Abstract
Chiral amines are essential motifs in pharmaceuticals, agrochemicals, and specialty chemicals. While traditional chemical routes to chiral amines often lack stereoselectivity and require harsh conditions, biocatalytic methods using engineered enzymes can offer high efficiency and selectivity under sustainable conditions. This review discusses recent advances in protein engineering of transaminases, oxidases, and other enzymes to improve catalytic performance. Strategies such as directed evolution, immobilization, and computational redesign have expanded substrate scope and enhanced efficiency. Furthermore, process optimization guided by techno-economic assessments has been crucial for establishing viable biomanufacturing routes. Combining state-of-the-art enzyme engineering with multifaceted process development will enable scalable, economical enzymatic synthesis of diverse chiral amine targets.
Introduction
Chiral amines are foundational building blocks in many high-value molecules, including over 40% of commercial pharmaceuticals.Citation1 They have a high diversity in their structure and are involved in an array of biological activities due to their tendency to form hydrogen bonds. Most biocatalysts synthesize primary amines. However, the motif in many chemicals and pharmaceuticals comprises secondary and tertiary amines. Prominent examples are the anti-diabetic sitagliptin,Citation2 antifungal Posaconazole, narcotic analgesic Methadone, hyperparathyroidism drug Cinacalcet, anti-Alzheimer’s Rivastigmine,Citation3 anti-malarial drug Larium, anti-TB drug Ethambutol, HIV protease inhibitor Lopinavir, antiretroviral agent MaravirocCitation4 and anticancer agent Crizotinib (). Applications also extend to agrochemicals, natural products, and specialty chemicals. However, chemical synthesis of enantiopure chiral amines has remained an enduring challenge.Citation5
Conventional routes rely heavily on resolution of racemates or transition metal catalysis, often necessitating harsh conditions.Citation6 Resolution is capped at 50% theoretical yield for each enantiomer, while metal catalysis requires high-pressure hydrogen gas, expensive catalysts, and extensive purification generating metal waste.Citation7 Such drawbacks motivate developing alternative, sustainable processes.
Biocatalysis offers a promising solution, utilizing engineered enzymes to impart high chemo-, regio-, and stereoselectivity under mild, aqueous conditions.Citation8 Yet realizing industrial implementation requires expanding substrate scope, improving catalytic efficiency, and ensuring process economic viability ().
Computational tools like molecular docking and bioinformatics have become indispensable in guiding enzyme engineering efforts. Molecular docking predicts the binding orientation and affinity of substrates or inhibitors with the enzyme active site, providing valuable insights for rational design. Tools like AutoDock,Citation9 GOLD,Citation10 and GlideCitation11 have been widely used to study enzyme-substrate interactions and identify key residues for mutagenesis. Bioinformatics approaches, such as sequence alignment,Citation12 homology modelling,Citation13 and phylogenetic analysis,Citation14 have also been crucial in understanding structure-function relationships and identifying promising enzyme candidates from diverse sources.
Recent advancements in computational tools, particularly in the field of machine learning and artificial intelligence, have revolutionized the way researchers approach protein structure prediction and enzyme engineering. The ability to accurately predict protein structures from amino acid sequences has opened up new avenues for rational enzyme design and optimization. Several patent applications highlight the potential of these cutting-edge technologies in determining protein structures and guiding enzyme engineering efforts.
For instance, WO2020058177A1 describes methods and systems for performing protein structure prediction using a geometry neural network. The method involves iteratively determining alternative predicted structures of a given protein and processing a network input comprising the amino acid sequence and structure parameters to generate an output characterizing the similarity between the predicted and actual protein structures. Similarly, WO2020058174A1 discloses methods for protein structure prediction and domain segmentation by generating multiple predicted structures and iteratively updating structure parameters using gradient-based optimization of a quality score. Another patent application, WO2020058176, focuses on generating distance maps for a given protein by characterizing estimated distances between amino acid residues in the structure using distance map crops.
These innovative computational approaches, combined with experimental methods, have the potential to accelerate the discovery and engineering of enzymes with improved stability, activity, and specificity. By leveraging the power of machine learning and AI, researchers can explore vast sequence spaces, identify promising enzyme candidates, and guide rational design efforts. As these technologies continue to advance, their integration into enzyme engineering workflows will undoubtedly lead to the development of highly efficient biocatalysts for diverse applications, including the synthesis of chiral amines and other high-value compounds.
The advent of AlphaFold, a groundbreaking AI tool for protein structure prediction, has revolutionized the field of enzyme engineering.Citation15 By accurately predicting 3D structures from amino acid sequences, AlphaFold enables the rapid characterization of novel enzymes and the design of improved variants. This is particularly valuable for exploring the vast sequence space of uncharacterized enzymes from metagenomics and extremophile sources. Integration of AlphaFold with docking and molecular dynamics simulations can provide unprecedented insights into enzyme-substrate interactions and guide rational design.
This review will discuss advances in engineering key enzymes like transaminases and oxidases. It will also highlight crucial process development factors including enzyme immobilization, cofactor recycling, and techno-economic assessments.
Engineering Amine Oxidases
Amine oxidases catalyze the oxidative deamination of amines into imines while generating hydrogen peroxide. They have shown excellent potential for deracemization of chiral amines when coupled to non-selective chemical reducing agents.Citation16 However, most oxidases have narrow substrate scope limited to small aliphatic amines. Ghislieri et al employed directed evolution to engineer monoamine oxidase from Aspergillus niger (MAO-N) for bulkier aromatic substrates.Citation17 Mutagenesis using rational design and directed evolution creates changes in the amino acid-enhancing catalytic pocket and provides insight for creating novel variants of enzymes ().
