Abstract
Recent advances in nanotechnology and biotechnology have contributed to the development of engineered nanoscale materials as innovative prototypes to be used for biomedical applications and optimized therapy. Due to their unique features, including a large surface area, structural properties, and a long circulation time in blood compared with small molecules, a plethora of nanomaterials has been developed, with the potential to revolutionize the diagnosis and treatment of several diseases, in particular by improving the sensitivity and recognition ability of imaging contrast agents and by selectively directing bioactive agents to biological targets. Focusing on cancer, promising nanoprototypes have been designed to overcome the lack of specificity of conventional chemotherapeutic agents, as well as for early detection of precancerous and malignant lesions. However, several obstacles, including difficulty in achieving the optimal combination of physicochemical parameters for tumor targeting, evading particle clearance mechanisms, and controlling drug release, prevent the translation of nanomedicines into therapy. In spite of this, recent efforts have been focused on developing functionalized nanoparticles for delivery of therapeutic agents to specific molecular targets overexpressed on different cancer cells. In particular, the combination of targeted and controlled-release polymer nanotechnologies has resulted in a new programmable nanotherapeutic formulation of docetaxel, namely BIND-014, which recently entered Phase II clinical testing for patients with solid tumors. BIND-014 has been developed to overcome the limitations facing delivery of nanoparticles to many neoplasms, and represents a validated example of targeted nanosystems with the optimal biophysicochemical properties needed for successful tumor eradication.
Introduction
The application of nanotechnology in medicine is providing significant opportunities and new perspectives for novel and effective treatments in many disorders, with great potential in health care. Nanomedicine can be defined as the design and development of therapeutics and/or diagnostic agents in the nanoscale range (with diameters ranging from 1 nm to 1,000 nm), with the possibility, by moving within biological systems, to transport and deliver a variety of biomedical entities for the treatment, prevention, and diagnosis of many diseases ().Citation1–Citation5
Biological transport processes, from external barriers (skin and mucosa), en route compartments (blood and extracellular matrix), and cellular membranes, to destinations at the cellular and subcellular levels are affected by the physical features of nanocarriers, including their size, shape, surface charge, and intrinsic chemical properties, as well as the incorporation of active ligands for recognition of biological receptors.Citation6–Citation10 Due to their unique characteristics, including large surface area, structural properties, and long circulation time in blood compared with small molecules, nanoparticles have emerged as attractive candidates for optimized therapy through personalized medicine.Citation11,Citation12 Potential advantages of engineered therapeutic nanoparticles are the ability to: revert unfavorable physicochemical properties of bioactive molecules to desirable biopharmacologic profiles; improve delivery of therapeutics across biological barriers and compartments; control release of bioactive agents; enhance therapeutic efficacy by selective delivery of therapeutics to biological targets; and perform theranostic functions by combining multimodal imaging and simultaneous diagnosis and therapy into multifunctional nanoplatforms.Citation13–Citation18
Over the last few decades, many original tools have been developed based on various components from metals to proteins, including carbon, silica oxides, metal oxides, nanocrystals, lipids, polymers, dendrimers, and quantum dots, as well as newly developed materials.Citation1,Citation2,Citation4,Citation9,Citation19–Citation23 For example, carbon nanomaterials with a carbon cage (eg, fullerenes, nanodiamonds) and graphene structures (eg, carbon nanotubes, nanohorns) have been explored as carriers for drug delivery and other biomedical applications,Citation24–Citation27 due to their high variability, chemical stability, and unique characteristics, such as highly tailorable surface chemistry and high carrier capacity, and the feasibility of incorporating a variety of molecules as anticancer therapeutics. However, to enable drug delivery platforms, the major emphasis should lie in investigation of the mechanism, thermodynamics, and kinetics of adsorption/desorption equilibria for putative drugs on/from carbon nanomaterials with differing purity, surface chemistry, and agglomeration state under different conditions. Moreover, a detailed understanding of the pharmacologic and toxicologic properties of these nanomaterials, and a balanced and detailed evaluation of their risks and benefits to human health, are expected before their translation into clinical use. New perspectives using innovative nanomaterials for cancer treatment have been offered by a multifunctional platform based on gold nanoparticles, with the possibility of combining imaging and therapy, and also implementing multiple receptor targeting.Citation28–Citation32 Gold nanospheres, nanorods, nanoshells, and nanocages, for example, are currently being investigated for in vivo imaging, cancer therapy, and drug delivery. In particular, most studies of gold nanoparticle-based cancer therapy have involved a photothermal approach by near-infrared region laser exposure for the destruction of cancer cells or tumor tissue, which might have great potential in the clinical setting.Citation29–Citation31 Thus, laser-exposed gold nanoparticles could act as therapeutic agents by themselves without the need for co-conjugated drugs. In addition, gold nanoparticles have been used in exploratory drug delivery applications by: partitioning and diffusion-driven release of hydrophobic drug molecules; loading therapeutics by surface complexation; anchoring drugs directly to particle surfaces; loading by layer-by-layer assembly; and loading drugs inside nanoparticles.Citation32
As far as drug delivery is concerned, the most important nanoparticle platforms are liposomes, polymer conjugates, polymeric micelles, dendrimers, nanoshells, and protein and nucleic acid-based nanoparticles.Citation1–Citation3,Citation6,Citation7 Among these, the two prominent types of nanoparticles, ie, liposomes and polymer-based nanoformulations, constitute the majority of the nanoparticle therapeutics available for clinical use ().Citation7,Citation10,Citation15,Citation33–Citation40
Figure 2 Representative nanocarriers for drug delivery, ie, liposomes (left) and polymeric nanoparticles (right). Liposomes are self-assembling vesicles with a bilayered membrane structure containing amphiphilic molecules (phospholipids) and hydrophobic and hydrophilic groups that self-assemble in water. Polymeric nanoparticles are biocompatible and biodegradable polymeric nanoformulations in which drugs are dissolved, entrapped, or conjugated to the surface of the nanoparticles.
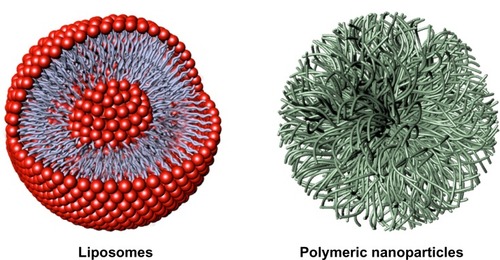
Although these materials are likely to provide a high degree of biocompatibility, their utilization in biomedicine requires controlled interactions with biomacromolecules. To translate developed nanomedicines into clinical practice successfully, several issues, including a favorable blood half-life and physiologic behavior with minimal off-target effects, effective clearance from the human organism, and minimal or no toxicity to healthy tissues in living organisms, should be taken into consideration.Citation10,Citation41 More specifically, hydrodynamic diameter, surface charge, and hydrophobic/hydrophilic balance are important physicochemical properties that can affect the in vivo biodistribution and clearance of administered nanoparticles.Citation10,Citation42 For example, optimal nanoparticle surface modification using appropriate biocompatible and biodegradable polymers, such as polyethylene glycol (PEG), constitutes a suitable strategy to reduce opsonization and uptake processes in the reticuloendothelial system, also facilitating efficient clearance of nanoparticles and/or their metabolites from the body.Citation36,Citation43–Citation47 Therefore, to achieve a favorable blood half-life and biodistribution with minimal recognition and elimination by the reticuloendothelial system, great effort should be devoted to optimizing the physicochemical profile of nanoparticles.
This review highlights recent advances in the development of targeted controlled-release nanoparticles for antitumor chemotherapy, starting from the potential impact of nanotechnology in medicine, through to the clinical development of emerging passive and active targeting nanoparticles for effective controlled drug delivery. Moreover, the evolution of BIND-014, the first clinically tested targeted nanomedicine for management of cancer, as well as its pharmacobiologic properties and potential within the chemotherapeutic arena, are herein detailed.
Emerging and current nanomedicines and targeting methods: focus on cancer
The incidence of cancer has been increasing in recent decades, and eradication of the major types of the disease remains an elusive clinical goal, largely due to the heterogeneous and idiosyncratic nature of individual cancers, and the inability to target therapeutics to neoplastic areas without damaging normal tissues.Citation48,Citation49 Most of the antitumor agents currently administered by validated therapeutic protocols are systemically distributed without preferential localization to cancer tissue. This widespread biodistribution of chemotherapeutics results in both anticancer effects and off-target adverse effects. Thus far, a few nanotherapeutic (and diagnostic) materials have been approved by the US Food and Drug Administration (FDA) for clinical use, although more are currently in various stages of preclinical and clinical development. Given that most of these products were not specifically designed to have selectivity toward biological targets, they should be considered as first-generation nanomedicines.Citation1,Citation2,Citation7,Citation8,Citation9,Citation10,Citation40
First generation of clinically approved nanomedicines
Among a wide variety of nanosystems, only a few nanomedicines, such as Doxil® (Janssen Biotech Inc., Horsham, PA, USA), Myocet® (Sopherion Therapeutics Inc., Princeton, NJ, USA), DaunoXome® (Galen US Inc., Souderton, PA, USA), Depocyt® (Pacira Pharmaceuticals Inc., San Diego, CA, USA), Abraxane® (Celgene Corporation, Inc., Berkeley Heights, NJ, USA), Genexol-PM® (Samyang Biopharmaceuticals Corporation, Jongno-gu, Seoul, Korea), and Oncaspar® (Enzon Pharmaceuticals Inc., Bridgewater, NJ, USA), are approved for use in the treatment of cancer ().
