Abstract
Reconstructive urologists are constantly facing diverse and complex pathologies that require structural and functional restoration of urinary organs. There is always a demand for a biocompatible material to repair or substitute the urinary tract instead of using patient’s autologous tissues with its associated morbidity. Biomimetic approaches are tissue-engineering tactics aiming to tailor the material physical and biological properties to behave physiologically similar to the urinary system. This review highlights the different strategies to mimic urinary tissues including modifications in structure, surface chemistry, and cellular response of a range of biological and synthetic materials. The article also outlines the measures to minimize infectious complications, which might lead to graft failure. Relevant experimental and preclinical studies are discussed, as well as promising biomimetic approaches such as three-dimensional bioprinting.
Introduction
Reconstructive urology is a subspecialty dealing with repair, restoration, and replacement of parts or all of an organ of the urinary system.Citation1,Citation2 Reconstructive surgeons are continuously searching for an ultimate biocompatible material, which when needed can substitute segments of urinary tracts in preference to patient’s own tissues. As urologists always implement novel diagnostic techniques and therapeutic procedures to the field,Citation3,Citation4 tissue engineering became the up-to-date area of research especially to those involved with reconstructive procedures.
Autologous tissues, such as intestinal segments, skin, and oral mucosa, are the current substitutes for reconstruction.Citation5,Citation6 Nevertheless, there are numerous local and systemic complications associated with harvesting those tissues. The complications are clearly outlined in . Although tissue transfer techniques have been innovated throughout the years, aiming to reach the highest possible level of functional success, the reconstructive armamentarium requires an “off the shelf tissue” to limit patient’s morbidity. Modernization of reconstructive urology recently involved the introduction of minimally invasive surgeries such as endoscopic, laparoscopic, and robotic procedures. This realistically decreased patient morbidity, enhanced postoperative recovery, and improved cosmetic outcome.Citation7 Nevertheless, despite this “high technology”,Citation8 the functional outcome is rather similar to conventional surgeries. Using a “substitute” that physiologically behaves similar to urinary tissues will provide a practical solution to complex and recurrent cases, which are considered challenging even to experts.
Table 1 Contemporary reconstructive approaches for management of different urinary tract pathologies
The aim of this study was to highlight how biomimetic approaches are implemented in order to address the unmet needs in developing a bioengineered material that can stand as a future graft for urinary tract reconstruction. This study specially focuses on the fabrication techniques as well as the biofunctionalization strategies, which form the platform technology for regenerating urological tissues. The physiochemical and biological properties of materials can be tailor-made to match those of the urinary tract. The three elements of urinary tract regeneration are the cells, the scaffolds, and the physiological environment for tissue growth and survival. Classically, the approaches involve either cell-based therapy alone or implantation of a biomatrix whether or not seeded with cells.Citation9
Properties of an ideal biomaterial for urinary tract substitution
Broadly speaking, bioengineered tissues must be biocompatible, biodegradable, and well vascularized.Citation10,Citation11 Regenerated urinary tract tissues should be characterized by unique physical and biological properties to match the physiological functions of urine storage and emptying.Citation12,Citation13 Previous studies, as in bladder tissue engineering, showed that the biodegradation process reflects tissue remodeling. Eventually, if the degradation process follows the rate of regeneration within a suitable timeframe, this will result in a terminally differentiated functional urothelium.Citation14 Moreover, the material has to support adequate smooth muscle regeneration. This is best accomplished by seeding the scaffold with smooth muscle cells.Citation15 Achieving an appropriately thick muscular layer will allow the regenerated tissue to be robust, withstanding rupture if overfilled with urine. It is also of equivalent importance for effective contractions.
Structural integrity and mechanical stability are both necessary for surgical handling of tissues, as well as to avoid diverticulum formation when subjected to extreme voiding pressures. Although strength is imperative, the material must also be compliant to accommodate increasing volumes of urine during the bladder storage phase without generating high pressures.Citation16 Compliance could be adjusted by tailoring the mechanical properties of the material to simply stretch. This pliability is also critical for urethral reconstruction, in order to anastomose it freely with native tissues in a watertight fashion, ensuring a good blood supply. This is vital in penile urethral substitution, where shearing of the material could result from stress forces of erections.Citation17
Unquestionably, the material must be completely impermeable to urine, as urine is cytotoxic to the growing cells and urine leakage can subsequently lead to a devastating urinoma.Citation18 Furthermore, the surface of the material in contact with urine should resist the formation of bacterial biofilms and encrustations, avoiding long-term complications on the upper urinary tract.Citation13
and summarize the ideal properties for biomaterials substituting urinary tissues.
Figure 1 Approaches for biomaterial fabrication.
Abbreviation: 3D, three-dimensional.
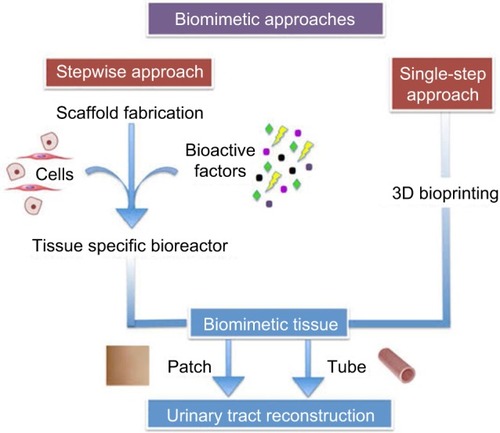
Table 2 Summary of the properties of the ideal biomaterial for urinary tract reconstruction
Biomaterials for urothelium regeneration
Biomatrix acts as a supporting backbone for tissue regeneration maintaining its structural stability and allowing its growth in a three-dimensional (3D) manner. Moreover, it should incite cellular interaction and differentiation. Biomaterials in the history of urology started with the development of urethral catheters.Citation13 They are generally classified according to their source of origin into biological, synthetic, and hybrids of both. They can also be classified according to their degradation property into biodegradable and nonbiodegradable. Lastly, they can either be cell-seeded or non-cell-seeded.Citation19
Biofabrication of the urinary tract
Important experimental and clinical applications will be discussed later in the section of biomimetic strategies.
Biological scaffolds
Natural polymers
Natural polymers such as collagen, alginate, and gelatin are mechanically weak. It is challenging to alter their mechanical properties. Therefore, their biomedical use is usually restricted as adjuncts to other materials. Nonetheless, they have shown their usefulness as inks in tissue bioprinting.Citation20,Citation21 Chitosan, on the other hand, is an advantageous polymer as it is positively charged. This positive charge is useful for the immobilization of other negatively charged polymers such as gelatin and collagen as well as some growth factors. This immobilization property can aid in controlling the stability of the regenerative process.Citation22
Biological scaffolds such as bladder acellular matrix (BAM),Citation23–Citation27 small intestinal submucosa (SIS),Citation28,Citation29 acellular amniotic membrane,Citation30 and acellular corpus spongiosum (ACSM)Citation31 have been previously experimented primarily because they mimic the native extracellular matrix (ECM). However, research is still ongoing to optimize their use for urological reconstruction.Citation32 They also harbor various growth factors and structural proteins such as glycosaminoglycan, collagen type 1, and elastin. Therefore, their mechanical properties are almost matching with those of the innate tracts. A studyCitation34 that involved testing the mechanical behaviors of SIS, BAM, ACSM, and polyglycolic acid (PGA), comparing them to rabbit urethra as control, showed that there was no significant difference in terms of Young’s modulus or stress at break between the SIS and BAM grafts. However, when it tested a four-layer graft, it showed better properties than one-layer graft (P<0.05). Therefore, it was concluded that SIS or BAM had higher strain-at-break measurements than ACSM and PGA (97.83%, 93.33% vs 53.30%, 39.70%), which were closer to normal rabbit urethra (173.67%).Citation33
In vivo studies demonstrated evident growth of urothelium and smooth muscle cells over areas of defect when using cell-seeded biological scaffolds. In the non-cell-seeded approach, smooth muscle regeneration did not exceed 30% of the scaffold surface area. Hence, it was deemed unsuitable for long defects.Citation15
Acellular scaffolds have inherent limitations, most importantly their potential of eliciting cytotoxic immune reactions. This could be explained by the presence of residual cellular elements that remain even after extensive decellularization. Subsequently, the resulting fibrosis will complicate the implantation process. Also, the long processing time, together with the limited supply of these materials, further emphasizes that they are not the best option for urinary tract substitution. Although extremely rare, a risk of infection with prion viruses might occur, specifically from materials of xenogeneic origin, such as porcine SIS.Citation17
Silk fibroin (SF)
SF is naturally derived from Bombyx mori cocoons. It is an evolving material for use in urinary reconstructive procedures. It has excellent inherent biocompatibility with less immunogenic and inflammatory reactions, compared to other biological materials. The mechanical properties are superior in terms of elasticity and stability of its shape.Citation34 SF can also be electrospinned turning it to a highly porous scaffold. This unique feature is desired for cell-seeded approaches. Seeding SF with diverse cell types was successful in various preclinical urological studies.Citation35–Citation39 Electrospinned SF showed perfect biological and mechanical tissue performance when used in augmenting the bladder of a rabbit model. It was efficient at generating functional smooth muscle regeneration compared to BAM.Citation40
Electrospinned SF was also useful in promoting nerve regeneration. Nerve guidance conduits made from silk fibroin and poly(lactic-co-glycolic acid (PLGA) through electrospinning and weaving were able to promote and maintain Schwan cell growth in vivo in rabbits.Citation41 This could be applied in the near future as a biomaterial to promote nerve tissue regeneration for bladder or ureteric engineering. The aim was to have a functional tissue for bladder contractions or ureteric peristalsis.