Wild-type MAO-N was only active on small amines like butylamine and pentylamine. Alexeeva et al used random mutagenesis and screening to improve the activity of an enzyme toward methylbenzylamine. After several rounds, they identified a mutant containing the mutation Asn336Ser which exhibited a 50-fold increase in catalytic turnover (kcat) compared to the wild-type enzyme.Citation18 Further directed evolution of the Asn336Ser mutant yielded a triple mutant, Asn336Ser/Met348Lys/Ile246Met (designated MAO-3N), that exhibited expanded substrate scope.Citation19 This variant showed good general activity toward a range of chiral primary amines. Additionally, MAO-3N gained the ability to accept S-α-methylbenzylamine as a substrate and displayed improved turnover of chiral secondary amines including methyltetrahydroisoquinoline.Citation19 Further directed evolution experiments by Dunsmore led to the development of mutant MAO-5N, which was selected based on its acquired activity toward cyclic tertiary amines including N-methyl-2-phenylpyrrolidine.Citation20
Engineering Stereoselective Transaminases
Amine transaminases catalyze transfer of an amine group from a donor substrate to a prochiral ketone acceptor using pyridoxal 5’-phosphate (PLP) as an essential cofactor. Their high stereoselectivity makes transaminases ideal for chiral amine synthesis.Citation21 However, wild-type enzymes are mostly limited to small aliphatic amines. Extensive protein engineering has created transaminase variants capable of bulky, aromatic substrates. Transaminases have been applied to prepare complex pharmaceutical intermediates.Citation22
Bacterial transaminases continue to emerge as versatile biocatalysts for the production of enantiopure chiral amines. An (R)-selective transaminase from Arthrobacter sp. KNK168 was characterized for its ability to aminate prochiral ketones using whole cells as an amine donor.Citation23 Optimization of culture conditions enhanced transaminase production through inducer supplementation. In amination reactions, (R)-1-phenylethylamine served as an efficient amino donor, converting 3,4-dimethoxyphenylacetone to (R)-3,4-dimethoxyamphetamine in 82% yield and >99% enantiomeric excess (ee) under optimized conditions. Additionally, various substituted acetophenones underwent asymmetric amination to give the corresponding (R)-amines with excellent stereoselectivity. Such transaminase-mediated syntheses highlight the potential of biocatalysis for scalable and sustainable production of pharmaceutically relevant chiral amines.
A seminal example involved engineering Arthrobacter transaminases for asymmetric synthesis of sitagliptin (), the active pharmaceutical in Januvia®. Savile et alCitation24 first identified Arthrobacter strains with sitagliptin precursor activity by a substrate walking, modeling, and mutation approach to create a transaminase with marginal activity for the synthesis of the chiral amine, which was then further engineered via directed evolution for practical application in a manufacturing setting. Since wild-type yields were very low (<15%), the activity toward sitagliptin ketone was established in the library designs by opening the small pocket to accommodate the trifluorophenyl group. The starting transaminase (ATA-117) was modeled to have two binding pockets - a large pocket that accommodates the tetrahydro-triazolo[4,3-a]pyrazine (THTP) group of the substrate, and a small pocket predicted to be too constrained to fit the trifluorophenyl group. Four residues were predicted to line the small pocket of the binding site: V69, F122, T283, and A284. All of these residues and adjacent F70 were subjected to saturation mutagenesis. Saturation mutagenesis of residues in the large pocket, especially S223P, improved activity towards an intermediate methyl ketone substrate. Mutations in the small pocket like V69G, F122I, and A284G were designed to open up space and reduce steric clashes with the trifluorophenyl group. This enabled the enzyme to process the actual prositagliptin ketone substrate. Mutations in the small pocket like V69G, F122I, and A284G were designed to open up space and reduce steric clashes with the trifluorophenyl group. This enabled the enzyme to process the actual prositagliptin ketone substrate. The variant identified with activity toward pro-sitagliptin ketone had three programmed mutations (V69G, F122I, and A284G) as well as two random mutations (Y26H and V65A). After recombination of beneficial mutations from both binding pockets, the best variant in round 2 contained 12 mutations and showed 75-fold increased activity over the parent. Further rounds of directed evolution under increasingly harsh reaction conditions yielded a final variant with 27 mutations and ~27,000-fold total improvement in activity. The final enzyme variant enabled the amination of 200 g/L prositagliptin ketone substrate with 92% isolated yield of sitagliptin product in >99.95% ee. No detectable formation of the minor enantiomer was observed, indicating excellent stereocontrol and selectivity of the engineered transaminase. These findings are further supported by patents from Codexis Inc. (US8293507B2 and US8921079B2), which describe engineered transaminase variants from Arthrobacter sp. KNK168 with improved activity and stereoselectivity for sitagliptin synthesis.
Midelfort et al report the engineering of the Vibrio fluvialis aminotransferase (Vfat) enzyme for improved activity and selectivity in the synthesis of (3S,5R)-ethyl 3-amino-5-methyloctanoate, a key intermediate in the production of the investigative drug imagabalin for treating generalized anxiety disorder.Citation25 The engineered Vfat enzyme is S-selective for the amination reaction to generate (3S,5R)-ethyl 3-amino-5-methyloctanoate. Through an integrated approach combining homology modeling, bioinformatics, site saturation mutagenesis, and directed evolution, the authors identified beneficial mutations including W57F, F85A, V153A, and I259V. By recombining these mutations, they generated a variant containing eight mutations (F19W/ W57F/ F85A/ R88K/ V153A/ K163F/ I259V/ R415F) that improved the initial velocity of Vfat 60-fold for the desired transamination reaction. X-ray crystal structures provided insights into how the mutations expanded the binding pocket to better accommodate the substrate. This engineered Vfat enabled scalable enzymatic synthesis of the imagabalin intermediate, demonstrating the power of protein engineering for pharmaceutical production.
Overall, recent advances demonstrate that laboratory evolution can substantially improve transaminase activity, expand substrate scope, and even invert stereopreference. Combining computational modeling with high-throughput screening has accelerated generation of superior biocatalysts. Tailored enzyme engineering guided by structural insights and process considerations will continue unlocking wider application of transaminases.
However, as reversible catalysts, transaminases can suffer from unfavorable equilibria limiting amine yields. Strategies to enhance product formation include excess amine donor, sparging to remove acetone from isopropylamine processes, or “smart” diamine donors that spontaneously cyclize upon deamination. Gomm et al report an effective strategy to overcome reaction equilibria limitations in transaminase-catalyzed synthesis of chiral amines using diamine donors like 1,5-diaminopentane (cadaverine).Citation26 With only 1–3 equivalents of these “smart” donors, an engineered transaminase achieved excellent conversions and stereoselectivity for various prochiral ketones, significantly outperforming iso-propylamine. The diamine by-products likely undergo irreversible cyclization and trimerization. The findings provide a promising biocatalytic approach to displace challenging equilibria and enable scalable production of pharmaceutically relevant chiral amines from inexpensive and readily available chemicals. Metabolic engineering to generate the diamine donors in situ further offers the potential for self-sufficient fermentation processes for sustainable chiral amine synthesis.