Table 1 Examples of nontargeted nanosystems in clinical use for anticancer therapy
Doxil, the first nanomedicine to secure regulatory approval by the FDA (for the treatment of Kaposi’s sarcoma in 1995 and in Europe in 1997 with the brand name Caelyx®), was obtained by encapsulating doxorubicin within liposomes.Citation50,Citation51 This nanoformulation consistently improved the pharmacokinetics and biodistribution profile of doxorubicin, thus facilitating a longer circulation half-life and maximizing drug accumulation in tumor tissue. However, despite clinical validation of this representative prototype of liposome technology in the treatment of metastatic breast cancer, ovarian cancer, and multiple myeloma, this class of nanovehicles generally fails to provide the controlled release and stability needed to control the kinetic profile and drug localization at the target tumor tissue. The non-PEGylated liposomal doxorubicin formulations, Myocet and DaunoXome, have been used for metastatic breast cancer and Kaposi sarcoma, respectively.Citation52,Citation53 Further, DepoCyt, a non-PEGylated liposomal nanocarrier loaded with cytarabine, was approved in 1999 for local intrathecal treatment of lymphomatous meningitis, and has now entered Phase III trials for leukemia and Phase I/II clinical trials for glioblastoma.Citation52
Another therapeutic nanoformulation to secure clinical approval by the FDA (in 2005) is Abraxane, a co-condensate of albumin and paclitaxel.Citation53 In comparison with paclitaxel formulated using Cremophor® EL (the nononic surfactant used in the Taxol® formulation), Abraxane demonstrated significantly higher tumor response rates and longer times to tumor progression in patients with metastatic breast cancer. However, the utility of this technology is mainly limited to improving the therapeutic index of hydrophobic therapeutic agents (able to bind to albumin) used in formulations with poorly tolerated vehicles.
More recently, in 2007 in Korea, another polymeric nanocarrier was approved for marketing, ie, Genexol-PM, a paclitaxel-loaded poly(lactic acid)-block-poly(ethylene glycol) micelle formulation, that was developed to avoid the need to use Cremophor EL.Citation54 Genexol-PM led to an increase in the potency of paclitaxel in the treatment of breast and lung cancer, and is currently in Phase II clinical development in the US and Russia. With regard to PEGylated devices, Oncaspar, a combination of PEG-L-asparaginase, has recently been approved for the treatment of leukemia.Citation52
Liposomes and polymeric drug release nanoformulations in clinical development
Despite the progress made in the clinical development of liposome-based nanocarriers, there are several issues that limit their wider consideration as a main drug delivery platform, including the difficulty in modulating their drug release in vivo, the limited amount of drug that can be loaded, possible oxidation of liposomal phospholipids, and the difficulty in maintaining a stable shelf-life. Otherwise, polymeric nanocarriers demonstrate similar to superior stability in vivo as compared to liposomes, have a high drug-loading capacity, and enable both controlled and triggered release of drugs. Therefore, polymeric-based nanomaterials have the potential to provide solutions for a range of problems in nanomedicine.Citation40,Citation55–Citation58
The way forward for the clinical translation of controlled-release polymeric nanoparticles was paved by the seminal work of Langer and Folkman in 1976, which demonstrated the controlled release of macromolecules from biodegradable polymers in a temporal manner.Citation36 Moreover, a landmark paper by Langer et al in 1994 provided further evidence that diblock copolymers of biocompatible polymers with PEG can dramatically increase the controlled release and circulation half-life of polymeric nanoparticles.Citation37 Because of their biocompatibility, biodegradability, and chemical and technologic flexibility, the polymeric materials most widely used for controlled drug release include PLA, poly(D,L-lactide-co-glycolide) (PLGA), poly(caprolactone) (PCL), poly(glutamic acid), N-(2-hydroxypropyl)-methacrylate copolymers, and poly(amino acids).Citation56
“Drug-targeting” strategies
Since Paul Ehrlich, considered the “father of chemotherapy”, suggested the concept of a “magic bullet”, ie, “a drug that selectively attaches to diseased cells but is not toxic to healthy cells” approximately a century ago,Citation59 a great deal of interest has been channeled in this direction, focused particularly on the treatment of cancer.Citation60 Several strategies can be exploited to reach a putative biological target, and include passive drug targeting, active drug targeting, active drug targeting to endothelial cells, and triggered drug delivery ().Citation6–Citation8,Citation10,Citation12,Citation40,Citation56–Citation58
Figure 3 Strategies adopted for drug targeting and localization of nanosystems to tumor cells and tissues.
Notes: (A) Passive drug targeting. Circulating nanoparticles passively extravasate in solid tumor tissue via the enhanced permeability of blood vessels, ie, through the disorganized and leaky vasculature surrounding the solid tumor coupled with the absence of lymphatic drainage, and preferentially accumulate in tumor cells (the EPR effect). (a) The drug is released into the extracellular matrix and diffuses through the cells and tissue. (B) Active drug delivery. Once nanoparticles passively extravasate and concentrate in the target tissue via the EPR effect, the presence of ligands grafted onto the nanoparticle surface enable active targeting of the nanoparticles to receptors that are overexpressed on tumor cells or tissue, resulting in enhanced uptake and internalization via receptor-mediated endocytosis. (b) Tumor-specific ligands on the nanoparticles bind to cell surface receptors, triggering internalization of the nanoparticles into the cell through endosomes on which, due to an internal acidic pH, the drug is released from the nanoparticles and diffuses into the cytoplasm. (C) Active drug targeting to endothelial cells. Nanoparticles can be targeted to bind to angiogenic endothelial cell surface receptors with the aims of: enhancing drug accumulation in the tumor endothelium, thereby inhibiting growth of blood vessels supplying the tumor rather than inhibiting tumor cells per se (c); and improving delivery of chemotherapeutic agents to tumor cells via the EPR effect with the potential to act synergistically in targeting both the vascular tissue and tumor cells. (D) Triggered drug delivery by stimuli-sensitive nanomedicines. Nanoparticles passively accumulate in the tumor via the EPR effect. After localization at the target site or while circulating in the tumor vasculature (d), the nanoparticles can be activated by external stimuli (eg, hyperthermia, light, magnetic fields, ultrasound) that induce release of the payload drugs. Images adapted from J Control Release, 161(2), Lammers T, Kiessling F, Hennink we, Storm G, Drug targeting to tumors: principles, pitfalls and (pre-) clinical progress, 175–187, Copyright 2012, with permission from Elsevier.Citation10
Abbreviation: EPR, enhanced permeability and retention.
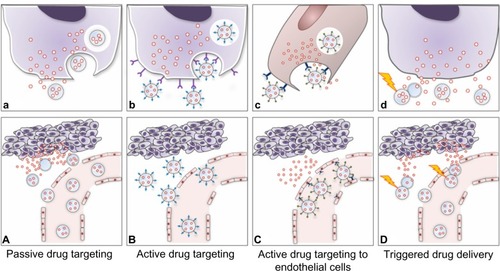
Passive drug targeting
The majority of nanosystems show prolonged blood circulation times in vivo and accumulate at particular sites simply due to the balance between vascular hemodynamic forces and diffusion mechanisms. Passive drug targeting is widely exploited in chemotherapy because nanoparticles circulating in the bloodstream can localized to neoplastic tissues through the well known enhanced permeation and retention effect.Citation61,Citation62 Anatomically, tumor microvasculature is leaky and characterized by abnormal branching and enlarged interendothelial gaps, with associated breakdown of tight junctions between endothelial cells and a disrupted basement membrane.Citation63 These large gaps between endothelial cells facilitate the extravasation of particulate material from the surrounding vessels into the tumor ().Citation64 Therefore, in contrast with the vasculature in healthy tissues, the poorly differentiated vasculature of immature tumors, with pores smaller than about 400 nm, allows for extravasation and selective accumulation of nanoparticles in the tumor interstitium via a passive targeting mechanism ().Citation42,Citation65 Importantly, it should be borne in mind that the enhanced permeation and retention effect is a very heterogeneous phenomenon, varying dramatically from tumor to tumor and from patient to patient.Citation66 For example, even considering a single tumor model, there are many differences with regard to vascular permeability, where particles with a diameter >200–300 nm are able to extravasate, whereas in another part of the same tumor, molecules only a few nanometers in size may have difficulty entering the interstitium.
Most passive targeting nanosystems have a surface coated with PEG for biocompatibility and “stealth” purposes.Citation42–Citation44 Importantly, it should be noted that increased hydrophilicity on the nanoparticle surface can impede its uptake by cancer cells, thereby hampering efficient drug delivery to tumors by passive targeting nanoparticles.Citation42,Citation47,Citation67 However, PEG-based block copolymers have resulted in the clinical translation of numerous passive targeting polymeric nanoparticles, including the abovementioned Genexol-PM, as well as SP1049C, NK911, and other prototypes now entering early clinical trials for the treatment of a variety of cancers ().