In a recent urethral study,Citation39 acellular bilayered silk fibroin was used as an onlay patch in a rabbit urethral model. It was capable of supporting urethral regeneration similar to conventional SIS scaffolds, but with much less immunogenicity.Citation39
Synthetic polymers
Synthetic polymers include polycaprolactone (PCL), polylactic acid (PLA), PGA, PLGA, and polyurethane (PU). Since their Food and Drug Administration (FDA) approval, they were extensively tested in various areas of biomedicine.Citation11
They have myriads of advantages, making them theoretically superior for urinary tract applications. Their supply is limitless. Furthermore, porosity, ultrastructure and surface area to volume, elasticity, and degradation can be well handled to match the original tissues. Nevertheless, synthetic polymers do lack biological recognition. Unlike biological materials, they do not possess inherent growth factors and proteins mandatory for cellular interactions.Citation19
Biofabrication of the urinary tract
ECM consists of various proteins, most importantly collagen, elastin, and glycosaminoglycan, ligands, and growth factors. They provide the structural support and tensile strength, as well as cellular adhesions and signaling.Citation42,Citation43 Mimicking urinary ECM can be achieved by modifying and assembling the biomatrix structure at the micro or nano scale. This changes its physical and biological behaviors to adapt to the urinary environment.
Strategies to match the physical properties
Hybrid biomaterials
Combining synthetic and naturally derived or natural biomaterials to manufacture a hybrid material is well known for utilizing the desirable properties of each. Biological scaffolds as BAM will enhance the stretchability of the graft, as it is rich in collagen type 4. Synthetic polymers, on the other hand, will maintain the mainframe structure of the tissue. A contemporary limitation in blending different materials is the difference in their structure chemistry. However, chemical modifications to conjugate them together were successful but potentially altered their biological behavior. Manufacturing techniques, such as electrospinning, have been successful in mechanically stabilizing hybrid polymers, which in turn supported the growth of different cell types. Electrospinned PLGA fibers were efficaciously mixed with BAM to support the growth and differentiation of bladder smooth muscle cells.Citation44 In an experimental study involving,Citation45 a hybrid material composed of equal amounts of PLGA and chitosan showed similar mechanical results to SIS controls. Moreover, canine smooth muscle viability was superior to the controls.Citation45
Modifiable scaffolds
Recently, nanostructured titanium dioxide (TiO2) scaffolds were tested as new nanocomposites for possible urological applications. TiO2 scaffolds of different shapes such as tubes, wires, spheres, and porous membranes resulted in a terminally differentiated multilayered urothelium 3 weeks after seeding with porcine urothelial cells. These results highlighted their ability to adapt to different contours without changing their biocompatibility. TiO2 scaffolds represent a good nominee for future widespread applications in urinary reconstruction.Citation46
Strategies to match the biological properties
Topographical modifications
Surface roughness
The surface topography of scaffolds is crucial for cellular growth and differentiation.Citation47,Citation48 Synthetic nanocomposites possess the advantage of tailoring their structure at the nano and submicron scales. Increasing polymer surface roughness improves its attachment to cellular proteins, a necessary affair for urothelial cell adhesion.Citation47 Poly-carbonate-urea-urethane was treated in an experimental studyCitation50 to increase its surface roughness by adding carbon nanotubes. This resulted in an increased adsorption of fibronectin without altering the surface chemistry of the polymer.Citation49
An alternative approach to modify material roughness is chemical modification of the polymer fibers by alkaline hydrolysis. This modification also improves polymer biocompatibility.Citation50 However, chemical etching using sodium hydroxide hydrolysis is associated with change in the chemical composition and architectural orientation of synthetic polymer fibers. Therefore, the mechanical properties will change accordingly.Citation50,Citation51 For that reason, additive manufacturing (AM), which will be discussed in the next section, has the advantage of controlling the material surface roughness without chemical processing. In a recent bone tissue-engineering study,Citation52 AM-guided 3D selective laser melting of the polymer has produced the optimal surface roughness for cell adhesion. The material morphology and visual observations were assessed using scanning electron microscopy and 3D micro computed tomography (CT).Citation52
Pore geometry
A morphological feature of utmost importance for cell seeding is pore geometry. Optimum pore size, number, and interconnectivity are essential for the regenerating tissues to endure. The presence of pores provides a pathway for the diffusion of nutrients to the cells as well as for the elimination of unnecessary metabolic wastes.Citation53,Citation54 Cellular attachment and proliferation require pore sizes ranging between 100 and 400 μm and should involve at least 75% of the scaffold surface area.Citation55 Various methods were described in the literature to produce a 3D porous scaffold. Conventional fabrication techniques for porosity of the scaffold such as salt leaching, gas forming, phase separation, and freeze-drying cannot control the microarchitecture of the scaffold. This issue could be solved using a computer-assisted AM approach.Citation56
Relation between surface topography and stone deposition
A chief concern for using synthetic polymers in urological reconstruction is their exposure to urine, as the bladder is the main reservoir of urine. Therefore, the urine transit time is at its maximum. In some metabolic conditions, such as hypercalcuria and oxaluria, the calcium and oxalate crystal supersaturation permits nucleation of stone crystals. Consequently, it was imperative to study the relation between the topography of scaffolds and stone deposition. Chun et alCitation58 studied the effects of nano-rough surface and the presence of submicron pores of PLGA and PU polymers on both urothelial cell adhesion and calcium oxalate deposition. An increased urothelial cell density was evident in the highly porous scaffold compared to nonporous ones. In addition, higher amounts of both calcium and oxalate crystals were deposited into submicron pores of the nano-rough PU inhibiting its crystallization in solution. The amount of calcium adsorbed on the submicron PU pores was four times higher than that on conventional PU. The authors concluded that submicron porous nano-rough PU prevented calcium oxalate crystal nucleation and formation.Citation57
Influence of fabrication techniques on scaffold topography
Conventional methods
Several classic assembly techniques aim to modify synthetic polymer fibers for optimum cell anchoring, which include electrospinning (ES), thermally induced phase separation, self-assembly, and wet spinning. ES is the most widely used technique. Unfortunately, it lays down smaller fibers with haphazardly arranged pores, not in favor of cell penetration. However, the morphology can be better optimized when ES is combined with other techniques like AM.Citation58
AM
Unlike conventional techniques, AM-3D printing-rapid prototyping controls scaffold microarchitecture with greatest precision.Citation59 AM builds scaffold layers from ground level. It also uses a variety of raw materials to customize the scaffold architecture. The process is assisted with the aid of a computer software (computer-aided design [CAD]) for prototyping models aiming to mimic the original structure of the tissue or organ. Implementation of computer-designed models to direct scaffold fabrication is referred to as computer-assisted tissue engineering. It is a groundbreaking tool recently introduced to biomaterial designing technology. Several AM methods are available now on the market, which include fused deposition modeling, selective laser sintering, stereolithography, precision extrusion deposition, and 3D printing.Citation60,Citation61
Hybrid AM combines AM techniques with conventional methods, most commonly ES. It aims to integrate materials at the assembly or technique levels and, accordingly, gain a dual benefit.Citation62 Hybrid AM in the field of bone and cartilage tissue engineering successfully manufactured 3D hierarchical structures with high porosity.Citation63–Citation66 Subsequently, it was extended even further to involve the field of soft tissue engineering such as skin, vascular, and nerve regeneration.Citation67–Citation69
Although AM approaches are superior in designing and optimizing polymer architecture, the range of suitable biomaterials available for processing is limited with respect to soft tissue engineering. For example, hydrogels are attractive materials for soft tissues, but their processing and maintaining their 3D integrity are challenging with several AM techniques.Citation70
Incorporation of bioactive molecules into the scaffold
Several studies used exogenous trophic factors to biofunctionalize scaffolds, particularly in bladder tissue engineering.Citation71–Citation73 Bioactive factors are categorized into 1) natural ECM proteins (GAGs) such as collagen, laminin, and fibronectin; 2) short peptide sequences such as RGD and YIGSR; 3) growth factors such as vascular endothelial growth factor (VEGF), insulin-like growth factor 1 (IGF-1), basic fibroblast growth factor (bFGF), platelet-derived growth factor (PDGF), and hyaluronic acid (HA); and 4) synthetic molecules. Loading scaffolds with bioactive agents creates a microenvironment simulating ECM. This environment allows cells to grow, interact, and differentiate into complex tissues, specifically urothelial, smooth muscle, vascular, and nerve tissues.Citation74,Citation76 Bioactive molecules can be incorporated into the bulk of the polymer either during synthesis or by surface conjugation.