Cascades that consume the amine product in situ also help overcome thermodynamic constraints. Transaminases have been combined with various enzymes in cascades, including alanine dehydrogenase, lactate dehydrogenase, pyruvate dehydrogenase, alcohol dehydrogenase, carboxylic acid reductase, monoamine oxidase, and imine reductase.Citation27 Although widely used industrially, transaminases now face competition from emerging amine dehydrogenases and imine reductases that can generate secondary and tertiary amines.Citation28
Engineering Dehydrogenases
Amine dehydrogenases catalyze the synthesis of chiral amines from aldehyde or ketone using reductive amination. Ammonia is an amino donor in this process, and NAD(P)H acts as a co-factor.Citation29
Enzyme engineering has enabled the development of amine dehydrogenases (AmDHs) for chiral amine synthesis. Bommarius et al engineered phenylalanine dehydrogenase (BbPheDH) from Bacillus badius and leucine dehydrogenase (BsLeuDH) from Bacillus stearothermophilus by mutating the carboxylate pockets of the enzymes.Citation30 This allowed the enzymes to accept ketones rather than α-keto acids as substrates, with excellent stereoselectivity. Substituting polar residues with non-polar amino acids at positions 68 and 261 of LeuDH (K68S/N261L) altered substrate specificity from keto acids to ketones. One engineered variant (K68T/N261L) synthesized (S)-ethambutol, an anti-tuberculosis drug precursor. However, some ketones were still too bulky to bind properly.Citation31 Mutating two large residues (A113G/T134G) along with K68S/N261L enabled conversion of α-hydroxy ketones to (S)-configured vicinal amino alcohols with 99% ee. The most active variant (L-AmDH; K68S/E114V/N261L/V291C) converted 2-methylbutanone to (R)-2-methylbutylamine with 92.5% conversion and 99.8% e.e. These AmDH variants displayed broad ketone substrate scope, although they lost native leucine activity.Citation32
Studies on other amino acid dehydrogenases like BbPheDH (48% sequence similarity to LeuDH) revealed conserved active site residues amenable to homologous mutation. The BbPheDH variant K77M/N276V showed activity towards aromatic ketones like p-fluorophenylacetone, converting it to (S)-1-(4-fluorophenyl)propylamine. Further mutagenesis improved catalytic efficiency 15-fold (kcat 2.8 s−1).Citation33 The Rhodococcus sp. M4 PhDH variant K66Q/S149G/N262C catalyzed 4-phenyl-2-butanone to (R)-1-methyl-3-phenylpropylamine with 98% e.e. Co-factor regeneration was achieved by coupling with glucose/formate dehydrogenase.Citation34 Immobilization of AmDHs on magnetic nanoparticles enabled enzyme reuse up to three cycles with 81% residual activity.Citation35 Jiang et al engineered Bacillus halodurans PheAmDH for activity towards benzylic and aliphatic ketones. The E113D/N276L variant showed 6-fold increased catalytic efficiency via enhanced oxidative deamination and reductive amination. Thermostable AmDHs were developed using Caldalkalibacilus thermarum PhDH as template, increasing melting temperature to 83.5°C.Citation36
Engineered amino acid dehydrogenases have enabled efficient biocatalytic synthesis of pharmaceutical intermediates. An example is the production of (S)-N-boc-3-hydroxyadamantylglycine, a key intermediate in the synthesis of saxagliptin, an anti-diabetic drug.Citation37 Phenylalanine dehydrogenase from Thermoactinomyces intermedius was modified by altering two amino acids and adding a 12 amino acid C-terminal extension. The engineered enzyme catalyzed the reductive amination of 2-(3-Hydroxy-1-Adamantyl)-2-Oxoethanoic acid to generate the desired amino acid product in high yield. To regenerate the NAD(P)H cofactor, formate dehydrogenase was coupled to the system.Citation38 For process intensification, the modified phenylalanine dehydrogenase and formate dehydrogenase were co-expressed in recombinant E. coli or Pichia pastoris. Scale-up using P. pastoris cell extracts gave nearly quantitative yields and 100% ee. Protection of the amine as a boc derivative enabled efficient biotransformation. Overall, these studies demonstrate the utility of engineered dehydrogenases to accomplish asymmetric synthesis of complex pharmaceutical intermediates at preparative scale. Further optimization of enzyme activity, cofactor recycling, and process engineering will enable more sustainable manufacture of chiral amine drugs.
Advanced Enzyme Immobilization
Enzyme immobilization onto heterogeneous solid materials can enhance stability, prevent aggregation, simplify product separation, and enable reuse. Solid supports are crucial in stabilizing the enzymes’ three-dimensional structure, which helps preserve their catalytic activity. One of the main objectives of immobilizing enzymes is to make it easier to retrieve them from aqueous solutions efficiently. These advantages make immobilization strategies crucial, especially for industrial-scale chiral amine synthesis. Enzyme immobilization consists of two methods, ie, physical and chemical methods based on the enzyme and solid support nature ().
Figure 5 Enzyme Immobilization- An approach for improving enzyme efficiency for chiral amine synthesis.