SP1049C is a Pluronic polymeric micelle nanoparticle carrying doxorubicin, and is currently undergoing Phase II trials in metastatic cancer of the esophagus refractory to standard chemotherapeutic protocols.Citation68 Another example of a polymeric nanocarrier that acts via a passive targeting mechanism is NK911, a micellar nanoparticle comprising PEG, doxorubicin, and poly(aspartic acid), currently in Phase II clinical development for various types of cancer.Citation69 Moreover, Opaxio™ (formerly Xyotax™), also a passively targeted paclitaxel/poly(L-glutamic acid) nanoconstruct, is proving effective in ovarian tumors,Citation70,Citation71 and CRLX101 (formerly IT-101), a camptothecin-cyclodextrin polymer conjugate, is showing enhanced pharmacokinetic efficacy in both preclinical and clinical studies.Citation72 Further conjugated polymer-drug nanotherapeutics, such as NC-6004 [a cisplatin-incorporated PEG-poly(glutamic acid) block copolymer micellar formulation] and ProLindac™ (a diaminocyclo-hexane-platinum hydroxypropylmethacrylamide prodrug), are in late-stage clinical trials.Citation10 In addition, Paclical®, a micellar formulation of paclitaxel, has recently received orphan drug designation by the FDA, and is currently in Phase III trials for ovarian carcinoma.Citation73 Passive targeting lipid nanocarriers are also moving into the advanced stages of clinical trials, and intensive effort is being made to get these systems into clinical practice (). Promising liposomal nanomedicines in clinical trials include Thermodox®, a thermosensitive liposomal doxorubicin formulation that releases the active drug at temperatures around 39°C, and is currently being tested in Phase III trials together with radiofrequency ablation in patients with hepatocellular carcinoma.Citation74 Finally, SPI-77, a PEGylated liposomal formulation of cisplatin, is in Phase II trials for patients with recurrent epithelial ovarian cancer,Citation75 and a nanoliposomal formulation of irinotecan (CPT-11) is in Phase I trials for glioma.Citation76 Several liposomal formulations carrying two different types of chemotherapeutics, such as cytarabine and daunorubicin, are also reported to be in the process of clinical development.Citation77
Active targeting as a strategy for developing site-directed nanoparticles
Passive targeting strategies have shown several limitations, so a considerable amount of work is now underway investigating active targeting nanoformulations that can maximize their accumulation at sites of interest.Citation7,Citation8,Citation10 In this respect, polymeric nanoparticles allow for versatile modification possibilities, and can act as functional platforms for the assembly of well defined multifunctional structures.Citation40,Citation56–Citation58,Citation78 In fact, slight variations in polymeric composition as well as ligand surface functionalization can facilitate the targeting ability of nanoparticles in biological systems. Active drug targeting involves the use of a variety of affinity ligands to direct the binding of nanoparticles to many biological targets, largely represented by antigens, that are differentially overexpressed both in the plasma membrane and in diseased tissue ().Citation40,Citation79 This approach can be used for controlled drug release applications, where the drug is released into either the extracellular or intracellular compartment. In the latter process, internalization of nanoparticles by receptor-mediated endocytosis can occur through several pathways that lead to endosome formation, and ultimately allow for generation of lysosomes ().Citation56,Citation80 Since the primary role of the targeting ligand is to enhance the uptake of nanoparticles into target cells, cell internalization by active targeting nanoparticles is postulated to improve the therapeutic efficacy as compared with nontargeted nanoparticles.Citation56–Citation58 This suggests that while the biodistribution would be strictly related to the colloidal properties of the nanoparticles, the targeting ligand is important for enhancing both cell recognition and cell uptake at target sites. While the potential benefit of active targeting nanoparticles seems to be widely accepted, this technology has resulted in only a few clinically validated nanoproducts ().Citation10,Citation40,Citation58
Table 2 Tumor-targeted nanomedicines in clinical development
Active drug targeting to endothelial cells and triggered drug delivery
Several important pitfalls in active targeting of drugs to tumor cells have been identified, including: poor tumor penetration by nanomedicines; the complexity of predicting the potential usefulness of an active drug targeting approach for a specific application; difficulty in managing nanoformulations that are too complex in design; the not always favorable implementation of nanomedicine formulations in clinically consistent combination regimens; and the low level of knowledge concerning the biological and (patho)physiologic principles of tumor progression and related treatment.Citation10 Although this approach has potential in controlled and targeted drug delivery, the failure of translation of a great number of targeted nanomedicines at the clinical level can likely be attributed to the inability of targeted nanoparticles to overcome a succession of membrane layers as biological barriers, represented by pericyte-based, smooth muscle cell-based, and fibroblast-based cell layers between endothelial and cancer cells, as well as a plethora of cellular processes and anatomic tumor issues (ie, the high cellular density within solid tumors and high interstitial fluid pressure) that represent important obstacles for reaching the target tumor interstitium.Citation4,Citation6,Citation8–Citation10,Citation56
To address these issues, a variety of vascular-targeted nanoformulations have been designed and evaluated ().Citation8–Citation10,Citation56 Example of ligands targeting angiogenic endothelium are the linear and cyclic derivatives of an oligopeptide containing the Arg-Gly-Asp (RGD) sequence, that binds to target endothelium via integrin receptors.Citation81,Citation82
Another targeting strategy capable of generating innovative nanoformulations focuses on the possibility that a nanosystem can be triggered to release its contents (ie, the payload drug) on exposure to external and confined stimuli, such as light, heat, ultrasound, and magnetic fields, in order to maximize drug release at the pathologic site ().Citation10 The above mentioned product, Thermodox, a temperature-sensitive doxorubicin-PEGylated liposome, is an interesting example of stimuli-responsive nanomedicine.Citation74 Despite the clinical potential of these nanoformulations, there are still several important limitations to their extensive clinical development, that include manufacturing difficulties and problems with managing the way in which they respond to stimuli-responsive drug release. However, much effort has been made to improve the stimuli-responsiveness of these tumor-targeted nanotherapeutics, and the development of more effective nanoparticles for triggered drug delivery is expected in the near future.Citation83,Citation84
Methods and ligands used for targeted drug delivery
In the course of the last decade, several strategies involving conjugation of targeting ligands to the surface of nanoparticles have been developed.Citation38,Citation40,Citation45,Citation55–Citation57 As far as the targeting approach is concerned, one key issue relies on the choice of optimal targeting ligands, possibly by balancing their stoichiometry in comparison with the antibiofouling surface of nanoparticles. More specifically, two important ligand properties, ie, affinity and density, can have a key role in effective targeting of nanoparticles to the cell surface membrane. Again, the ligand binding affinity is the result of the equilibrium between enthalpic advantages (for ligand-receptor interaction) and entropic losses (stretching, flexibility, or compressibility of the nanosystem). For example, greater ligand density does not necessarily lead to a higher intracellular concentration, given the decrease in “stealth” surface characteristics. Moreover, although the uptake of nanoparticles usually increases with an increasing +/− charge ratio of nanoparticles (in terms of zeta potential values), an excess positive charge can induce toxicity and promote an immunologic reaction. Therefore, the optimal ligand density and charge on the nanoparticle surface should be investigated on a case-by-case basis. However, a wide range of chemical modifications is now available to attach targeting ligands to nanoparticles.Citation55–Citation57,Citation85
Conventional methods of preparing targeted nanoparticles involve two typical options, ie, the “postcoupling” and “precoupling” approaches.Citation55–Citation57 The first approach involves bioconjugation of the preformed nanoparticle core with targeting ligands to the nanoparticle surface. This postcoupling approach for attaching affinity ligands does not allow for fine-tuning of the ligand-nanoparticle surface density for optimal efficacy. Conversely, most effective chemical strategies include preconjugation of a targeting ligand after nanoparticle formation followed by nanoformulation (ie, the precoupling method). For example, effective self-assembly of prefunctionalized triblock polymers (ie, copolymer-PEG ligands), accomplished in the pioneering work of Farokhzad and Langer,Citation86,Citation87 demonstrated the possibility of developing more homogenously targeted nanoparticles capable of controlling ligand-to-nanoparticle ratios and preventing a heterogenous distribution of ligands on the surface of nanoparticles. Summarizing this information, it is clear that a combination of several “unpredictable” factors, including the nature of the targeting ligand, the size, shape, and composition of the targeted nanosystem, as well as the balance of the ligand-nanoparticle surface ratio, can synergistically contribute to the efficiency of binding between targeted nanoparticles and their target cells, and directly affect the biological profile in vivo. Therefore, all these aspects should be investigated in a rational fashion when developing a nanosystem.