Growth factors
Basic fibroblast growth factor (bFGF) was chemically cross-linked to BAM to control its release. FGF promoted human fibroblast proliferation after implantation to a rat bladder reconstruction model.Citation76 The PLGA nanoparticles were used as delivery agents loaded with HA to modify porcine SIS scaffolds for bladder augmentation in Beagle dogs. In that study, full thickness regeneration was noted in all canine models.Citation77 Recently, a studyCitation77 used PLGA-loaded nanoparticles to deliver VEGF onto BAM to augment bladder in rabbits. The microvessel density in the experimental group was significantly higher compared to the controls. Moreover, shrinkage of the graft decreased by 27%.Citation78
IGF-1 enhances smooth muscle regeneration. A recombinant variant was immobilized within the polymerization process of a 3D fibrin matrix. This was effective in inducing smooth muscle proliferation both in vitro and in vivo.Citation79 VEGF is also a growth molecule that recruits and enhances proliferation and survival of endothelial cells, promoting vasculogenesis.Citation80 VEGF was delivered on its own or combined with other growth molecules to miscellaneous scaffolds for bladder augmentation in animal models. The histological outcome was appealing in terms of enhanced vessel, nerve, urothelial, and smooth muscle cell regeneration. The functional outcome was also promising as there was a marked increase in bladder capacity and compliance.Citation78,Citation81–Citation83
Combining different recombinant growth factors produces a positive synergistic effect on bladder tissue regeneration. Yang et alCitation83 compared in vitro the biological effects of eight different growth factors on proliferation, migration, and wound healing of three cells. The results indicated that PDGF, epidermal growth factor, and VEGF were the most exciting growth factors for improving human bladder smooth muscle, urothelial, and vascular endothelial cell activities. Those findings proposed their potential use for bladder regeneration and angiogenesis.Citation84 Sharma et alCitation85 bioactivated the elastomer poly(1, 8-octanediol-co-citrate) films (POCfs) with heparan sulfate, generating a heparin-binding POCf (HBPOCf), subsequently liberating proangiogenic factors such as VEGF, FGF2, and IGF-1 from HBPOCf. The in vitro analysis, after ~1 month, demonstrated topographical modifications together with a threefold increase in vessel density compared to the controls. The in vivo studies were conducted after subcutaneous implantation of the biofuntionalized scaffolds (HBPOCf-VEGF) within the dorsa of nude rats. Angiogenesis was further confirmed by CD31 and VWF immunostaining of the implanted sites.Citation85
Short-sequence peptides
Short-sequence peptides such as RGD and YIGSR are used in regenerative medicine.Citation86 They act by attaching the cytoskeleton by integrins to the ECM immobilizing them, allowing better adhesion and growth.Citation87 RGD-modified PLGA scaffolds demonstrate a better smooth muscle cell adhesion compared with unmodified ones.Citation88
Influence of surface chemistry on cell–biomaterial interaction
Modifying the material surface biochemistry can render it receptive for cellular attachment.Citation89 Hydrophilic surfaces are preferred for better interaction with urothelial cells, which is achieved by adding functional groups onto the polymer surface. Various chemical and physical methods were used to treat the polymers in order to achieve this goal.
Uchida et alCitation89 described layer-by-layer (LbL) modification of poly(carbonate urethane) urea (PCUU) scaffold surface by adding fibronectin and gelatin layers. The modified scaffold surfaces demonstrated higher affinity with urothelial and bladder smooth muscle cells compared to uncoated PCUU.Citation90 Garcia-Garcia et alCitation91 treated the surface of poly(3-hydroxybu-tyrate-co-3-hydroxyvalerate) by sodium hydroxide hydrolysis. It resulted in functionalization of the polymer with −NH2 and −COOH groups. Covalent attachment of the YIGSR sequence increased the hydrophilicity of the polymer surface, resulting in improved adhesion and growth of porcine urothelium.Citation91 The same group expanded their work further on a different polymer, poly(3-hydroxybutyrate-co-3-hydroxyhexanoate), using sodium hydroxide hydrolysis and ethylenediamine aminolysis in aqueous and isopropanol media.Citation92
One of the main advantages of plasma gas treatment of polymers is the introduction of functional groups to its surface. However, there is a concern of changing their physio-chemical properties.Citation93,Citation94 Pandiyaraj et alCitation94 used plasma-treated thin transparent TiO2 film coated on the surface of a flexible poly(ethylene terephthalate) film. It resulted in increased hydrophilicity by adding a negatively charged group, as well as increased surface roughness. This adjustment was not cytotoxic to the cultured osteoblasts. Of interest, an antibacterial activity against Staphylococcus group of bacteria was noted.Citation95
Ultraviolet cross-linking technology uses the same principle as plasma. Although it has been the focus of several vascular and cardiac tissue-engineering studies,Citation96–Citation98 several biocompatibility and mechanical issues limited its use for soft tissue engineering.Citation99
The role of cell seeding and cell–scaffold interaction
Autologous cells
Urothelial cells are attractive for cellularizing biomatrices for bladder and urethral reconstruction. As they are autologous, there is a low risk of inducing autoimmune reactions.Citation100 Moreover, their isolation, as well as their method of culture, is well established and described liberally in the literature.Citation101,Citation102 However, their use may not be proper in some diseases.Citation103 Also, harvesting cells from patients with urothelial carcinomas carries the risk of tumor recurrence.Citation104 Moreover, Subramaniam et al highlighted that urothelial cells derived from bladders with neuromuscular dysfunction revealed poor regenerative capability when cultured in vitro.Citation105
Another alternative autologous epithelial cell that is under focus of research is tissue-engineered buccal mucosa. It is showing promising results in preclinical and clinical trials for future implication as an alternative cell source for urethral tissue engineering.Citation35,Citation106,Citation107 In a recent clinical study involving 12 patients, stricture recurrence in the anterior urethra occurred in two patients only.Citation108 However, researchers should extend the follow-up period to include a higher number of patients and consider different stricture etiologies for further trials.