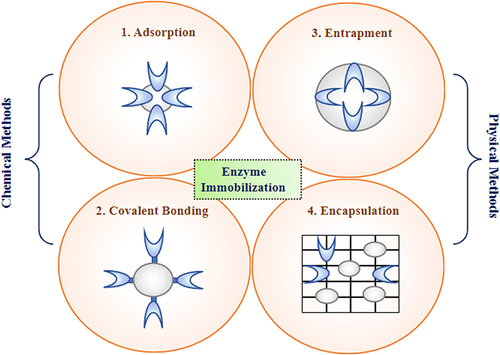
Advancements such as directed evolution and rational design have expanded the substrate scope and thermostability of ω-transaminases (ω-TAs). However, recovering soluble enzymes from the reaction mixture remains challenging, necessitating multiple downstream processes.Citation39 Fresh batches of enzymes are required for each Sitagliptin synthesis cycle, hampering industrial application due to a lack of long-term operational stability and difficulties in enzyme recovery and reuse. Immobilization techniques are necessary to improve stability, enable enzyme reuse, and facilitate product recovery.Citation39
Enzyme immobilization onto heterogeneous solid materials can enhance stability, prevent aggregation, simplify product separation, and enable reuse.Citation40 Solid supports stabilize the three-dimensional structure of enzymes, preserving their catalytic activity. Immobilization also facilitates efficient enzyme retrieval from aqueous solutions. These advantages make immobilization strategies crucial for industrial-scale chiral amine synthesis. Enzyme immobilization can be achieved through physical methods, such as entrapment and encapsulation, or chemical methods involving covalent binding to solid matrices.
Recent studies have demonstrated the benefits of immobilization. Immobilizing Burkholderia phytofirmans transaminase on agarose beads provided remarkable solvent stability, with the biocatalyst retaining >80% initial activity after 24 hours in 50% methanol or acetone.Citation41 The agarose-bound transaminase catalyzed repeated amine synthesis cycles without loss of enantioselectivity. Engineered Arthrobacter transaminase immobilized on cross-linked polystyrene resin exhibited high activity in neat organic solvents, enabling 100 g/L substrate loading. Immobilization also increased thermal stability, extending half-life at 45°C from 90 minutes for a soluble enzyme to nearly 7 days.Citation42 Patents from Merck & Co. Inc. (US9587229B2 and US9523107B2) disclose immobilized engineered transaminases that retain activity in organic solvents and exhibit improved stability, respectively. These advancements in enzyme immobilization techniques contribute to the development of efficient and reusable biocatalysts for industrial-scale chiral amine production.
In another study, covalent immobilization of ω-transaminase from Vibrio fluvialis JS17 on chitosan beads has been reported to be an effective method for enhancing the stability and reusability of the enzyme.Citation43 Chitosan beads, prepared by the emulsion method, were used as support for the immobilization process. The study reported a higher yield (54.3%) and residual activity (17.8%) of the immobilized enzyme on chitosan beads compared to other commercial supports. The optimal pH for the immobilized enzyme was found to be pH 9.0, similar to that of the free enzyme. The immobilized ω-transaminase on chitosan beads retained ca. 77% of its conversion after five consecutive reactions with the 25 mM substrate, demonstrating better stability and reusability compared to Eupergit® C. Additionally, the immobilized enzyme retained 70% of its initial activity after storage at 4°C for 3.5 weeks, further highlighting the advantages of using chitosan beads for enzyme immobilization. Gabor Koplanyi et al studied the simultaneous purification and immobilization of recombinant transaminase using an advanced nano-composite system.Citation44 The Chromobacterium violaceum transaminase was expressed in E. coli cells and affinity purified using silica nanoparticles with metal affinity linkers and within polylactic acid nanofibers. This method enhanced the efficiency of enzyme isolation and purification compared to classical methods. The study reported by Heinks et alCitation45 compared four immobilization strategies for four different transaminases from diverse species: covalent binding via a unique formylglycine residue per subunit to amine beads, multipoint attachment to glutaraldehyde-functionalized amine or epoxy beads, and cross-linking to form enzyme aggregates (CLEAs). Immobilization effects were highly heterogeneous - optimal immobilization depended on the specific transaminase and application. Multipoint binding to glutaraldehyde-amine beads provided highest activities up to 62 U/g beads for dimeric ATA-Vfl, ATA-3FCR-5M and tetrameric ATA-Bmu. However, site-selective immobilization via the aldehyde tag to amine beads enhanced stability and recyclability of hexameric ATA-Lsy. All immobilization techniques improved operational stability compared to soluble enzymes, enabling reusability over at least 10 cycles while maintaining full or high activity. Scaled-up kinetic resolutions using optimized immobilizates achieved full conversions with retained enantioselectivity. This systematic study highlights the transaminase-specific impacts of immobilization strategies on stability and catalytic properties.
Menegatti developed a microbioreactor using a porous hydrogel to immobilize a selected amine transaminase and its cofactor pyridoxal phosphate (PLP).Citation46 Immobilization efficiency exceeded 97%. After 10 days of continuous operation, the reactor retained 92% of its initial productivity without any observable leaching of the enzyme or cofactor. Co-immobilization of the enzyme and cofactor showed similar performance with or without additional PLP, suggesting exogenous PLP was unnecessary. The reactor achieved high space-time yield of 19.91 g L−1 h−1 and enzyme productivity of 5.4 mgenzyme−1 h−1. Immobilization also improved the enzyme’s stability over a wider pH and temperature range compared to the free enzyme. Given the efficient, time- and cost-effective immobilization and potential for capacity expansion, this microbioreactor shows great promise for industrial application.
In summary, enzyme immobilization techniques improve stability, enable reuse, and facilitate product recovery in ω-transaminase-catalyzed reactions. Recent studies have demonstrated the benefits of immobilization on various solid supports, such as agarose, cross-linked polystyrene, and chitosan. Advanced immobilization strategies, such as transaminase-PLP co-immobilization, hold promise in addressing industrial bioprocess limitations.
Cofactor Dependence and Regeneration
Since biocatalysts like transaminases rely on costly cofactors, developing efficient cofactor recycling methods is imperative for viability.Citation47 Enzymatic regeneration systems outperform direct supplementation and chemical reduction by enabling turnover through multiple reaction cycles. Popular cofactor recycling enzymes include glucose dehydrogenase (GDH), formate dehydrogenase (FDH), and phosphite dehydrogenase (PDH).