Using different approaches, nanoparticles can be functionalized using a variety of targeting ligands that include monoclonal antibodies or their fragments, proteins, or peptides, nucleic acid ligands (such as aptamers), and small molecules, the characteristics of which are outlined in the following sections and summarized in .Citation88
Monoclonal antibodies
Monoclonal antibodies have for several years been the preferred class of targeting ligands, and engineered monoclonal antibodies, preferably one capable of evading the immune system, have been widely used in the development of targeted nanoparticles. Current development of monoclonal antibodies has led to chimeric and humanized derivatives to enable modulation of their immunogenicity. The ability of engineered monoclonal antibodies to target and interfere with cellular processes has been demonstrated by the success of several monoclonal antibody therapeutics.Citation89,Citation90 Some of these, including rituximab and trastuzumab, have been conjugated to PLA nanoparticles, resulting in nanoconjugates that show a significant increase in the rate of particle uptake compared with their nontargeted counterparts.Citation91 However, despite the intense effort undertaken for their development, monoclonal antibodies still have a number of limitations, including their large size, requirement for extensive optimization through molecular engineering technologies, difficulty in managing nanoparticle scale-up and manufacturing, and potentially immunogenic characteristics, resulting in rapid nanoparticle clearance. Due to these problems in using monoclonal antibodies, there is increasing interest in engineering and using antibody fragments as targeting molecules to retain the high antigen binding specificity of the parent antibodies, but with less immunogenicity and a smaller size, and thus obtain monoclonal antibodies that are better suited for molecular targeting. These include the Fab fragments, single chain variable fragments (scFv), minibodies, diabodies and nanobodies.Citation2,Citation92
Aptamers
Aptamers are small, single-stranded RNA or DNA sequences of oligonucleotides that can be designed as ligands capable of binding to targets with high sensitivity and specificity.Citation93,Citation94 They are small in size (usually approximately 15 kDa), and have less immunogenicity with respect to monoclonal antibodies or other macromolecules, leading to better stability and biodistribution.Citation95,Citation96 Such biomolecules fold by intramolecular interactions into unique three-dimensional conformations and topologies, having the ligand-binding characteristics needed for efficient target (receptor/antigen) affinity. Selective aptamers, with binding affinity toward a specific target, are currently being identified by an in vitro chemical process known as “systemic evolution of ligands by exponential enrichment” (SELEX).Citation97 In this process, which can be easily scaled up at a relatively low cost, a library of ten random oligonucleotides is enriched in order to identify the prototype with the highest affinity and specificity. Using this technology, Langer and Farokhzad designed and developed customized controlled-release nanoparticles loaded with docetaxel and having tumortargeting capability toward prostate-specific membrane antigen (PSMA).Citation40,Citation86,Citation87,Citation98,Citation99 Targeted nanoparticles have been obtained by decorating their shell surface with RNA A10-aptamer to bind PSMA,Citation100–Citation102 a clinically validated tumor marker that is not only overexpressed on the surface of prostate cancers but also on the neovasculature of many solid tumors. This approach constituted the proof-of principle for development of the first targeted polymeric nanoformulation (ie, BIND-014), which is now approved for clinical use (see below).
Proteins and peptide-based targeting molecules
Several endogenous proteins capable of selectively binding to specific receptors on membrane cells can be used for targeting purposes via receptor-mediated endocytosis.Citation103 For example, transferrin, an iron-transporting protein that binds specifically to transferrin receptors (TfR) located on the cell surface, has been used to transport nanoparticles into different types of cells.Citation103,Citation104 However, their effectiveness for targeting purposes may be limited by their immunogenicity and susceptibility to early clearance. The target receptors for these proteins are also commonly expressed on various types of nontargeted cells, which can lead to unwanted off-target effects.
Peptide-based ligands are emerging as attractive alternative targeting molecules due to their small size, high stability, and relatively low immunogenicity as compared with many proteins. Identification of specific targeting ligands has usually derived from previously or newly focused peptide phage/bacterial/plasmid peptide display libraries, as well as use of newer and easier screening technologies.Citation105 Like monoclonal antibodies and aptamers, peptides can bind to several molecular targets with a high degree of affinity and specificity, and are easy to manufacture by conjugation to polymers, lipids, and various nanoparticle surfaces.Citation106,Citation107
Small molecules
Small molecules, usually defined as low molecular weight organic molecules with a molecular weight <500 Da, constitute a promising class of targeting molecules because of their small size, high stability, chemical management, and low production cost.Citation57,Citation58,Citation108 Some further advantages of small molecules as targeting ligands include: availability of suitable facile coupling chemical methods for their conjugation; the possibility to modulate ligand densities and charge on nanoparticle surfaces, since these parameters can affect stability, size, and morphology, as well as targeting efficiency; availability of a wide range of targeting ligands with variable physicochemical properties and a variety of functional groups; fewer immunogenic effects in vivo; and reproducible, scalable, and economical manufacturing. Among the large number of small molecules identified as potential targeting ligands, folic acid has been one of the most extensively studied and used ligands in targeted drug delivery.Citation108,Citation109 Due to its high affinity for folate receptors, which are overexpressed in many types of tumor cells, the vitamin folate has also been used to deliver drug conjugates and many drug delivery systems (ie, liposomes, polymeric nanoparticles, dendrimers) to selectively accumulate drugs into cancer cells using folate receptor-mediated endocytosis.Citation110
Pomper et al have previously identified small hydrophilic molecules from a series of urea-based PSMA inhibitors as novel diagnostic agents capable of targeting the PSMA receptor in prostate cancer cells with affinity and specificity similar to that of antibodies and aptamers.Citation111–Citation113 Interestingly, a small molecule belonging to this class of molecules, ie, the pseudomimetic dipeptide 2-{[(5-amino-1-carboxypentyl) carbamoyl]amino}pentanedioic acid,Citation111 already used by Sechi et al for development of (−)-epigallocatechin 3-gallate-loaded PSMA-targeted nanoparticlesCitation114 and by Chandran et al for preparation of docetaxel-PLA/PCL-based targeted nanoparticles,Citation115 was also used as a targeting ligand in the development of BIND-014.Citation116
Concerning other small molecules that could be used as targeting ligands, carbohydrates, ie, sugar-based compounds, have also gained attention due to their low molecular weight, high availability and biocompatibility, and ease of production. Several of these carbohydrate ligands target membrane carbohydrate-binding proteins (membrane lectins) that are differentially expressed on the cellular and intracellular membranes, and some carbohydrates, such as mannose, glucose, galactose, and their derivatives, have been considered as potential ligands for carriers in cell-selective drug delivery.Citation117,Citation118
The clinical translation of the above-described technologies has ushered in a new era in the development of innovative therapeutic nanoparticles that are capable of targeting and controlled release, also considering the possibility of codelivery of multiple active agents.
Clinical development of targeted nanoparticles
To date, only three targeted liposomes and four targeted polymeric nanoparticles have progressed in clinical development (). The prototype that reach clinical trials was MCC-465, a new generation of liposome-encapsulated doxorubicin with a surface covered by both PEG and antigen-binding fragments [F(ab′)2] to confer immune shielding and targeting, respectively, that is used in the treatment of human stomach cancer.Citation119 Another site-directed liposome is SGT53-01, a TfR-functionalized nanoformulation containing a chain antibody fragment (TfRscFv) as the targeting ligand. It has been designed to carry the p53 tumor suppressor gene to cancer cells, and is currently undergoing Phase I clinical trials in combination with doxorubicin for the treatment of solid tumors.Citation120 The third site-specific liposomal nanocarrier is MBP-426, a TfR-targeted liposome encapsulating oxaliplatin, that has been used clinically for the treatment of a variety of solid tumors.Citation121,Citation122
With regard to targeted polymeric nanoformulations, CALAA-01 was the first nanotherapeutic to reach clinical development for siRNA delivery in 2008.Citation123 This nanosystem consists of TfR-targeted cyclodextrin-based PEGylated nanoparticles containing siRNA, and is capable of reducing expression of the M2 subunit of ribonucleotide reductase. The safety of CALAA-01 was evaluated in a Phase I clinical trial by intravenous administration to adults with solid tumors refractory to standard of care therapies.Citation124
More recently, a new antiepidermal growth factor receptor nanoimmunoliposome loaded with doxorubicin (ie, C225-ILS-DOX) entered Phase I investigation for the treatment of solid tumors.Citation125 Fab fragments of the chimeric monoclonal antibody cetuximab (C225, Erbitux) were covalently conjugated to the liposome membrane to target nanoparticles against tumor cells overexpressing EGFR ().Citation126
Focusing on the first clinically tested targeted nanomedicine, a major effort has made on BIND-014 (),Citation116 a new docetaxel formulation developed by a team led by Langer and Farokhzad at the Massachusetts Institute of Technology, Harvard Medical School, and BIND Therapeutics, as a programmable nanomedicine that recently entered Phase II clinical testing for the treatment of patients with solid tumors (). The development of BIND-014, along with its pharmacobiologic profile and potential clinical outcomes, is discussed in the following sections.
Figure 5 Graphic representation of BIND-014 composed of a biodegradable and hydrophobic PLA polymeric core and a hydrophilic PEG corona decorated with small-molecule (a pseudomimetic PSMA-directed dipeptide) targeting ligands, and a semisynthetic taxane (docetaxel) as an encapsulated anticancer drug.
Note: Images adapted with the permission of BIND Therapeutics (by Gael McGill), Harvard Medical School, and Digizyme Inc.
Abbreviations: PLA, poly(lactic acid); PEG, poly(ethylene glycol); PSMA, prostate-specific membrane antigen.
