depression-like behavior and dendritic changes in mice after inflammation. International Journal of Neuropsychopharmacology, 18(4). https://doi.org/10.1093/ijnp/pyu077.
- Zhang J. C., Yao W., Dong C., Yang C., Ren Q., Ma M., Han M., Hashimoto K. (2015). Comparison of ketamine, 7,8-dihydroxyflavone, and ANA-12 antidepressant effects in the social defeat stress model of depression. Psychopharmacology, 232(23), 4325–4335. https://doi.org/10.1007/s00213-015-4062-3
- Zhang J. C., Yao W., Hashimoto K. (2016). Brain-derived neurotrophic factor (BDNF)-TrkB signaling in inflammation-related depression and potential therapeutic targets. Current Neuropharmacology, 14(7), 721–731. https://doi.org/10.2174/1570159x14666160119094646
- Zhang Y., Huang R., Cheng M., Wang L., Chao J., Li J., Zheng P., Xie P., Zhang Z., Yao H. (2019). Gut microbiota from NLRP3-deficient mice ameliorates depressive-like behaviors by regulating astrocyte dysfunction via circHIPK2. Microbiome, 7(1), 116. https://doi.org/10.1186/s40168-019-0733-3
- Zhang Z., Fan J., Ren Y., Zhou W., Yin G. (2013). The release of glutamate from cortical neurons regulated by BDNF via the TrkB/Src/PLC-γ1 pathway. Journal of Cellular Biochemistry, 114(1), 144–151. https://doi.org/10.1002/jcb.24311
- Zhao D. Y., Zhang W. X., Qi Q. Q., Long X., Li X., Yu Y. B., Zuo X. L. (2018). Brain-derived neurotrophic factor modulates intestinal barrier by inhibiting intestinal epithelial cells apoptosis in mice. Physiological Research, 67(3), 475–485. https://doi.org/10.33549/physiolres.933641
- Zheng G., Wu S. P., Hu Y., Smith D. E., Wiley J. W., Hong S. (2013). Corticosterone mediates stress-related increased intestinal permeability in a region-specific manner. Neurogastroenterology & Motility, 25(2), e127–e139. https://doi.org/10.1111/nmo.12066
- Zhou R., Yazdi A. S., Menu P., Tschopp J. (2011). A role for mitochondria in NLRP3 inflammasome activation. Nature, 469(7329), 221–225. https://doi.org/10.1038/nature09663
- Zhou Y., Hassel B., Eid T., Danbolt N. C. (2019). Axon-terminals expressing EAAT2 (GLT-1; Slc1a2) are common in the forebrain and not limited to the hippocampus. Neurochemistry International, 123, 101–113. https://doi.org/10.1016/j.neuint.2018.03.006
- Zhu C. B., Blakely R. D., Hewlett W. A. (2006). The proinflammatory cytokines interleukin-1beta and tumor necrosis factor-alpha activate serotonin transporters. Neuropsychopharmacology, 31(10), 2121–2131. https://doi.org/10.1038/sj.npp.1301029
- Zhu X., Sakamoto S., Ishii C., Smith M. D., Ito K., Obayashi M., Unger L., Hasegawa Y., Kurokawa S., Kishimoto T., Li H., Hatano S., Wang T. H., Yoshikai Y., Kano S. I., Fukuda S., Sanada K., Calabresi P. A., Kamiya A. (2023). Dectin-1 signaling on colonic γδ T cells promotes psychosocial stress responses. Nature Immunology, 24(4), 625–636. https://doi.org/10.1038/s41590-023-01447-8
- Zhu X. N., Li J., Qiu G. L., Wang L., Lu C., Guo Y. G., Yang K. X., Cai F., Xu T., Yuan T. F., Hu J. (2023). Propofol exerts anti-anhedonia effects via inhibiting the dopamine transporter. Neuron, 111(10), 1626–1636.e6. https://doi.org/10.1016/j.neuron.2023.02.017
- Zlatković J., Filipović D. (2013). Chronic social isolation induces NF-κB activation and upregulation of iNOS protein expression in rat prefrontal cortex. Neurochemistry International, 63(3), 172–179. https://doi.org/10.1016/j.neuint.2013.06.002
- Zlatković J., Todorović N., Bošković M., Pajović S. B., Demajo M., Filipović D. (2014). Different susceptibility of prefrontal cortex and hippocampus to oxidative stress following chronic social isolation stress. Molecular and Cellular Biochemistry, 393(1-2), 43–57. https://doi.org/10.1007/s11010-014-2045-z
- Zou J., Crews F. (2006). CREB And NF-kappaB transcription factors regulate sensitivity to excitotoxic and oxidative stress induced neuronal cell death. Cellular and Molecular Neurobiology, 26(4-6), 385–405. https://doi.org/10.1007/s10571-006-9045-9
- Zou W., Feng R., Yang Y. (2018). Changes in the serum levels of inflammatory cytokines in antidepressant drug-naive patients with major depression. PLoS One, 13(6), e0197267. https://doi.org/10.1371/journal.pone.0197267