For example, Walton et al employed an engineered PDH to recycle NADPH during transamination. Using glucose as the sacrificial substrate, just 1.5 mol% NADP+ was required relative to amine product. The cofactor recycling system enabled gram-scale transaminase-catalyzed synthesis of the antilipidemic drug fenofibrate. Process modeling suggested enzymatic cofactor recycling could reduce manufacturing costs up to 70% compared to chemical reduction.Citation48
An alternative regeneration strategy involves coupling two biocatalytic reactions in a self-sufficient manner, eliminating cofactor supplementation entirely.Citation49,Citation50 Kroutil et al reported the first successful amination of primary alcohols via a self-supporting redox biocatalytic network, though the end products were primary amines.Citation51 The first step of this artificial multienzyme cascade oxidized primary alcohols using an NAD+-dependent alcohol dehydrogenase from Rhodococcus ruber DSM 4454 (ADH-RR), generating the aldehyde and NADH. Next, ATA-CV, ATA-VF, or ATA-BM aminated the aldehyde intermediate. L-alanine was the preferred amine donor due to in situ recycling of pyruvate. LDH maintained cofactor regeneration by consuming NADH. The advantage of this artificial metabolic pathway is its self-sufficiency and recycling capacity, enabling a redox-neutral system without requiring external hydride sources like glucose or formate. Consequently, Kroutil et al designed a green, selective one-pot amination of alcohols via this biocatalytic network. A patent from Codexis (US11193157B2) use of an amino acid dehydrogenase in combination with a cofactor regenerating system comprising a ketoreductase, highlighting the importance of cofactor regeneration in these processes.
Process Development Guided by Technoeconomics
While protein engineering aims to enhance enzyme efficiency, economic feasibility assessments are equally crucial to establish viable processes. Detailed process modeling provides key insights into cost drivers, constraints, and sensitivities. These learnings guide targeted development to ensure commercial viability. Patents from Lek Pharmaceuticals (EP2935205B1 and US9388188B2) focus on the use of penicillin amidase and hydrolytic enzymes for the synthesis of sitagliptin intermediates, demonstrating the significance of process development in the enzymatic production of chiral amines.
Huo et al constructed process simulations for two multi-enzyme routes to chiral amines: transaminase vs amine dehydrogenase.Citation52 The analysis suggested enzyme cost accounted for >90% of raw materials costs in both processes. Thus, enzyme efficiency improvements would significantly reduce overall production costs. With 4–5 fold increased activity, the dehydrogenase route became cost competitive, motivating further enzyme engineering.
Characterization of immobilized enzymes enables evaluating the immobilization process itself and projecting the performance of the immobilized enzyme under process conditions. Such characterization guides selecting strategies for support functionalization and enzyme immobilization onto the activated support. It determines optimal conditions for conducting immobilization based on the intended biocatalyst application, linking biocatalyst production to use. Quantifying the catalytic potential of the immobilized enzyme under operational conditions provides invaluable insight by accounting for both activity and stability. This includes effects of mass transfer limitations (diffusional restrictions) and intrinsic enzyme inactivation from immobilization. Overall, comprehensive characterization of immobilized enzymes informs immobilization strategies and projects real-world performance, optimizing the connection between immobilized biocatalyst preparation and implementation.Citation53
Achieving economic viability with transaminase biocatalysis for industrial chiral amine production requires optimizing various process parameters. Efficient cofactor recycling systems are crucial to reduce costs associated with the expensive pyridoxal 5’-phosphate (PLP) cofactor. Strategies include coupling transaminase reactions with alanine dehydrogenase to regenerate PLP from pyruvate, or using enzyme cascades with amine dehydrogenases or glucose dehydrogenase for cofactor recycling. Immobilizing the transaminase and cofactor onto solid supports enhances stability and reuse, lowering enzyme costs. Process modeling indicates that high substrate concentrations (>100 g/L) and minimal byproduct formation are needed to obtain suitable product titers and yields at commercial scale. Furthermore, techno-economic analyses demonstrate the enzyme contribution should be <10% of overall production costs. Therefore, protein engineering to improve transaminase activity and stability is recommended to decrease required enzyme loading. In summary, realizing the industrial potential of transaminase biocatalysis requires integrated process development addressing cofactor recycling, enzyme immobilization, and reaction optimization guided by techno-economic targets.
Environmental Sustainability of Chiral Amine Synthesis
The pharmaceutical industry faces growing pressure to develop more sustainable manufacturing processes with lower environmental impacts. A key metric is the E factor, which measures waste generated per kilogram of product. In the late 1990s, Roger Sheldon highlighted the significantly higher E factors for pharmaceuticals (25–100) compared to other chemical industries.Citation54,Citation55 This waste contributes to the industry’s negative environmental footprint. A major pharmaceutical synthesis route involves chiral amine production using heavy metals and reducing agents, severely threatening ecosystems.
In response, green chemistry techniques like biocatalysis are gaining traction. Biocatalysis utilizes engineered enzymes to impart high chemo-, regio- and stereoselectivity under mild, aqueous conditions. While native enzymes have limited scope, protein engineering over the past decade has expanded the toolbox. Key engineered enzymes include monoamine oxidases, imine reductases, amine dehydrogenases and transaminases (). However, realizing biocatalysis’ full potential requires addressing hurdles like limited substrate scope, low catalytic efficiency and problematic equilibria ().
Table 1 Selective Examples of Biocatalysts in Synthesizing Chiral Amine-Containing Pharmaceuticals and Their Therapeutic Importance
Table 2 Represents Different Enzymes and Reaction Parameters Used in Biocatalysis
Insights from Patent Literature
The patent literature reveals significant innovation in developing biocatalytic routes for efficient and sustainable production of the important antidiabetic drug sitagliptin. Transaminase enzymes have emerged as promising biocatalysts due to their high stereoselectivity. Several patents describe engineering Arthrobacter and other transaminases to expand substrate scope and enhance catalysis of bulky chiral amine intermediates like sitagliptin. Other patents disclose immobilization techniques to improve transaminase stability and reuse, while mutations target enhanced performance under industrial conditions. Beyond transaminases, diverse enzymes including amidases, reductases, and hydrolases have been applied for specific sitagliptin intermediate steps. Overall, the patents exemplify a shift from traditional chemical synthesis to more efficient biocatalytic platforms. Key innovations involve re-engineering natural enzymes for non-native substrates; integrating computational modeling, directed evolution, and high-throughput screening to rapidly optimize biocatalysts; and immobilization/process engineering to realize advantages at manufacturing scale (). As growing diabetes prevalence increases demand, these patents suggest biocatalysis can offer green, economically viable production of essential medicines like sitagliptin. Realizing this potential requires continued enzyme and process engineering guided by techno-economic assessments. The patent literature provides a promising outlook for establishing enzymatic synthesis as a scalable and sustainable manufacturing platform for pharmaceuticals.