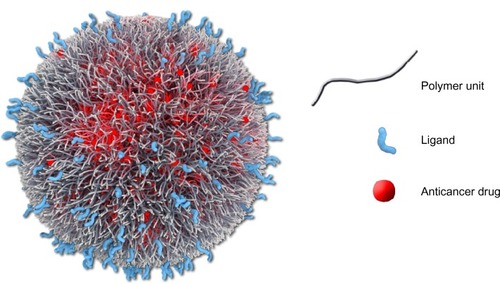
Pharmacology, mode of action, and pharmacokinetics of BIND-014
Development of BIND-014
The clinical translation of a targeted drug delivery nanosystem is strictly related to an optimal and synergistic combination of several physicochemical features, including polymer type, size, surface charge, hydrophilic/hydrophobic balance, ligand type and density, drug properties (type, solubility, loading, kinetics), and in vivo release capability (ie, tissue penetration, navigation across membrane layers and biological barriers, immune evasion or “stealth” characteristics, and cellular binding, uptake, and internalization processes).Citation8,Citation40,Citation56,Citation58,Citation116
Considering these issues, BIND Biosciences (now BIND Therapeutics) developed customized controlled-release nanoparticles by using new high-throughput technology to achieve multifactorial optimization of polymeric nanosystems. The history of this project started with the pioneering work of Langer and Farokhzad that led to proof-of-concept for the development of innovative drug delivery vehicles composed of several safe or FDA-approved materials as biocompatible polymers (ie, PLA, PLGA, and PEG) and aptamers to target PSMA.Citation40,Citation85,Citation86 Such nanoparticles were loaded with docetaxel, a semisynthetic taxane and one of the most commonly used cancer chemotherapy drugs.Citation127–Citation129 More specifically, a hydrophilic spacer (ie, PEG) was incorporated into the outer shell of the nanoparticle in order to achieve minimal self-self and self-nonself interaction, to escape capture by the reticuloendothelial system, and to reduce opsonization, thus enabling “stealth” properties for immune evasion.Citation42,Citation43,Citation130 The tumor-targeting capability of these nanoparticles was obtained by “decorating” the shell surface with RNA A10-aptamer (then replaced with a small molecule urea-based PSMA inhibitor) to bind PSMA.Citation40 The biological results demonstrated that the encapsulated drug efficiently interacted with the tumor and selectively delivered docetaxel to prostate cancer cells.Citation40,Citation86,Citation87
Optimization of such construct to BIND-014 was done by adopting a modular self-assembly approach involving use of prefunctionalized polymeric materials to generate a combinatorial library of more than 100 self-assembled polymeric targeted nanoparticles of varying composition. This library was designed by introducing physicochemical diversity into each nanoparticle via a focused number of components, and by systematically varying both the nanoformulation and the critical process parameters ().Citation116
Figure 6 Development of BIND-014 by high-throughput technology. Multifactorial optimization of polymeric nanosystems was performed by varying a range of formulation process parameters and physicochemical nanoparticle properties. The green dotted line indicates optimized nanoparticle parameters.
Notes: Molecular weight expressed in Dalton (Da); zeta potential expressed in millivolts (mV); diameter expressed in nanometers (nm); initial release rate calculated by mass flow per unit time (%/h). From Hrkach J, Von Hoff D, Mukkaram Ali M, et al. Preclinical develop ment and clinical translation of a PSMA-targeted docetaxel nanoparticle with a differentiated pharmacological profile. Sci Transl Med. 2012;4(128):128ra139.Citation116 Adapted with permission from AAAS.
Abbreviations: NP, nanoparticles; PEG, poly(ethylene glycol); PL(G)A, poly(lactic acid) or poly(D,L-lactide-co-glycolide).
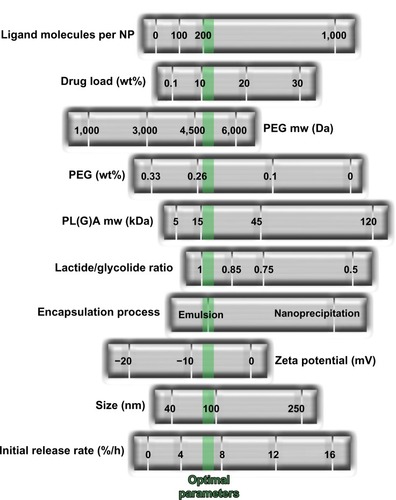
These targeted docetaxel-loaded nanoparticles were constructed with the above mentioned ternary structure and comprise a biodegradable copolymeric core (PLA or PLGA and PEG), a pseudomimetic dipeptide as a PSMA-targeting ligand, and docetaxel as the payload anticancer drug. Importantly, because scale-up of production for nanoformulations is a huge challenge that has precluded many prototypes going from bench scale experiments to clinical trials, several key factors that can contribute to the translation of this nanotherapeutic platform were identified. These include the use of FDA-approved polymeric materials that have already been validated for preparation of pharmaceutical products and biomedical application. Moreover, a major goal has been reached by the development of a robust, scalable, and continuous process for efficient nanoparticle formation by using a suitable model (encapsulation of a hydrophobic cancer-preventive compound) to obtain high drug loading and encapsulation efficiency. For example, instantaneous mixing of materials during nanoformulation provides the opportunity to achieve the higher drug loading parameter.Citation116 Therefore, understanding of the fundamental thermodynamics and kinetics to optimize production of the nanoparticles in a repeatable and scalable manner constitutes a key aspect to enable manufacturing of clinical candidate nanoparticles in the kilogram quantities needed for clinical use. The evolution of this process resulted in BIND-014, the first targeted and controlled release polymeric nanoparticle for cancer chemotherapy to reach clinical Phase I trials in January 2011.Citation116,Citation131
Pharmacobiologic profile of BIND-014
Overall, BIND-014 has been demonstrated to be up to 10-fold more effective in delivering docetaxel to tumors with respect to an equivalent dose of free drug in multiple animal models, with no increase in toxicity.Citation116 In particular, as far as tissue biodistribution is concerned, intravenous administration of nanoparticles in in vivo experiments in rats showed a blood circulation half-life of about 20 hours, with higher plasma docetaxel concentrations relative to those of the free drug (as formulated in Taxotere™), where encapsulation prevents rapid distribution of docetaxel from the plasma compartment to tissues (lower accumulation of nanoparticles being detected in liver and bone marrow with respect to plasma). The in vitro release and in vivo pharmacokinetic results also demonstrated that the large amount of docetaxel released from these polymeric nanoprototypes resulted in a long blood circulation time, with sustained release of the drug, leading to an increase of up to 1,000-fold in the docetaxel rate in the plasma, available to reach tumor sites with respect to the conventional docetaxel formulation (ie, Taxotere).Citation116
Moreover, toxicologic characterization of the composition of free nanoparticles (without drug), targeted nanoparticles, and the respective nontargeted nanoparticles, has been done in a single-dose tolerability study in rats. The results indicated that the ingredients used in the nanoformulation are in general well tolerated, without observation of the hypersensitivity or anaphylactic/anaphylactoid reactions that have been seen after infusion of higher doses of nanoparticles.Citation116
The in vivo antitumor activity of the targeted docetaxel-loaded nanoparticles was assessed in mice bearing PSMA-expressing (LNCaP) and PSMA-negative ([breast cancer (MX-1) and nonsmall cell lung cancer (NCI-H460)]) xenograft models, using targeted and nontargeted nanoparticles and free docetaxel for comparison.Citation116 For nanoparticles with a long circulation time, an active targeting mechanism improves antitumor efficacy compared with passive targeting nanoparticles in PSMA-positive models. Mechanistically, once nanoparticles enter the tumor tissue, their retention in tumor tissue and specific uptake by cancer cells can be facilitated by active targeting and receptor-mediated endocytosis. This process would result in higher intracellular drug concentrations and increased cytotoxicity. The similar effects of targeted and nontargeted nanoparticles in non-PSMA-expressing tumors are presumably due to increased drug accumulation at tumor sites via the enhanced permeability and retention effect.
Further, the pharmacokinetic profile of the docetaxel-loaded nanoparticles (compared with that of the free drug) was investigated in tumor-bearing mice, healthy rats, and nonhuman primates to assess the translation of pharmacokinetic results across different species. The results indicate a high concentration of drug in the plasma compartment, as well as an effective controlled drug release performance.Citation116
These preclinical results suggest that nanotherapeutics developed in this way may be beneficial for treatment of solid tumors by improving the therapeutic index of docetaxel through targeting and controlled release of the drug in the tumor microenvironment. Moreover, since the molecular target, PSMA, is expressed on prostate tumor cells and on the neovasculature of other solid tumors, these nanosystems have the potential to increase the utility of docetaxel in the treatment of a range of cancers.
Safety, tolerability, and efficacy of BIND-014 and range of cancer targets
The clinical data for BIND-014 are consistent with the observations outlined above. The behavior of BIND-014 in preclinical studies suggests that docetaxel is more effective when formulated as BIND-014 than as Taxotere. More specifically, on the basis of these promising preclinical results, a Phase I clinical trial was undertaken to assess the dose-limiting toxicity and the maximum tolerated dose of BIND-014.Citation116,Citation131 The nanochemotherapy was performed by intravenous infusion of BIND-014 to patients with advanced or metastatic cancer, using two dosing schedules, ie, once every 3 weeks (Q3W) and once weekly for 3 weeks followed by one week of no treatment over a 4-week cycle (Q1W). To date, BIND-014 has been clinically tested in over 45 patients with advanced or metastatic cancer who have failed prior therapies.