Stem cells (SCs)
SC application in reconstructive urology is unveiling hopeful results.Citation109 Adult mesenchymal stem cells (MSCs) are more favorable than fetal SCs because they are not associated with teratoma formation, nor the ethical dilemma surrounding them.Citation110 They are harvested from different sources (eg, bone marrow, adipose tissue, and skeletal muscles). They have an exquisite pluripotentiality to differentiate into multiple cell lineages, including osteoblasts, chondrocytes, myocytes, adipocytes, and neurons.Citation111 Adipose-derived stem cells (ADSCs) are one of the most promising cells for urological tissue engineering, mainly due to their high abundance in and their simple isolation from patients.Citation112
Urine-derived stem cells (UDSCs), similar to MSCs, have the pluripotentiality to differentiate into several tissue types, including urinary structures. They do not necessitate aggressive techniques for sampling and are cost-effective. They also have an immune regulatory function as well as angiogenic properties.Citation113 Both MSCs and UDSCs exhibit paracrine effects by secreting various cytokines and growth factors such as hepatocyte growth factor, nerve growth factor (NGF), glial growth factor, IGF-1, and VEGF. The paracrine effects of SCs play a major role in tissue regeneration by exhibiting both antifibrotic and angiogenic effects.Citation114
Induced pluripotent stem cells (IPSCs) are genetically bred SCs and are generated from somatic cells by inducing the expression of different transcription factors. They could offer several advantages over the adult SCs while maintaining the plasticity of embryonic SCs.Citation109 They have a marvelous self-regenerative ability and also can differentiate into diverse cell lineages. They were reported successfully for the first time for their use in prostate, bladder, and ureter regenerative applications by reprograming stromal cells into an embryonic SC-like pluripotent state.Citation115
The functional aspect of the regenerated tissues, especially the urinary bladder, should not be underestimated. As previously mentioned, the most important function of the bladder is to effectively contract and empty urine. This requires proper smooth muscle and nerve regeneration. Detrusor muscle is the major functional tissue of the bladder, but has to be well innervated by sympathetic and parasympathetic nerve supply. This enables the bladder to coordinate its storage and emptying.Citation109,Citation116 UDSCs and MSCs have both shown in numerous studies that they are a well-established source for detrusor muscle engineering, and exhibit pheno-typing features similar to bladder smooth muscle cells.Citation117–Citation120 A recent study involving ADSCs with a BAM graft showed an enhanced detrusor muscle, neuronal regeneration, and increased bladder capacity in a rat bladder model.Citation24 This indicates that ADSCs are very promising in bladder regeneration.Citation24 Biofunctionalized scaffolds with NGFs and MSCs were effective in promoting nerve regeneration in bladder TE.Citation116,Citation121 Further strategies for the functional aspects will be discussed later in the section of promising strategies.
Antimicrobial surface properties
Urological diseases necessitating reconstruction, such as urethral stricture and neurogenic bladders, are chronic in nature and are associated with urine stasis. Frequently, they are complicated by urinary tract infections,Citation122 which are usually polymicrobial and multidrug resistant.Citation123–Citation125 Similar to urethral catheters and ureteric stents, colonization with virulent uropathogens on the inner surface of biomaterials forms a sturdy biofilm resistant to the action of standard antibiotics and disinfectants.Citation126,Citation127 Urea-splitting bacteria, particularly Proteus species, can deposit encrustations on the synthetic material surface.Citation128–Citation131 Those encrustations can theoretically block the lumen of tubularized synthetic scaffolds. For that reason, antibiofilm modifications are essential preventive strategies to maintain the patency of synthetic tubular grafts. Nanostructured surfaces may influence the behavior of bacteria on the surface of biomaterials.Citation132 Bacterial adherence and biofilm formation onto these surfaces vary widely according to the biological properties of the species and the nano-topographical features of the surface.Citation133,Citation134
Noble metals
Noble metals such as silver and gold have excellent inherent properties for antimicrobial applications. Biocompatibility, hydrophobicity, and slow development of resistant strains make them the best surface agents for long-term use in the urinary tract. Nevertheless, their cost-effectiveness, biodistribution profile, and possible systemic toxicities need to be addressed for future lifelong use in urinary reconstruction.Citation135
An experimental study involved surface functionalization of nylon catheters by coating them with a 100 nm layer of silver nitrate.Citation136 A sustained release of silver particles was observed at the implantation sites over 10 days. This modification prevented the biofilm formation of various gram-positive and gram-negative bacterial uropathogens, as well as Candida species. The in vivo analysis in male mice revealed that the biodistribution of silver was stable. Only 20% accumulated locally in the implantation site with <1% systemic organ distribution.Citation137 Also, functional gold nanoparticles effectively suppressed the growth of multidrug-resistant uropathogens in vitro.Citation136
Nitric oxide (NO) donors
NO is known to have an antibacterial activity by suppressing bacterial adhesion and causing dispersion of the attached biofilms.Citation138,Citation139 Slow release of NO can be accomplished by surface impregnation of NO donors into synthetic polymers. A novel study by Colletta et alCitation139 tested the possibility of using S-nitroso-N-acetylpenicillamine (SNAP)-impregnated silicone Foley catheters for the prevention of catheter-associated urinary tract infections (CAUTIs). SNAP is a synthetic NO donor able to generate NO at stable levels.Citation141 It is also able to suppress the formation of biofilms of two well-known microorganisms triggering CAUTIs and urinary device infections, Proteus mirabilis and Staphylococcus epidermidis.Citation140
Promising strategies
Tissue/organ engineering is undergoing a major shift. Smart biomaterials and organ bioprinting are promising research areas for biomedical applications. They can overcome the current limitations of materials used in soft tissue and complex organ reconstruction.
Smart polymers
Smart or stimulus-responsive biomaterials sense the changes in microenvironments, such as the pH, temperature, or mechanical stimuli, and consequently respond by changing their properties.Citation142,Citation143 In general, smart biomaterials that are clinically relevant and currently used for some medical applications are smart hydrogels, shape-memory polymers (SMPs), and stimulus-responsive nanoparticles.
SMPs are implemented in various medical applications such as devices for minimally invasive surgery, the delivery of therapeutics, and scaffolds for tissue engineering.Citation144 The earliest application of SMPs in urology was their integration in stents with thermo-expandable shape-memory properties. The stents were used to circumvent many ureteric, urethral, and prostatic gland diseases.Citation145,Citation146 Regarding regenerative medicine applications, SMPs were experimented in various vascular and bone tissue engineering/regenerative medicine studies.Citation147–Citation149 Their biocompatibility with cells and their tailored mechanical properties are promising. SMPs can respond to different stimuli by changing their shapes and dimensions; subsequently, on removal of the stimulus, they can regain their original shape.Citation150 For that reason, it is relevant to explore the concept in response to urinary stimuli. In bladder reconstruction, these polymers can be tailored to change their shape in response to bladder filling, urine pH, or temperature and subsequently regain back their original shape after emptying. For urethral applications, if used as implants in the penile urethra, they can stretch with penile erections, and then can shrink back to their original shape after detumescence.