Table 3 Overview of Sitagliptin Biocatalysis Patents
Conclusions
The enzymatic synthesis of chiral amines has made remarkable progress in recent years, driven by advances in protein engineering and process optimization. By re-engineering key enzymes like transaminases, amine oxidases, and amine dehydrogenases, researchers have significantly expanded the substrate scope and enhanced the catalytic efficiency of these biocatalysts. Directed evolution, rational design, and high-throughput screening have been instrumental in creating enzyme variants capable of selectively producing complex chiral amine pharmaceuticals, such as sitagliptin, saxagliptin, and abrocitinib. Moreover, immobilization techniques have improved enzyme stability and reusability, while cofactor recycling strategies have reduced process costs.
However, realizing the full potential of enzymatic chiral amine synthesis at an industrial scale requires further optimization guided by techno-economic assessments. Process modeling and detailed cost analyses are crucial for identifying key cost drivers, constraints, and sensitivities. These insights can guide targeted enzyme and process development to ensure commercial viability. Additionally, integrating state-of-the-art protein engineering with advanced process intensification strategies, such as cascade reactions and in situ product removal, will be essential for achieving economically competitive and sustainable biomanufacturing.
The patent literature highlights the growing interest in biocatalytic routes for chiral amine synthesis, particularly for high-value pharmaceuticals like sitagliptin. Numerous patents disclose engineered transaminases, amine dehydrogenases, and other enzymes with improved activity, stability, and stereoselectivity. These innovations, combined with immobilization techniques and process optimization, demonstrate the potential for enzymatic synthesis to replace traditional chemical methods.
In conclusion, recent advances in enzyme engineering and process development have positioned biocatalysis as a promising alternative to chemical synthesis for the production of chiral amines. By combining cutting-edge protein engineering with integrated process optimization, guided by techno-economic considerations, the pharmaceutical industry can harness the power of enzymes to establish scalable, sustainable, and economically viable routes to these essential building blocks. The future of chiral amine synthesis lies in the synergy between biocatalysis and process engineering, driving the development of green and efficient manufacturing processes.
Disclosure
The authors report no conflicts of interest in this work.
Acknowledgments
The discussion above reflects solely the personal views and considerations of authors and does not constitute either legal advice or the views of Pergament & Cepeda LLP. Thus, neither the authors nor Pergament & Cepeda can be bound either philosophically or as representatives of their various past, present and future clients to the comments and views expressed herein. Although every attempt has been made to ensure that the statements herein are accurate, errors or omissions may be present for which we disclaim any liability.
Additional information
Funding
References
- Höhne M, Bornscheuer UT. Biocatalytic routes to optically active amines. ChemCatChem. 2009;1(1):42–51. doi:10.1002/cctc.200900110
- Desai AA. Sitagliptin manufacture: a compelling tale of green chemistry, process intensification, and industrial asymmetric catalysis. Angew Chem Int Ed Engl. 2011;50(9):1974–1976. doi:10.1002/anie.201007051
- Fuchs M, Koszelewski D, Tauber K, Kroutil W, Faber K. Chemoenzymatic asymmetric total synthesis of (S)-Rivastigmine using ω-transaminases. Chem. Commun. 2010;46(30):5500–5502. doi:10.1039/c0cc00585a
- Bedell TA, Hone GAB, Bois JD, Sorensen EJ. An expedient synthesis of maraviroc (UK-427,857) via C-H functionalization. Tetrahedron Lett. 2015;56(23):3620–3623. doi:10.1016/j.tetlet.2015.01.074
- Lakhani P, Modi CK. Shaping enantiochemistry: recent advances in enantioselective reactions via heterogeneous chiral catalysis. Mol Catal. 2023;548:113429. doi:10.1016/j.mcat.2023.113429
- Trowbridge A, Walton SM, Gaunt MJ. New strategies for the transition-metal catalyzed synthesis of aliphatic amines. Chem Rev. 2020;120(5):2613–2692. doi:10.1021/acs.chemrev.9b00462
- Economidou M, Mistry N, Wheelhouse KMP, Lindsay DM. Palladium extraction following metal-catalyzed reactions: recent advances and applications in the pharmaceutical industry. Org Process Res Dev. 2023;27(9):1585–1615. doi:10.1021/acs.oprd.3c00210
- Bell EL, Finnigan W, France SP, et al. Biocatalysis. Nat Rev Method Primers. 2021;1:46.
- Wang H, Lin Q, Liu M, et al. Molecular docking and site-directed mutagenesis of GH49 family dextranase for the preparation of high-degree polymerization isomaltooligosaccharide. Biomolecules. 2023;13(2):300. doi:10.3390/biom13020300
- Pullmann P, Ulpinnis C, Marillonnet S, Gruetzner R, Neumann S, Weissenborn MJ. Golden Mutagenesis: an efficient multi-site-saturation mutagenesis approach by Golden Gate cloning with automated primer design. Sci Rep. 2019;9(1):10932. doi:10.1038/s41598-019-47376-1
- Friesner RA, Banks JL, Murphy RB, et al. Glide: a new approach for rapid, accurate docking and scoring. 1. Method and assessment of docking accuracy. J Med Chem. 2004;47(7):1739–1749. doi:10.1021/jm0306430
- Mariano DCB, Santos LH, Machado KDS, Werhli AV, De lima LHF, de Melo-Minardi RC. A computational method to propose mutations in enzymes based on Structural Signature Variation (SSV). Int J Mol Sci. 2019;20(2):333. doi:10.3390/ijms20020333
- Ubhayasekera W. Homology Modeling for Enzyme Design. Methods Mol Biol. 2018;1796:301–320.