Based on the widespread expression of PSMA in solid tumor neovasculature, the activity of free docetaxel in many common types of cancer, and the efficacy of BIND-014 in preclinical models of prostate and other cancers, a Phase I study was undertaken to evaluate the efficacy of BIND-014 in a panel of solid tumors. It is worth noting that the pharmacokinetics of docetaxel when released from BIND-014 were markedly similar in humans to those observed in preclinical studies, and that the pharmacokinetics of BIND-014 were different from those of Taxotere in both the preclinical and clinical studies. In the Q3W portion of the study, BIND-014 was well tolerated with predictable and manageable toxicities, and no unexpected toxicity has been observed with BIND-014. BIND-014 was demonstrated to be essentially safe and well tolerated, with transient and manageable neutropenia as the dose-limiting toxicity. Other treatment-related adverse events included mild neuropathy, fatigue, mucositis, diarrhea, fluid retention, anemia, rash, hair loss, infusion-related reactions, nail changes, and nausea and vomiting, which are commonly associated with docetaxel as well as many other anticancer drugs.Citation132 Further, the maximum tolerated dose was established to be 60 mg/m2 as a 60-minute infusion under the Q3W treatment regimen.Citation116 Using this dose regimen, BIND-014 demonstrated antitumor efficacy in nine of 28 patients treated, ranging from one complete response (cervical cancer), three partial responses (non-small-cell lung cancer, metastatic/castration-resistant prostate cancer, and ampullary cancer), and five additional patients with stabilization of disease lasting longer that 12 weeks (pancreatic, colorectal, gallbladder, tonsillar, and anal cancer). These results indicate that the disappearance of cancer evidences in response to treatment, while a partial response refers to a decrease in the size of the tumor or in the extent of cancer in the body.
In summary, the results of a Phase I clinical trial indicate that BIND-014 has a different pharmacologic profile with respect to free docetaxel, including pharmacokinetic properties consistent with a prolonged circulation half-life for BIND-014 and retention of docetaxel in the vascular compartments, with multiple cases of tumor shrinkage at doses up to five times less than the docetaxel dose typically administered.Citation133 These results demonstrate that a combination of long nanoparticle circulation time, nanoparticle targeting, and controlled release of docetaxel markedly increases the amount of docetaxel delivered to the tumor, and achieves tumor growth inhibition for an extended period of time. Although the Q1W portion of this study is ongoing, Phase II development of BIND-014 as second-line therapy for patients with non-small-cell lung cancer (NCT01792479)Citation134 and those with metastatic castrate-resistant prostate cancer (NCT01812746)Citation135 was started in 2013 using the Q3W dosing schedule. Further clinical studies to evaluate BIND-014 in a broad range of solid tumors are currently under consideration.
Place in therapy
Nanotechnologies are having a significant impact on drug delivery, and over recent years, several first-generation therapeutic nanoproducts have been moving ahead towards clinical development. In particular, targeted polymeric nanoparticles, capable of increased cell uptake and enhanced accumulation in target tissue, can be successfully obtained by attaching specific binding entities onto the surface of the nanoparticles. Therefore, active targeting, along with other targeting-based approaches, is envisaged to provide an effective strategy, and several ligand-targeted nanotherapeutics are either approved or under clinical evaluation, leading to second-generation nanomedicines. However, although there are many useful and validated chemical methods for functionalizing nanoparticles with a wide range of targeting ligands, several important issues, including the optimal interplay of physicochemical features, need to be addressed before translation from preclinical to clinical development. Importantly, careful detailing of the physicochemical characteristics of nanoparticles that dictate their in vivo efficacy and a better understanding of the biological and (patho)physiologic principles of drug targeting are need to overcome the current bottlenecks in research and development of rationally designed nanoparticles.
The “drug-targeting” paradigm can be exploited to finally formulate therapeutic carriers that can selectively treat neoplastic tissues without affecting normal cells. The use of targeted nanoparticles in the treatment of cancer, developed in the pioneering work of Farokhzad and Langer, has been validated by the Phase II clinically used prototype BIND-014 (marketed by BIND Therapeutics under the brand name Accurins™), a polymeric drug delivery nanovehicle containing the chemotherapeutic agent docetaxel, which is approved for use in the treatment of several common cancers, including breast, lung, and prostate.
To date, other complex targeted nanosystems addressed to various cancers, also combining diagnostic and therapeutic agents, or that can trigger drug release at the target site when exposed to external stimuli, are currently in clinical development. At this stage, BIND-014 can reasonably be seen as representing an evolution of the “magic bullet” concept, the first description of the drug-targeting paradigm, that can also be used to develop programmable and personalized nanomedicine for routine use in clinic practice.
Acknowledgments
The authors gratefully acknowledge the Regione Autonoma della Sardegna for financial support grant CRP-25920 “Development of novel targeted nanodevices for the prevention, diagnosis and treatment of prostate cancer”, awarded to MS within the frame of “Legge regionale n 7/2007, promozione della ricerca scientifica e dell’innovazione tecnologica in Sardegna, – Annualità 2010”. They also thank Gael McGill (Harvard Medical School) and Digizyme Inc. for their permission to adapt and use the Accurins image.
Disclosure
The authors report no conflicts of interest in this work.
References
- KimBYRutkaJTChanWCNanomedicineN Engl J Med2010363252434244321158659
- PeerDKarpJMHongSFarokhzadOCMargalitRLangerRNanocarriers as an emerging platform for cancer therapyNat Nanotechnol200721275176018654426
- ZhangLGuFXChanJMWangAZLangerRSFarokhzadOCNanoparticles in medicine: therapeutic applications and developmentsClin Pharmacol Ther200883576176917957183
- RiehemannKSchneiderSWLugerTAGodinBFerrariMFuchsHNanomedicine – challenge and perspectivesAngew Chem Int Ed Engl200948587289719142939
- DoaneTLBurdaCThe unique role of nanoparticles in nanomedicine: imaging, drug delivery and therapyChem Soc Rev20124172885291122286540
- FerrariMCancer nanotechnology: opportunities and challengesNat Rev Cancer20055316117115738981
- DavisMEChenZGShinDMNanoparticle therapeutics: an emerging treatment modality for cancerNat Rev Drug Discov20087977178218758474
- FarokhzadOCLangerRImpact of nanotechnology on drug deliveryACS Nano200931162019206243
- PetrosRADeSimoneJMStrategies in the design of nanoparticles for therapeutic applicationsNat Rev Drug Discov20109861562720616808
- LammersTKiesslingFHenninkWEStormGDrug targeting to tumors: principles, pitfalls and (pre-) clinical progressJ Control Release2012161217518721945285
- JainKKRole of nanobiotechnology in the development of personalized medicineNanomedicine (Lond)20094324925219331532
- ZhangXQXuXBertrandNPridgenESwamiAFarokhzadOCInteractions of nanomaterials and biological systems: Implications to personalized nanomedicineAdv Drug Deliv Rev201264131363138422917779
- GindyMEPrud’hommeRKMultifunctional nanoparticles for imaging, delivery and targeting in cancer therapyExpert Opin Drug Deliv20096886587819637974
- JanibSMMosesASMacKayJAImaging and drug delivery using theranostic nanoparticlesAdv Drug Deliv Rev201062111052106320709124
- LammersTKiesslingFHenninkWEStormGNanotheranostics and image-guided drug delivery: current concepts and future directionsMol Pharm2010761899191220822168
- Fernandez-FernandezAManchandaRMcGoronAJTheranostic applications of nanomaterials in cancer: drug delivery, image-guided therapy, and multifunctional platformsAppl Biochem Biotechnol20111657–81628165121947761
- YooDLeeAHShinTHCheonJTheranostic magnetic nanoparticlesAcc Chem Res2011441086387421823593
- LeeDEKooHSunICRyuAHKimKKwonICMultifunctional nanoparticles for multimodal imaging and theragnosisChem Soc Rev20124172656267222189429
- BaeKHChungHJParkTGNanomaterials for cancer therapy and imagingMol Cells201131429530221360197