Electroconductive polymers are also an interesting subset of smart polymers and can be very beneficial for bladder tissue regeneration. Electroactive PU polymers have shown great potentials for Schwan cell regeneration and muscle cell expression. This could help growth of detrusor and bladder nerve regeneration for effective contractions. This might be further maintained by a sort of electromagnetic stimulation.Citation116,Citation151–Citation154 Electroconductive natural polymer-based hydro-gels can compatibly be suitable for bladder bioprinting.Citation155
3D bioprinting
Tissue bioprinting involves direct printing of living tissues in a 3D manner in order to produce a complex structure simulating the original organs. Unlike other AM methods, it involves incorporation of cells, growth factors, and biomaterials simultaneously in a one-step approach.Citation156 Recently, Kang et alCitation156 reported a novel 3D bioprinting system able to fabricate human-scale tissue constructs of any shape. The integrated tissue–organ printer (ITOP) is a smart technology that involves the integration of medical imaging data from CT or magnetic resonance imaging scans to a computer software. The software analyses the anatomical defects, translating them to a 3D CAD model. A visualized motion program controls the bioprinter nozzles to dispense cell-laden hydrogels within specific areas of biodegradable polymers, such as PCL. This technique controlled several tissue micro-architectures in a precise manner resulting in uniform pore geometry. The ITOP system successfully printed the calvarial bone, ear cartilage, and skeletal muscles. Cell viability was not affected by the printing process when tested using sensitive assays. It is expected that this technology will soon be long-drawn-out to print more complex tissues and organs.Citation157
Another groupCitation157 used a computer-aided four-headed 3D printing system to design and print heterogeneous tissue/organ constructs. The system used cell-laden hydrogels and thermoplastic polymers such as PCL, PLA, and PLGA. The algorithm using the multiple-head tissue/organ building system (MtoBS) is an LbL process that is able to lay down two or more kinds of cells per gel solution. Products were well integrated mimicking original tissues both internally and externally. It was able to print external ear, kidney, and tooth with diverse tissue components.Citation158 In another study,Citation159 the 3D bioprinters utilized decellularized ECM bioinks to print tissue analogs. This technique was versatile. Different tissues such as cartilage, heart, and adipose were successfully printed. The tissue constructs demonstrated prolonged stability and cellular compatibility in all experimental assays.Citation159
The earliest application of 3D printing in the field of urology was for tissue simulation as a surgical education tool. It was also used for prototyping cancer models for correlation between imaging and histology in prostate and renal cancers.Citation160–Citation163 A recent study by Zhang et al,Citation164 which is considered the first, described 3D bioprinting of the urethra. This group succeeded in using the previously mentioned ITOP system to bioprint a composite urethral scaffold. It consisted of a PCL/PLCL-blended porous spiral scaffold together with two types of autologous cells (rabbit bladder urothelial and smooth muscle cells) dispensed in fibrin hydrogel of which a thorough in vitro assessment of the biomimetic mechanical properties and cell growth environment was carried out. The results of the study revealed that the biomaterial mechanical properties were equivalent to native rabbit urethra. Cell viability and proliferation were maintained sufficiently in the hydrogel. This study provided a strong foundation for future studies on 3D bioprinting of the urethra but has to be tested first in animal models.Citation164
Although tissue bioprinting appears ideal for tissue/organ reconstruction, its use is still experimental. The limited supply of materials, non-optimized mechanical properties, infrequent preclinical studies, time consumption, and high cost restrict its current use in laboratory research.Citation156,Citation165
Conclusion and future directions
The ultimate goal of reconstruction of urinary organs is not only anatomical but also functional. They are hollow, are elastic, and can be seeded with the similar cell types. The bladder is the most sophisticated to mimic, structurally and dynamically. On the other hand, the urethra, ureters, and neo-conduits are geometrically simpler to refashion as tubes.
Despite the enthusiasm and achievements so far, there is no “ideal biomaterial” available yet. Modification of biomaterial nanostructure will enhance its biological properties for cellular integration. Accordingly, successful tissue remodeling will be achieved. Smart polymers need to be explored even further to optimize their use in urinary reconstruction. They can be custom-made to change their shape and response when contacting urine. 3D bioprinting can further integrate sophisticated imaging techniques such as optical coherence tomography (OCT). OCT should be able to scan the depth of tissues on the ultra-molecular scale.
Overall, the field of tissue engineering is growing fast, implementing modern machinery to achieve its goal. The future might reveal definitive time-saving solutions, for example, intraoperative scanning of tissue defects with a “real time 3D bioprinting” using autologous tissues to reconstruct the diseased segments.
Acknowledgments
The authors thank Professor Anthony R Mundy and Miss Daniela E Andrich for their helpful comments and Dr Dina Okasha for proofreading the article and assisting with the figure. MME is sponsored by University of Alexandria/the Ministry of Higher Education, Government of Egypt. AdM is a coinvestigator of Engineering and Physical Sciences Research Council (EPSRC) project EP/L020904/1.
Disclosure
The authors report no conflicts of interest in this work.
References
- WoodhouseCRReconstructive urologyCurr Opin Urol200919655520072104
- SievertKDSeiboldJSchultheissDRekonstruktive Urologie im Wandel [Reconstructive urology in transition. From its origin into the all too near future]Urologe A200645Suppl 45258 German16933123
- DamaserMSSievertKDTissue engineering and regenerative medicine: bench to bedside in urology. PrefaceAdv Drug Deliv Rev201582–83vvii
- AlbersenMCartwrightRChoykePLooking forward, looking back – 10 years in urologyNat Rev Urol2014111164965525348169
- JordanGHPrinciples of tissue transfer techniques in urethral reconstructionUrol Clin North Am200229226727512371218
- BrykDJYamaguchiYZhaoLCTissue transfer techniques in reconstructive urologyKorean J Urol201556747848626175866
- TraxelEJMinevichEANohPHA review: the application of minimally invasive surgery to pediatric urology: lower urinary tract reconstructive proceduresUrology201076111512020303150
- MundyARThe future of urologyBJU Int200392433733912930411
- ZhangCMurphySVAtalaARegenerative medicine in urologySemin Pediatr Surg201423310611124994523
- O’BrienFJBiomaterials & scaffolds for tissue engineeringMater Today20111438895
- UleryBDNairLSLaurencinCTBiomedical applications of biodegradable polymersJ Polym Sci B Polym Phys2011491283286421769165
- FengCXuYMFuQZhuWDCuiLChenJEvaluation of the biocompatibility and mechanical properties of naturally derived and synthetic scaffolds for urethral reconstructionJ Biomed Mater Res A201094131732520166222
- BeikoDTKnudsenBEWattersonJDCadieuxPAReidGDenstedtJDUrinary tract biomaterialsJ Urol20041716 Pt 12438244415126872
- AtalaABauerSBSokerSYooJJRetikABTissue-engineered autologous bladders for patients needing cystoplastyLancet200636795181241124616631879
- AdamowiczJJuszczakKBajekAMorphological and uro-dynamic evaluation of urinary bladder wall regeneration: muscles guarantee contraction but not proper function – a rat model research studyTransplant Proc20124451429143422664029
- LuSHSacksMSChungSYBiaxial mechanical properties of muscle-derived cell seeded small intestinal submucosa for bladder wall reconstitutionBiomaterials200526444344915275818