- Fondeur-Gelinotte M, Lattard V, Oriol R, et al. Phylogenetic and mutational analyses reveal key residues for UDP-glucuronic acid binding and activity of beta1,3-glucuronosyltransferase I (GlcAT-I). Protein Sci. 2006;15(7):1667–1678. doi:10.1110/ps.062089106
- Casadevall G, Duran C, Osuna S. AlphaFold2 and deep learning for elucidating enzyme conformational flexibility and its application for design. JACS Au. 2023;3(6):1554–1562. doi:10.1021/jacsau.3c00188
- Wahart AJC, Staniland J, Miller GJ, Cosgrove SC. Oxidase enzymes as sustainable oxidation catalysts. Royal Soc Open Sci. 2022;9(1):211572. doi:10.1098/rsos.211572
- Ghislieri D, Green AP, Pontini M, et al. Engineering an enantioselective amine oxidase for the synthesis of pharmaceutical building blocks and alkaloid natural products. J Am Chem Soc. 2013;135(29):10863–10869. doi:10.1021/ja4051235
- Alexeeva M, Enright A, Dawson MJ, Mahmoudian M, Turner NJ. Deracemization of alpha-methylbenzylamine using an enzyme obtained by in vitro evolution. Angew Chem Int Ed Engl. 2002;41(17):3177–3180. doi:10.1002/1521-3773(20020902)41:17<3177::AID-ANIE3177>3.0.CO;2-P
- Carr R, Alexeeva M, Dawson MJ, Gotor-Fernandez V, Humphrey CE, Turner NJ. Directed evolution of an amine oxidase for the preparative deracemisation of cyclic secondary amines. Chembiochem. 2005;6(4):637–639. doi:10.1002/cbic.200400329
- Dunsmore CJ, Carr R, Fleming T, Turner NJ. A chemo-enzymatic route to enantiomerically pure cyclic tertiary amines. J Am Chem Soc. 2006;128(7):2224–2225. doi:10.1021/ja058536d
- Kelly SA, Pohle S, Wharry S, et al. Application of omega-transaminases in the pharmaceutical industry. Chem Rev. 2018;118(1):349–367. doi:10.1021/acs.chemrev.7b00437
- Adams JP, Brown MJB, Diaz-Rodriguez A, Lloyd RC, Roiban G-D. Biocatalysis: a pharma perspective. ASC. 2019;361:2421–2432.
- Iwasaki A, Yamada Y, Kizaki N, Ikenaka Y, Hasegawa J. Microbial synthesis of chiral amines by (R)-specific transamination with Arthrobacter sp. KNK168. Appl Microbiol Biotechnol. 2006;69(5):499–505. doi:10.1007/s00253-005-0002-1
- Savile CK, Janey JM, Mundorff EC, et al. Biocatalytic asymmetric synthesis of chiral amines from ketones applied to sitagliptin manufacture. Science. 2010;329(5989):305–309. doi:10.1126/science.1188934
- Midelfort KS, Kumar R, Han S, et al. Redesigning and characterizing the substrate specificity and activity of Vibrio fluvialis aminotransferase for the synthesis of imagabalin. Protein Eng Des Sel. 2013;26(1):25–33. doi:10.1093/protein/gzs065
- Gomm A, Lewis W, Green AP, O’Reilly E. A new generation of smart amine donors for transaminase-mediated biotransformations. Chemistry. 2016;22(36):12692–12695. doi:10.1002/chem.201603188
- Musa MM, Hollmann F, Mutti FG. Synthesis of enantiomerically pure alcohols and amines via biocatalytic deracemisation methods. Catal Sci Technol. 2019;9(20):5487–5503. doi:10.1039/C9CY01539F
- Gilio AK, Thorpe TW, Turner N, Grogan G. Reductive aminations by imine reductases: from milligrams to tons. Chem Sci. 2022;13(17):4697–4713. doi:10.1039/D2SC00124A
- Knaus T, Bohmer W, Mutti FG. Amine dehydrogenases: efficient biocatalysts for the reductive amination of carbonyl compounds. Green Chem. 2017;19(2):453–463. doi:10.1039/C6GC01987K
- Bommarius BR, Schurmann M, Bommarius AS. A novel chimeric amine dehydrogenase shows altered substrate specificity compared to its parent enzymes. Chem Commun. 2014;50(95):14953–14955. doi:10.1039/C4CC06527A
- Liu J, Kong W, Bai J, et al. Amine dehydrogenases: current status and potential value for chiral amine synthesis. ChemCatalysis. 2022;2:1288–1314.
- Patil MD, Grogan G, Bommarius A, Yun H. Oxidoreductase-catalyzed synthesis of chiral amines. ACS Catal. 2018;8(12):10985–11015. doi:10.1021/acscatal.8b02924
- Abrahamson MJ, Wong JW, Bommarius AS. The evolution of an amine dehydrogenase biocatalyst for the asymmetric production of chiral amines. ASC. 2013;355:1780–1786.
- Vanhooke JL, Thoden JB, Brunhuber NM, Blanchard JS, Holden HM. Phenylalanine dehydrogenase from Rhodococcus sp. M4: high-resolution X-ray analyses of inhibitory ternary complexes reveal key features in the oxidative deamination mechanism. Biochemistry. 1999;38(8):2326–2339. doi:10.1021/bi982244q
- Liu J, Pang BQW, Adams JP, Snajdrova R, Li Z. Coupled immobilized amine dehydrogenase and glucose dehydrogenase for asymmetric synthesis of amines by reductive amination with cofactor recycling. ChemCatChem. 2016;9(3):425–431. doi:10.1002/cctc.201601446
- Pushpanath A, Siirola E, Bornadel A, Woodlock D, Schell U. Understanding and overcoming the limitations of bacillus badius and caldalkalibacillus thermarum amine dehydrogenases for biocatalytic reductive amination. ACS Catal. 2017;7(5):3204–3209. doi:10.1021/acscatal.7b00516
- Dave DJ. Saxagliptin: a dipeptidyl peptidase IV inhibitor in the treatment of type II diabetes mellitus. J Pharmacol Pharmacother. 2011;2(4):230–235. doi:10.4103/0976-500X.85934
- Hanson RL, Goldberg SL, Brzozowski DB, et al. Preparation of an amino acid intermediate for the dipeptidyl peptidase iv inhibitor, saxagliptin, using a modified phenylalanine dehydrogenase. ASC. 2007;349:1369–1378.
- Khanam W, Dubey NC. Recent advances in immobilized ω-transaminases for chiral amine synthesis. Mater Today Chem. 2022;24:100922.