- TaylorAWilsonKMMurrayPFernigDGLevyRLong-term tracking of cells using inorganic nanoparticles as contrast agents: are we there yet?Chem Soc Rev20124172707271722362426
- Villalonga-BarberCMicha-ScrettasMSteeleBRGeorgopoulosADemetzosCDendrimers as biopharmaceuticals: synthesis and propertiesCurr Top Med Chem20088141294130918855710
- CliftMJStoneVQuantum dots: an insight and perspective of their biological interaction and how this relates to their relevance for clinical useTheranostics20122766868022896769
- YamashitaTYamashitaKNabeshiHCarbon nanomaterials: efficacy and safety for nanomedicineMaterials201252350363
- ParthaRConyersJLBiomedical applications of functionalized fullerene-based nanomaterialsInt J Nanomedicine2009426127520011243
- KaurRBadeaINanodiamonds as novel nanomaterials for biomedical applications: drug delivery and imaging systemsInt J Nanomedicine2013820322023326195
- KlumppCKostarelosKPratoMBiancoAFunctionalized carbon nanotubes as emerging nanovectors for the delivery of therapeuticsBiochim Biophys Acta20061758340441216307724
- ZhuSXuGSingle-walled carbon nanohorns and their applicationsNanoscale20102122538254920957266
- SperlingRARivera-GilPZhangFZanellaMParakWJBiological applications of gold nanoparticlesChem Soc Rev20083791896190818762838
- CaiWGaoTHongHSunJApplications of gold nanoparticles in cancer nanotechnologyNanotechnol Sci Appl20081173224198458
- LalSClareSEHalasNJNanoshell-enabled photothermal cancer therapy: impending clinical impactAcc Chem Res200841121842185119053240
- GiljohannDASeferosDSDanielWLMassichMDPatelPCMirkinCAGold nanoparticles for biology and medicineAngew Chem Int Ed Engl201049193280329420401880
- DreadenECAlkilanyAMHuangXMurphyCJEl-SayedMAThe golden age: gold nanoparticles for biomedicineChem Soc Rev20124172740277922109657
- BanghamADStandishMMWatkinsJCDiffusion of univalent ions across the lamellae of swollen phospholipidsJ Mol Biol19651312382525859039
- TorchilinVPRecent advances with liposomes as pharmaceutical carriersNat Rev Drug Discov20054214516015688077
- AllenTMCullisPRDrug delivery systems: entering the mainstreamScience200430356651818182215031496
- LangerRFolkmanJPolymers for the sustained release of proteins and other macromoleculesNature19762635580797800995197
- GrefRMinamitakeYPeracchiaMTTrubetskoyVTorchilinVLangerRBiodegradable long-circulating polymeric nanospheresScience19942635153160016038128245
- LangerRDrug delivery and targetingNature1998392Suppl 66795109579855
- AlexisFPridgenEMLangerRFarokhzadOCNanoparticle technologies for cancer therapyHandb Exp Pharmacol2010197558620217526
- ShiJXiaoZKamalyNFarokhzadOCSelf-assembled targeted nanoparticles: evolution of technologies and bench to bedside translationAcc Chem Res201144101123113421692448
- WalkeyCDChanWCUnderstanding and controlling the interaction of nanomaterials with proteins in a physiological environmentChem Soc Rev20124172780279922086677
- AlexisFPridgenEMolnarLKFarokhzadOCFactors affecting the clearance and biodistribution of polymeric nanoparticlesMol Pharm20085450551518672949
- OtsukaHNagasakiYKataokaKPEGylated nanoparticles for biological and pharmaceutical applicationsAdv Drug Deliv Rev200355340341912628324
- AvgoustakisKPegylated poly(lactide) and poly(lactide-co-glycolide) nanoparticles: preparation, properties and possible applications in drug deliveryCurr Drug Deliv20041432133316305394
- BetancourtTByrneJDSunaryoNPEGylation strategies for active targeting of PLA/PLGA nanoparticlesJ Biomed Mater Res A200991126327618980197
- JokerstJVLobovkinaTZareRNGambhirSSNanoparticle PEGylation for imaging and therapyNanomedicine (Lond)20116471572821718180
- KnopKHoogenboomRFischerDSchubertUSPoly(ethylene glycol) in drug delivery: pros and cons as well as potential alternativesAngew Chem Int Ed Engl201049366288630820648499
- HanahanDWeinbergRAThe hallmarks of cancerCell20001001577010647931
- HanahanDWeinbergRAHallmarks of cancer: the next generationCell2011144564667421376230
- GabizonAShmeedaHBarenholzYPharmacokinetics of pegylated liposomal doxorubicin: review of animal and human studiesClin Pharmacokinet200342541943612739982
- US Food and Drug AdministrationDrugs Available from: http://www.accessdata.fda.gov/scripts/cder/drugsatfda/index.cfmAccessed November 5, 2013
- HuynhNTPassiraniCSaulnierPBenoitJPLipid nanocapsules: a new platform for nanomedicineInt J Pharm2009379220120919409468
- GradisharWJTjulandinSDavidsonNPhase III trial of nanoparticle albumin-bound paclitaxel compared with polyethylated castor oil-based paclitaxel in women with breast cancerJ Clin Oncol200523317794780316172456
- MontanaMDucrosCVerhaeghePTermeTVanellePRathelotPAlbumin-bound paclitaxel: the benefit of this new formulation in the treatment of various cancersJ Chemother2011232596621571619
- MakadiaHKSiegelSJPoly lactic-co-glycolic acid (PLGA) as biodegradable controlled drug delivery carrierPolymers (Basel)2011331377139722577513
- ElsabahyMWooleyKLDesign of polymeric nanoparticles for biomedical delivery applicationsChem Soc Rev20124172545256122334259
- NicolasJMuraSBrambillaDMackiewiczNCouvreurPDesign, functionalization strategies and biomedical applications of targeted biodegradable/biocompatible polymer-based nanocarriers for drug deliveryChem Soc Rev20134231147123523238558
- KamalyNXiaoZValenciaPMRadovic-MorenoAFFarokhzadOCTargeted polymeric therapeutic nanoparticles: design, development and clinical translationChem Soc Rev20124172971301022388185
- StrebhardtKUllrichAPaul Ehrlich’s magic bullet concept: 100 years of progressNat Rev Cancer20088647348018469827
- CollinsIWorkmanPNew approaches to molecular cancer therapeuticsNat Chem Biol200621268970017108987
- MatsumuraYMaedaHA new concept for macromolecular therapeutics in cancer chemotherapy: mechanism of tumoritropic accumulation of proteins and the antitumor agent smancsCancer Res19864612 Pt 1638763922946403
- MaedaHWuJSawaTMatsumuraYHoriKTumor vascular permeability and the EPR effect in macromolecular therapeutics: a reviewJ Control Release2000651–227128410699287
- CarmelietPJainRKAngiogenesis in cancer and other diseasesNature2000407680124925711001068
- DanquahMKZhangXAMahatoRIExtravasation of polymeric nanomedicines across tumor vasculatureAdv Drug Deliv Rev201163862363921144874
- JainRKDelivery of molecular and cellular medicine to solid tumorsAdv Drug Deliv Rev2001461–314916811259838
- JainRKStylianopoulosTDelivering nanomedicine to solid tumorsNat Rev Clin Oncol201071165366420838415
- HatakeyamaHAkitaHHarashimaHA multifunctional envelope type nanodevice (MEND) for gene delivery to tumours based on the EPR effect: a strategy for overcoming the PEG dilemmaAdv Drug Deliv Rev201163315216020840859
- ValleJWArmstrongANewmanCA phase 2 study of SP1049C, doxorubicin in P-glycoprotein-targeting pluronics, in patients with advanced adenocarcinoma of the esophagus and gastroesophageal junctionInvest New Drugs20112951029103720179989
- MatsumuraYHamaguchiTUraTPhase I clinical trial and pharmacokinetic evaluation of NK911, a micelle-encapsulated doxorubicinBr J Cancer200491101775178115477860
- TongRChengJAnticancer polymeric nanomedicinesPolym Rev (Phila Pa)2007473345381
- SingerJWPaclitaxel poliglumex (XYOTAX, CT-2103): a macromolecular taxaneJ Control Release20051091–312012616297482
- YoungCSchluepTHwangJEliasofSCRLX101 (formerly IT-101)-a novel nanopharmaceutical of camptothecin in clinical developmentCurr Bioact Compd20117181422081768
- Clinical Trials.govStudy of paclitaxel in patients with ovarian cancer Available from: http://clinicaltrials.gov/ct2/show/results/NCT00989131Accessed November 5, 2013
- TagamiTErnstingMJLiSDEfficient tumor regression by a single and low dose treatment with a novel and enhanced formulation of thermosensitive liposomal doxorubicinJ Control Release2011152230330921338635
- SeetharamuNKimEHochsterHMartinFMuggiaFPhase II study of liposomal cisplatin (SPI-77) in platinum-sensitive recurrences of ovarian cancerAnticancer Res201030254154520332467
- Clinical Trials.govA Phase I trial of nanoliposomal CPT-11 (NL CPT-11) in patients with recurrent high-grade gliomas Available from: http://clinicaltrials.gov/show/NCT00734682Accessed November 5, 2013
- TardiPJohnstoneSHarasymNIn vivo maintenance of synergistic cytarabine:daunorubicin ratios greatly enhances therapeutic efficacyLeuk Res200933112913918676016
- ByrneJDBetancourtTBrannon-PeppasLActive targeting schemes for nanoparticle systems in cancer therapeuticsAdv Drug Deliv Rev200860151615162618840489
- AllenTMLigand-targeted therapeutics in anticancer therapyNat Rev Cancer200221075076312360278
- CantonIBattagliaGEndocytosis at the nanoscaleChem Soc Rev20124172718273922389111
- ArapWPasqualiniRRuoslahtiECancer treatment by targeted drug delivery to tumor vasculature in a mouse modelScience199827953493773809430587