- Ribeiro-FilhoLASievertKDAcellular matrix in urethral reconstructionAdv Drug Deliv Rev201582–833846
- VaeglerMMaurerSToomeyPAmendBSievertKDTissue engineering in urothelium regenerationAdv Drug Deliv Rev201582–836468
- LinHKMadihallySVPalmerBFrimbergerDFungKMKroppBPBiomatrices for bladder reconstructionAdv Drug Deliv Rev201582–834763
- YanYWangXXiongZDirect construction of a three-dimensional structure with cells and hydrogelJ Bioact Compat Polym2005203259269
- JiaJRichardsDJPollardSEngineering alginate as bioink for bioprintingActa Biomater201410104323433124998183
- ChungBIHamawyKJZinmanLNLibertinoJAThe use of bowel for ureteral replacement for complex ureteral reconstruction: long-term resultsJ Urol2006175117918316406903
- DahmsSEPiechotaHJDahiyaRLueTFTanaghoEAComposition and biomechanical properties of the bladder acellular matrix graft: comparative analysis in rat, pig and humanBr J Urol19988234114199772881
- ZheZJunDYangZBladder acellular matrix grafts seeded with adipose-derived stem cells and incubated intraperitoneally promote the regeneration of bladder smooth muscle and nerve in a rat model of bladder augmentationStem Cells Dev20162540541426863067
- LiaoWYangSSongCLiXLiYXiongYConstruction of ureteral grafts by seeding bone marrow mesenchymal stem cells and smooth muscle cells into bladder acellular matrixTransplant Proc201345273073423498814
- MengLLiaoWYangSXiongYSongCLiuLTissue-engineered tubular substitutions for urinary diversion in a rabbit modelExp Biol Med (Maywood)2016241214715626286106
- LiCLLiaoWBYangSXUrethral reconstruction using bone marrow mesenchymal stem cell- and smooth muscle cell-seeded bladder acellular matrixTransplant Proc20134593402340724182824
- ChengEYKroppBPUrologic tissue engineering with small-intestinal submucosa: potential clinical applicationsWorld J Urol2000181263010766040
- LinHKGodiwallaSYPalmerBUnderstanding roles of porcine small intestinal submucosa in urinary bladder regeneration: identification of variable regenerative characteristics of small intestinal submucosaTissue Eng Part B Rev2014201738323777420
- WangFLiuTYangLUrethral reconstruction with tissue-engineered human amniotic scaffold in rabbit urethral injury modelsMed Sci Monit2014202430243825424000
- FengCXuYMFuQZhuWDCuiLReconstruction of three-dimensional neourethra using lingual keratinocytes and corporal smooth muscle cells seeded acellular corporal spongiosumTissue Eng Part A20111723–243011301921736450
- SongLMurphySVYangBXuYZhangYAtalaABladder acellular matrix and its application in bladder augmentationTissue Eng Part B Rev201420216317223895225
- FengCXuYMFuQZhuWDCuiLChenJEvaluation of the biocompatibility and mechanical properties of naturally derived and synthetic scaffolds for urethral reconstructionJ Biomed Mater Res A201094131732520166222
- SackBSMauneyJREstradaCRJrSilk fibroin scaffolds for urologic tissue engineeringCurr Urol Rep20161721626801192
- XieMXuYSongLWangJLvXZhangYTissue-engineered buccal mucosa using silk fibroin matrices for urethral reconstruction in a canine modelJ Surg Res201418811724411303
- WangYRudymDDWalshAIn vivo degradation of three-dimensional silk fibroin scaffoldsBiomaterials20082924–253415342818502501
- KimUJParkJKimHJWadaMKaplanDLThree-dimensional aqueous-derived biomaterial scaffolds from silk fibroinBiomaterials200526152775278515585282
- TuDDChungYGGilESBladder tissue regeneration using acellular bi-layer silk scaffolds in a large animal model of augmentation cystoplastyBiomaterials201334348681868923953839
- ChungYGTuDFranckDAcellular bi-layer silk fibroin scaffolds support tissue regeneration in a rabbit model of onlay urethroplastyPLoS One201493e9159224632740
- HuangJWXuYMLiZBTissue performance of bladder following stretched electrospun silk fibroin matrix and bladder acellular matrix implantation in a rabbit modelJ Biomed Mater Res A2016104191626148477
- WangYLGuXMKongYFengQLYangYMElectrospun and woven silk fibroin/poly(lactic-co-glycolic acid) nerve guidance conduits for repairing peripheral nerve injuryNeural Regen Res201510101635164226692862
- AitkenKJBagliDJThe bladder extracellular matrix. Part I: architecture, development and diseaseNat Rev Urol200961159661119890339
- AitkenKJBagliDJThe bladder extracellular matrix. Part II: regenerative applicationsNat Rev Urol200961161262119890340
- HorstMMadduriSMilleretVSulserTGobetREberliDA bilayered hybrid microfibrous PLGA–acellular matrix scaffold for hollow organ tissue engineeringBiomaterials20133451537154523177021
- LawrenceBJMaaseELLinHKMadihallySVMultilayer composite scaffolds with mechanical properties similar to small intestinal submucosaJ Biomed Mater Res A200988363464318314898
- ImaniRPazokiMZupancicDBiocompatibility of different nanostructured TiO2 scaffolds and their potential for urologic applicationsProtoplasma20152531439144726497540
- ChunYWLimHWebsterTJHaberstrohKMNanostructured bladder tissue replacementsWiley Interdiscip Rev Nanomed Nanobiotechnol20113213414520730887
- YangYLeongKWNanoscale surfacing for regenerative medicineWiley Interdiscip Rev Nanomed Nanobiotechnol20102547849520803682
- KhangDLuJYaoCHaberstrohKMWebsterTJThe role of nanometer and sub-micron surface features on vascular and bone cell adhesion on titaniumBiomaterials200829897098318096222
- GaoJNiklasonLLangerRSurface hydrolysis of poly(glycolic acid) meshes increases the seeding density of vascular smooth muscle cellsJ Biomed Mater Res19984234174249788505
- YangXZhaoKChenGQEffect of surface treatment on the biocompatibility of microbial polyhydroxyalkanoatesBiomaterials20022351391139711804295
- MazzoliAFerrettiCGiganteASalvoliniEMattioli-BelmonteMSelective laser sintering manufacturing of polycaprolactone bone scaffolds for applications in bone tissue engineeringRapid Prototyp J2015214386392
- BrehmerBRohrmannDBeckerCRauGJakseGDifferent types of scaffolds for reconstruction of the urinary tract by tissue engineeringUrol Int2007781232917192728
- MurphyCMDuffyGPSchindelerAO’BrienFJEffect of collagen-glycosaminoglycan scaffold pore size on matrix mineralization and cellular behavior in different cell typesJ Biomed Mater Res A201610429130426386362
- DoratiRColonnaCTomasiCGentaIBruniGContiBDesign of 3D scaffolds for tissue engineering testing a tough polylactide-based graft copolymerMater Sci Eng C Mater Biol Appl20143413013924268242
- LohQLChoongCThree-dimensional scaffolds for tissue engineering applications: role of porosity and pore sizeTissue Eng Part B Rev201319648550223672709
- ChunYWKhangDHaberstrohKMWebsterTJThe role of polymer nanosurface roughness and submicron pores in improving bladder urothelial cell density and inhibiting calcium oxalate stone formationNanotechnology200920808510419417440
- Rnjak-KovacinaJWeissASIncreasing the pore size of electrospun scaffoldsTissue Eng Part B Rev201117536537221815802
- PuppiDZhangXYangLChielliniFSunXChielliniENano/microfibrous polymeric constructs loaded with bioactive agents and designed for tissue engineering applications: a reviewJ Biomed Mater Res B Appl Biomater201410271562157924678016
- GiannitelliSMAccotoDTrombettaMRainerACurrent trends in the design of scaffolds for computer-aided tissue engineeringActa Biomater201410258059424184176
- MelchelsFPWDomingosMANKleinTJMaldaJBartoloPJHutmacherDWAdditive manufacturing of tissues and organsProg Polym Sci201237810791104
- GiannitelliSMMozeticPTrombettaMRainerACombined additive manufacturing approaches in tissue engineeringActa Biomater20152411126134665
- DorjBParkJ-HKimH-WRobocasting chitosan/nanobioactive glass dual-pore structured scaffolds for bone engineeringMater Lett201273119122
- ZhuNLiMGCooperDChenXBDevelopment of novel hybrid poly(L-lactide)/chitosan scaffolds using the rapid freeze prototyping techniqueBiofabrication20113303410521725153
- KimMSKimGElectrohydrodynamic jet process for pore-structure-controlled 3D fibrous architecture as a tissue regenerative material: fabrication and cellular activitiesLangmuir201430288551855724971728
- KimMKimGPhysical and biological activities of newly designed, macro-pore-structure-controlled 3D fibrous poly(ε-caprolactone)/hydroxyapatite composite scaffoldsRSC Adv20155342695426964