- Breuer M, Ditrich K, Habicher T, et al. Industrial methods for the production of optically active intermediates. Angew Chem Int Ed Engl. 2004;43(7):788–824. doi:10.1002/anie.200300599
- Du Y, Dong W, Jiang J, et al. 种来源于Burkholderia phytofirmans PsJN的ω-转氨酶的表达纯化及性质分析 [Expression and characterization of a novel omega-transaminase from Burkholderia phytofirmans PsJN]. Sheng Wu Gong Cheng Xue Bao. 2016;32(7):912–926. Chinese. doi:10.13345/j.cjb.150456
- Agrawal YK, Bhatt HG, Raval HG, Oza PM, Gogoi PJ. Chirality--a new era of therapeutics. Mini Rev Med Chem. 2007;7(5):451–460. doi:10.2174/138955707780619617
- Yi -S-S, Lee C-W, Kim J, Kyung D, Kim B-G, Lee Y-S. Covalent immobilization of ω-transaminase from Vibrio fluvialis JS17 on chitosan beads. Process Biochem. 2007;42(5):895–898. doi:10.1016/j.procbio.2007.01.008
- Koplanyi G, Bell E, Molnar Z, et al. Novel approach for the isolation and immobilization of a recombinant transaminase: applying an advanced nanocomposite system. Chembiochem. 2023;24(7):e202200713. doi:10.1002/cbic.202200713
- Heinks T, Montua N, Teune M, et al. Comparison of four immobilization methods for different transaminases. Catalysts. 2023;13(2):300. doi:10.3390/catal13020300
- Menegatti T, Znidarsic-Plazl P. Hydrogel-based enzyme and cofactor co-immobilization for efficient continuous transamination in a microbioreactor. Front Bioeng Biotechnol. 2021;9:752064. doi:10.3389/fbioe.2021.752064
- Ghislieri D, Turner NJ. Biocatalytic approaches to the synthesis of enantiomerically pure chiral amines. Topics Catal. 2014;57(5):284–300. doi:10.1007/s11244-013-0184-1
- Pollard DJ, Woodley JM. Biocatalysis for pharmaceutical intermediates: the future is now. Trends Biotechnol. 2007;25(2):66–73. doi:10.1016/j.tibtech.2006.12.005
- Abrahamson MJ, Vazquez-Figueroa E, Woodall NB, Moore JC, Bommarius AS. Development of an amine dehydrogenase for synthesis of chiral amines. Angew Chem Int Ed Engl. 2012;51(16):3969–3972. doi:10.1002/anie.201107813
- D. Patil M, Grogan G, Bommarius A, Yun H. Recent advances in ω-transaminase-mediated biocatalysis for the enantioselective synthesis of chiral amines. Catalysts. 2018;8(7):254. doi:10.3390/catal8070254
- Sattler JH, Fuchs M, Tauber K, et al. Redox self-sufficient biocatalyst network for the amination of primary alcohols. Angew Chem Int Ed Engl. 2012;51(36):9156–9159. doi:10.1002/anie.201204683
- Huo H, Yao G, Wang S. Economy assessment for the chiral amine production with comparison of reductive amination and transamination routes by multi-enzyme system. Catalysts. 2020;10(12):1451. doi:10.3390/catal10121451
- Illanes A, Wilson L. Parameters for the evaluation of immobilized enzymes under process conditions. Methods Mol Biol. 2020;2100:65–81.
- Sheldon RA. Selective catalytic synthesis of fine chemicals: opportunities and trends. J Mol Catal. 1996;107(1–3):75–83. doi:10.1016/1381-1169(95)00229-4
- Sheldon RA. Catalysis: the key to waste minimization. J Chem Technol Biotechnol. 1997;68(4):381–388. doi:10.1002/(SICI)1097-4660(199704)68:4<381::AID-JCTB620>3.0.CO;2-3
- Li T, Liang J, Ambrogelly A, et al. Efficient, chemoenzymatic process for manufacture of the Boceprevir bicyclic [3.1.0]proline intermediate based on amine oxidase-catalyzed desymmetrization. J Am Chem Soc. 2012;134(14):6467–6472. doi:10.1021/ja3010495
- Njoroge FG, Chen KX, Shih NY, Piwinski JJ. Challenges in modern drug discovery: a case study of boceprevir, an HCV protease inhibitor for the treatment of hepatitis C virus infection. Acc Chem Res. 2008;41(1):50–59. doi:10.1021/ar700109k
- Kumar R, Karmilowicz MJ, Burke D, et al. Biocatalytic reductive amination from discovery to commercial manufacturing applied to abrocitinib JAK1 inhibitor. Nat Catal. 2021;4(9):775–782. doi:10.1038/s41929-021-00671-5
- Duan J, Li B, Qin Y, Dong Y, Ren J, Li G. Recent progress in directed evolution of stereoselective monoamine oxidases. Bioresources Bioprocess. 2019;6(1):37. doi:10.1186/s40643-019-0272-6
- Green AP, Turner NJ. Biocatalytic retrosynthesis: redesigning synthetic routes to high-value chemicals. Perspect Sci. 2016;9:42–48. doi:10.1016/j.pisc.2016.04.106
- Herter S, Medina F, Wagschal S, Benhaim C, Leipold F, Turner NJ. Mapping the substrate scope of monoamine oxidase (MAO-N) as a synthetic tool for the enantioselective synthesis of chiral amines. Bioorg Med Chem. 2018;26(7):1338–1346. doi:10.1016/j.bmc.2017.07.023
- Guo F, Berglund P. Transaminase biocatalysis: optimization and application. Green Chem. 2017;19(2):333–360. doi:10.1039/C6GC02328B
- Matzel P, Gand M, Höhne M. One-step asymmetric synthesis of (R)- and (S)-rasagiline by reductive amination applying imine reductases. Green Chem. 2017;19(2):385–389. doi:10.1039/C6GC03023H
- Zhang J, Ma Y, Zhu F, et al. Structure-guided semi-rational design of an imine reductase for enantio-complementary synthesis of pyrrolidinamine. Chem Sci. 2023;14(16):4265–4272. doi:10.1039/D2SC07014F