- MitraAMulhollandJNanAMcNeillEGhandehariHLineBRTargeting tumor angiogenic vasculature using polymer-RGD conjugatesJ Control Release2005102119120115653145
- OerlemansCBultWBosMStormGNijsenJFHenninkWEPolymeric micelles in anticancer therapy: targeting, imaging and triggered releasePharm Res201027122569258920725771
- DeckersRMoonenCTUltrasound triggered, image guided, local drug deliveryJ Control Release20101481253320709123
- GuFLangerRFarokhzadOCFormulation/preparation of functionalized nanoparticles for in vivo targeted drug deliveryMethods Mol Biol200954458959819488725
- FarokhzadOCChengJTeplyBATargeted nanoparticle-aptamer bioconjugates for cancer chemotherapy in vivoProc Natl Acad Sci U S A2006103166315632016606824
- GuFZhangLTeplyBAPrecise engineering of targeted nanoparticles by using self-assembled biointegrated block copolymersProc Natl Acad Sci U S A200810572586259118272481
- YuBTaiHCXueWLeeLJLeeRJReceptor-targeted nanocarriers for therapeutic delivery to cancerMol Membr Biol201027728629821028937
- SchramaDReisfeldRABeckerJCAntibody targeted drugs as cancer therapeuticsNat Rev Drug Discov20065214715916424916
- ChamesPVan RegenmortelMWeissEBatyDTherapeutic antibodies: successes, limitations and hopes for the futureBr J Pharmacol2009157222023319459844
- NobsLBucheggerFGurnyRAllemannEBiodegradable nanoparticles for direct or two-step tumor immunotargetingBioconjug Chem200617113914516417262
- WeinerLMAdamsGPNew approaches to antibody therapyOncogene200019536144615111156528
- EllingtonADSzostakJWIn vitro selection of RNA molecules that bind specific ligandsNature199034662878188221697402
- TuerkCGoldLSystematic evolution of ligands by exponential enrichment: RNA ligands to bacteriophage T4 DNA polymeraseScience199024949685055102200121
- KeefeADPaiSEllingtonAAptamers as therapeuticsNat Rev Drug Discov20109753755020592747
- XiaoZFarokhzadOCAptamer-functionalized nanoparticles for medical applications: challenges and opportunitiesACS Nano2012653670367622574989
- DanielsDAChenHHickeBJSwiderekKMGoldLA tenascin-C aptamer identified by tumor cell SELEX: systematic evolution of ligands by exponential enrichmentProc Natl Acad Sci U S A200310026154161542114676325
- FarokhzadOCJonSKhademhosseiniATranTNLavanDALangerRNanoparticle-aptamer bioconjugates: a new approach for targeting prostate cancer cellsCancer Res200464217668767215520166
- ChengJTeplyBASherifiIFormulation of functionalized PLGA-PEG nanoparticles for in vivo targeted drug deliveryBiomaterials200728586987617055572
- ChangSSO’KeefeDSBacichDJReuterVEHestonWDGaudinPBProstate-specific membrane antigen is produced in tumor-associated neovasculatureClin Cancer Res19995102674268110537328
- GhoshAHestonWDTumor target prostate specific membrane antigen (PSMA) and its regulation in prostate cancerJ Cell Biochem200491352853914755683
- SchulkeNVarlamovaOADonovanGPThe homodimer of prostate-specific membrane antigen is a functional target for cancer therapyProc Natl Acad Sci U S A200310022125901259514583590
- ChouLYMingKChanWCStrategies for the intracellular delivery of nanoparticlesChem Soc Rev201140123324520886124
- ChoiCHAlabiCAWebsterPDavisMEMechanism of active targeting in solid tumors with transferrin-containing gold nanoparticlesProc Natl Acad Sci U S A201010731235124020080552
- BrissetteRPrendergastJKGoldsteinNIIdentification of cancer targets and therapeutics using phage displayCurr Opin Drug Discov Devel200693363369
- SugaharaKNTeesaluTKarmaliPPCoadministration of a tumor-penetrating peptide enhances the efficacy of cancer drugsScience201032859811031103520378772
- ChanJMZhangLTongRSpatiotemporal controlled delivery of nanoparticles to injured vasculatureProc Natl Acad Sci U S A201010752213221820133865
- TalekarMKendallJDennyWGargSTargeting of nanoparticles in cancer: drug delivery and diagnosticsAnticancer Drugs2011221094996221970851
- LuYLowPSFolate-mediated delivery of macromolecular anticancer therapeutic agentsAdv Drug Deliv Rev200254567569312204598
- LiangCYangYLingYHuangYLiTLiXImproved therapeutic effect of folate-decorated PLGA-PEG nanoparticles for endometrial carcinomaBioorg Med Chem201119134057406621641806
- MarescaKPHillierSMFemiaFJA series of halogenated heterodimeric inhibitors of prostate specific membrane antigen (PSMA) as radiolabeled probes for targeting prostate cancerJ Med Chem200952234735719111054
- ByunYMeaseRCLupoldSEPomperMGRecent development of diagnostic and therapeutic agents targeting glutamate carboxypeptidase II (GCPII)SupuranCTWinumJYDrug Design of Zinc-Enzyme Inhibitors: Functional, Structural, and Disease ApplicationsNew York, NYBinghe Wang Wiley Series in Drug Discovery and Development2009
- ZhouJNealeJHPomperMGKozikowskiAPNAAG peptidase inhibitors and their potential for diagnosis and therapyNat Rev Drug Discov20054121015102616341066
- SannaVPintusGRoggioAMTargeted biocompatible nanoparticles for the delivery of (−)-epigallocatechin 3-gallate to prostate cancer cellsJ Med Chem20115451321133221306166
- ChandranSSBanerjeeSRMeaseRCPomperMGDenmeadeSRCharacterization of a targeted nanoparticle functionalized with a urea-based inhibitor of prostate-specific membrane antigen (PSMA)Cancer Biol Ther20087697498218698158
- HrkachJVon HoffDMukkaram AliMPreclinical development and clinical translation of a PSMA-targeted docetaxel nanoparticle with a differentiated pharmacological profileSci Transl Med20124128128ra139
- WijagkanalanWKawakamiSHashidaMGlycosylated carriers for cell-selective and nuclear delivery of nucleic acidsFront Biosci (Landmark Ed)2011162970298721622215
- HashidaMNishikawaMYamashitaFTakakuraYCell-specific delivery of genes with glycosylated carriersAdv Drug Deliv Rev200152318719611718943
- MatsumuraYGotohMMuroKPhase I and pharmacokinetic study of MCC-465, a doxorubicin (DXR) encapsulated in PEG immunoliposome, in patients with metastatic stomach cancerAnn Oncol200415351752514998859
- ClinicalTrials.govSafety study of infusion of SGT-53 to treat solid tumors Available from: http://clinicaltrials.gov/show/NCT00470613Accessed November 5, 2013
- ClinicalTrials.govStudy of MBP-426 in patients with second line gastric, gastroesophageal, or esophageal adenocarcinoma Available from: http://clinicaltrials.gov/show/NCT00964080Accessed November 5, 2013
- SankhalaKKMitaACAdininRA phase I pharmacokinetic (PK) study of MBP-426, a novel liposome encapsulated oxaliplatinJ Clin Oncol200927S2535
- ClinicalTrials.govSafety study of CALAA-01 to treat solid tumor cancers Available from: http://clinicaltrials.gov/show/NCT00689065Accessed November 5, 2013
- DavisMEThe first targeted delivery of siRNA in humans via a self-assembling, cyclodextrin polymer-based nanoparticle: from concept to clinicMol Pharm20096365966819267452
- TrialsUnitedA Phase I study of doxorubicin-loaded anti-EGFR immunoliposomes in patients with advanced solid tumors Available from: http://www.trialsunited.com/studies/NCT01702129
- MamotCRitschardRVogelBA phase I study of doxorubicin-loaded anti-EGFR immunoliposomes in patients with advanced solid tumorsJ Clin Oncol20112915S3029
- GelmonKThe taxoids: paclitaxel and docetaxelLancet19943448932126712727967989
- MonteroAFossellaFHortobagyiGValeroVDocetaxel for treatment of solid tumours: a systematic review of clinical dataLancet Oncol20056422923915811618
- ZhangLZhangNHow nanotechnology can enhance docetaxel therapyInt J Nanomedicine201382927294123950643
- GrefRLuckMQuellecP‘Stealth’ corona-core nanoparticles surface modified by polyethylene glycol (PEG): influences of the corona (PEG chain length and surface density) and of the core composition on phagocytic uptake and plasma protein adsorptionColloids Surf B Biointerfaces2000183–430131310915952
- ClinicalTrials.govA study of BIND-014 given to patients with advanced or metastatic cancer Available from: http://clinicaltrials.gov/show/NCT01300533Accessed November 5, 2013
- BakerJAjaniJScotteFDocetaxel-related side effects and their managementEur J Oncol Nurs2009131495919201649
- Von HoffDDMitaMEisenbergPA Phase 1study of BIND-014, a PSMA-targeted nanoparticle containing docetaxel, in patients with refractory solid tumorsAbstract LB-203 presented at the annual meeting of the American Association for Cancer ResearchApril 6–10, 2013Washington, DC, USA
- ClinicalTrials.govA Phase 2 study to determine the safety and efficacy of BIND-014 (docetaxel nanoparticles injectable suspension) as second line therapy to patients with non-small cell lung cancer Available from: http://clinicaltrials.gov/show/NCT01792479Accessed November 5, 2013
- ClinicalTrials.govA Phase 2 study to determine the safety and efficacy of BIND-014 (docetaxel nanoparticles injectable suspension), administered to patients with metastatic castration-resistant prostate cancer Available from: http://clinicaltrials.gov/show/NCT01812746Accessed November 5, 2013