- FarrugiaBLBrownTDUptonZHutmacherDWDaltonPDDargavilleTRDermal fibroblast infiltration of poly(ε-caprolactone) scaffolds fabricated by melt electrospinning in a direct writing modeBiofabrication20135202500123443534
- BrownTDSlotoschAThibaudeauLDesign and fabrication of tubular scaffolds via direct writing in a melt electrospinning modeBiointerphases20127111622589044
- BrownTDDaltonPDHutmacherDWDirect writing by way of melt electrospinningAdv Mater201123475651565722095922
- BillietTVandenhauteMSchelfhoutJVan VlierbergheSDubruelPA review of trends and limitations in hydrogel-rapid prototyping for tissue engineeringBiomaterials201233266020604122681979
- MauneyJRAdamRMDynamic reciprocity in cell-scaffold interactionsAdv Drug Deliv Rev201582–837785
- KanematsuAYamamotoSNoguchiTOzekiMTabataYOgawaOBladder regeneration by bladder acellular matrix combined with sustained release of exogenous growth factorJ Urol20031701633163814501679
- MondalekFGAshleyRARothCCEnhanced angiogenesis of modified porcine small intestinal submucosa with hyaluronic acid-poly(lactide-co-glycolide) nanoparticles: from fabrication to preclinical validationJ Biomed Mater Res2010A 94712719
- RiceJJMartinoMMDe LaporteLTortelliFBriquezPSHubbellJAEngineering the regenerative microenvironment with biomaterialsAdv Healthc Mater201321577123184739
- SharmaAKChengEYGrowth factor and small molecule influence on urological tissue regeneration utilizing cell seeded scaffoldsAdv Drug Deliv Rev201582–838692
- ChenWShiCHouXZhangWLiLBladder acellular matrix conjugated with basic fibroblast growth factor for bladder regenerationTissue Eng Part A20142015–162234224224483233
- RothCCMondalekFGKibarYBladder regeneration in a canine model using hyaluronic acid-poly(lactic-co-glycolic-acid) nanoparticle modified porcine small intestinal submucosaBJU Int2011108114815520942834
- JiangXXiongQXuGVEGF-loaded nanoparticle-modified BAMAs enhance angiogenesis and inhibit graft shrinkage in tissue-engineered bladderAnn Biomed Eng201543102577258625711152
- LorentzKMYangLFreyPHubbellJAEngineered insulin-like growth factor-1 for improved smooth muscle regenerationBiomateri-als2012332494503
- BauerSMBauerRJLiuZJChenHGoldsteinLVelazquezOCVascular endothelial growth factor-C promotes vasculogenesis, angiogenesis, and collagen constriction in three-dimensional collagen gelsJ Vasc Surg200541469970715874936
- KikunoNKawamotoKHirataHNerve growth factor combined with vascular endothelial growth factor enhances regeneration of bladder acellular matrix graft in spinal cord injury-induced neurogenic rat bladderBJU Int2009103101424142818990142
- YoussifMShiinaHUrakamiSEffect of vascular endothelial growth factor on regeneration of bladder acellular matrix graft: histologic and functional evaluationUrology200566120120715992890
- XiongQLinHHuaXA nanomedicine approach to effectively inhibit contracture during bladder acellular matrix allograft-induced bladder regeneration by sustained delivery of vascular endothelial growth factorTissue Eng Part A2015211–2455224947133
- YangBZhouLPengBSunZDaiYZhengJIn vitro comparative evaluation of recombinant growth factors for tissue engineering of bladder in patients with neurogenic bladderJ Surg Res20141861637224095026
- SharmaAKBuryMIFullerNJGrowth factor release from a chemically modified elastomeric poly(1,8-octanediol-co-citrate) thin film promotes angiogenesis in vivoJ Biomed Mater Res A2012100356157022162300
- StorrieHGulerMOAbu-AmaraSNSupramolecular crafting of cell adhesionBiomaterials200728314608461817662383
- PierschbacherMDRuoslahtiECell attachment activity of fibronectin can be duplicated by small synthetic fragments of the moleculeNature1984309596330336325925
- HarringtonDAChengEYGulerMOBranched peptide-amphiphiles as self-assembling coatings for tissue engineering scaffoldsJ Biomed Mater Res A200678115716716619254
- KearnsVRMcMurrayRJDalbyMJBiomaterial surface topography to control cellular response: technologies, cell behaviour and biomedical applicationsWilliamsRSurface Modification of BiomaterialsWoodhead Publishing2011169201
- UchidaNSivaramanSAmorosoNJNanometersized extracellular matrix coating on polymer-based scaffold for tissue engineering applicationsJ Biomed Mater Res A20161049410326194176
- Garcia-GarciaJMLopezLParisRSurface modification of poly(3-hydroxybutyrate-co-3-hydroxyvalerate) copolymer films for promoting interaction with bladder urothelial cellsJ Biomed Mater Res A2012100171721972181
- Garcia-GarciaJMQuijada-GarridoILopezLThe surface modification of poly(3-hydroxybutyrate-co-3-hydroxyhexanoate) copolymers to improve the attachment of urothelial cellsMater Sci Eng C Mater Biol Appl201333136236925428082
- ChuPKChenJYWangLPHuangNPlasma-surface modification of biomaterialsMater Sci Eng R Rep2002365–6143206
- YoshidaSHagiwaraKHasebeTHottaASurface modification of polymers by plasma treatments for the enhancement of biocompatibility and controlled drug releaseSurf Coat Technol201323399107
- PandiyarajKNDeshmukhRRMahendiranRInfluence of operating parameters on surface properties of RF glow discharge oxygen plasma treated TiO2/PET film for biomedical applicationMater Sci Eng C Mater Biol Appl20143630931924433917
- DeLongSAMoonJJWestJLCovalently immobilized gradients of bFGF on hydrogel scaffolds for directed cell migrationBiomaterials200526163227323415603817
- MastersKSShahDNWalkerGLeinwandLAAnsethKSDesigning scaffolds for valvular interstitial cells: cell adhesion and function on naturally derived materialsJ Biomed Mater Res A200471117218015368267
- GobinASWestJLVal-ala-pro-gly, an elastin-derived non-integrin ligand: smooth muscle cell adhesion and specificityJ Biomed Mater Res A200367125525914517884
- BaroliBPhotopolymerization of biomaterials: issues and potentialities in drug delivery, tissue engineering, and cell encapsulation applicationsJ Chem Technol Biotechnol2006814491499
- ZakrzewskiJLvan den BrinkMRHubbellJAOvercoming immunological barriers in regenerative medicineNat Biotechnol201432878679425093888
- ZhangYAtalaAUrothelial cell culture: stratified urothelial sheet and three-dimensional growth of urothelial structureMethods Mol Biol201394538339923097119
- KloskowskiTUzarskaMGurtowskaNHow to isolate urothelial cells? Comparison of four different methods and literature reviewHum Cell2014272859324368576
- BasuJJayoMJIlaganRMRegeneration of native-like neo-urinary tissue from nonbladder cell sourcesTissue Eng A2012189–1010251034
- DrewaTAdamowiczJSharmaATissue engineering for the oncologic urinary bladderNat Rev Urol201291056157222907387
- SubramaniamRHinleyJStahlschmidtJSouthgateJTissue engineering potential of urothelial cells from diseased bladdersJ Urol201118652014202021944117
- OsmanNIHillaryCBullockAJMacNeilSChappleCRTissue engineered buccal mucosa for urethroplasty: progress and future directionsAdv Drug Deliv Rev201582–836976
- MikamiHKuwaharaGNakamuraNYamatoMTanakaMKodamaSTwo-layer tissue engineered urethra using oral epithelial and muscle derived cellsJ Urol201218751882188922439965
- BarbagliGRam LiebigGFahlenkampDLazzeriMNew bulbar urethroplasty using tissue-engineered oral mucosal graft: a preliminary clinical reportJ Urol20131894 Supple1
- MousaNAAbou-TalebHAOrabiHStem cell applications for pathologies of the urinary bladderWorld J Stem Cells20157581582226131312
- StoltzJFde IslaNLiYPStem cells and regenerative medicine: myth or reality of the 21th centuryStem Cells Int2015201573473126300923
- JiangYJahagirdarBNReinhardtRLPluripotency of mesenchymal stem cells derived from adult marrowNature20024186893414912077603
- SterodimasAde FariaJNicarettaBPitanguyITissue engineering with adipose-derived stem cells (ADSCs): current and future applicationsJ Plast Reconstr Aesthet Surg201063111886189219969517
- BharadwajSLiuGShiYMultipotential differentiation of human urine-derived stem cells: potential for therapeutic applications in urologyStem Cells20133191840185623666768
- ZhangDWeiGLiPZhouXZhangYUrine-derived stem cells: a novel and versatile progenitor source for cell-based therapy and regenerative medicineGenes Dis20141181725411659
- MoadMPalDHepburnACA novel model of urinary tract differentiation, tissue regeneration, and disease: reprogramming human prostate and bladder cells into induced pluripotent stem cellsEur Urol201364575376123582880
- SmolarJSalemiSHorstMSulserTEberliDStem cells in functional bladder engineeringTransfus Med Hemother201643532833527781020
- LangRLiuGShiYSelf-renewal and differentiation capacity of urine-derived stem cells after urine preservation for 24 hoursPLoS One201381e5398023349776
- ZhangYMcNeillETianHUrine derived cells are a potential source for urological tissue reconstructionJ Urol200818052226223318804817
- BodinABharadwajSWuSGatenholmPAtalaAZhangYTissue-engineered conduit using urine-derived stem cells seeded bacterial cellulose polymer in urinary reconstruction and diversionBiomaterials201031348889890120800278
- KanematsuAYamamotoSIwai-KanaiEInduction of smooth muscle cell-like phenotype in marrow-derived cells among regenerating urinary bladder smooth muscle cellsAm J Pathol2005166256557315681839
- HuJTianLPrabhakaranMDingXRamakrishnaSFabrication of nerve growth factor encapsulated aligned poly(ε-caprolactone) nanofibers and their assessment as a potential neural tissue engineering scaffoldPolymers20168254
- HeynsCFUrinary tract infection associated with conditions causing urinary tract obstruction and stasis, excluding urolithiasis and neuropathic bladderWorld J Urol2012301778321720861
- Mohammad-JafariHSaffarMJNemateISaffarHKhalilianARIncreasing antibiotic resistance among uropathogens isolated during years 2006–2009: impact on the empirical managementInt Braz J Urol2012381253222397779
- ZowawiHMHarrisPNRobertsMJThe emerging threat of multidrug-resistant Gram-negative bacteria in urologyNat Rev Urol2015121057058426334085
- ShameliKAhmadMBZargarMYunusWMRustaiyanAIbrahimNASynthesis of silver nanoparticles in montmorillonite and their antibacterial behaviorInt J Nanomedicine2011658159021674015
- SticklerDJClinical complications of urinary catheters caused by crystalline biofilms: something needs to be doneJ Intern Med2014276212012924635559
- HoibyNCiofuOJohansenHKThe clinical impact of bacterial biofilmsInt J Oral Sci201132556521485309
- SchafferJNPearsonMMProteus mirabilis and urinary tract infectionsMicrobiol Spectr201535
- SighinolfiMCSighinolfiGPGalliEChemical and mineralogical analysis of ureteral stent encrustation and associated risk factorsUrology201586470370626297602
- BouzidiHTraxerODoreBCaractéristiques des incrustations des endoprothèses urétérales chez les patients lithiasiques [Characteristics of encrustation of ureteric stents in patients with urinary stones]Prog Urol2008184230237 French18501303
- BithelisGBouropoulosNLiatsikosENPerimenisPKoutsoukosPGBarbaliasGAAssessment of encrustations on polyurethane ureteral stentsJ Endourol200418655055615333220
- AnselmeKDavidsonPPopaAMGiazzonMLileyMPlouxLThe interaction of cells and bacteria with surfaces structured at the nanometre scaleActa Biomater20106103824384620371386
- GarrettTRBhakooMZhangZBacterial adhesion and biofilms on surfacesProg Nat Sci200818910491056
- HoriKMatsumotoSBacterial adhesion: from mechanism to controlBiochem Eng J2010483424434
- RaiMIngleAPGuptaIBrandelliABioactivity of noble metal nanoparticles decorated with biopolymers and their application in drug deliveryInt J Pharm2015496215917226520406
- LiXRobinsonSMGuptaAFunctional gold nanoparticles as potent antimicrobial agents against multi-drug-resistant bacteriaACS Nano2014810106821068625232643
- RoeDKarandikarBBonn-SavageNGibbinsBRoulletJBAntimicrobial surface functionalization of plastic catheters by silver nanoparticlesJ Antimicrob Chemother200861486987618305203
- KishikawaHEbberydARömlingUControl of pathogen growth and biofilm formation using a urinary catheter that releases antimicrobial nitrogen oxidesFree Radic Biol Med2013651257126424084579
- Regev-ShoshaniGKoMMillerCAv-GayYSlow release of nitric oxide from charged catheters and its effect on biofilm formation by Escherichia coliAntimicrob Agents Chemother201054127327919884372
- CollettaAWuJWoYS-Nitroso-N-acetylpenicillamine (SNAP) impregnated silicone foley catheters: a potential biomaterial/device to prevent catheter-associated urinary tract infectionsACS Biomater Sci Eng20151641642426462294
- de MelMuradFSeifalianAMNitric oxide: a guardian for vascular grafts?Chem Rev201111195742576721663322
- RibeiroCSencadasVCorreiaDMLanceros-MéndezSPiezoelectric polymers as biomaterials for tissue engineering applicationsColloids Surf B Biointerfaces2015136465526355812
- AguilarMRSan RománJIntroduction to smart polymers and their applicationsAguilarMRRománJSSmart Polymers and Their ApplicationsWoodhead Publishing2014111
- HardyJGPalmaMWindSJBiggsMJResponsive biomaterials: advances in materials based on shape-memory polymersAdv Mater201628275717572427120512
- KulkarniRBellamyENickel-titanium shape memory alloy Memokath 051 ureteral stent for managing long-term ureteral obstruction: 4-year experienceJ Urol200116651750175411586216
- PerryMJRoodhouseAJGidlowABSpicerTGEllisBWThermo-expandable intraprostatic stents in bladder outlet obstruction: an 8-year studyBJU Int200290321622312133055
- RickertDMosesMALendleinAKelchSFrankeRPThe importance of angiogenesis in the interaction between polymeric biomaterials and surrounding tissueClin Hemorheol Microcirc200328317518112775899
- TzonevaRSeifertBBehlMLendleinAElastic multiblock copolymers for vascular regeneration: protein adsorption and hemocompatibilityClin Hemorheol Microcirc2012522–433734822975945
- CorreiaCOLeiteAJManoJFChitosan/bioactive glass nanoparticles scaffolds with shape memory propertiesCarbohydr Polym2015123394525843832
- FuYQHuangWMLuoJKLuHPolyurethane shape-memory polymers for biomedical applicationsYahiaLShape Memory Polymers for Biomedical ApplicationsWoodhead Publishing2015167195
- HardyJCornelisonRSukhavasiRElectroactive tissue scaffolds with aligned pores as instructive platforms for biomimetic tissue engineeringBioengineering20152115
- WuYWangLGuoBShaoYMaPXElectroactive biodegradable polyurethane significantly enhanced Schwann cells myelin gene expression and neurotrophin secretion for peripheral nerve tissue engineeringBiomaterials201687183126897537
- AhyaiSASchmidMKuhlMOutcomes of ventral onlay buccal mucosa graft urethroplasty in patients after radiotherapyJ Urol2015194244144625846417
- StoltingMNArnoldASHaralampievaDHandschinCSulserTEberliDMagnetic stimulation supports muscle and nerve regeneration after trauma in miceMuscle Nerve201653459860726202157
- ShiZGaoXUllahMWLiSWangQYangGElectroconductive natural polymer-based hydrogelsBiomaterials2016111405427721086
- MurphySVAtalaA3D bioprinting of tissues and organsNat Biotechnol201432877378525093879
- KangHWLeeSJKoIKKenglaCYooJJAtalaAA 3D bioprinting system to produce human-scale tissue constructs with structural integrityNat Biotechnol20163431231926878319
- JungJWLeeJSChoDWComputer-aided multiple-head 3D printing system for printing of heterogeneous organ/tissue constructsSci Rep201662168526899876
- PatiFJangJHaDHPrinting three-dimensional tissue analogues with decellularized extracellular matrix bioinkNat Commun20145
- YoussefRFSpradlingKYoonRApplications of three-dimensional printing technology in urological practiceBJU Int201511669770226010346
- PriesterANatarajanSLeJDA system for evaluating magnetic resonance imaging of prostate cancer using patient-specific 3D printed moldsAm J Clin Exp Urol20142212713525374914
- CheungCLLooiTLendvayTSDrakeJMFarhatWAUse of 3-dimensional printing technology and silicone modeling in surgical simulation: development and face validation in pediatric laparoscopic pyeloplastyJ Surg Educ201471576276724776857
- SolimanYFeibusAHBaumN3D printing and its urologic applicationsRev Urol2015171202426028997
- ZhangKFuQYooJ3D bioprinting of urethra with PCL/PLCL blend and dual autologous cells in fibrin hydrogel: an in vitro evaluation of biomimetic mechanical property and cell growth environmentActa Biomater20175015416427940192
- WangXYanYZhangRRecent trends and challenges in complex organ manufacturingTissue Eng B Rev2010162189197
- MundyARAndrichDEUrethral stricturesBJU Int2011107162621176068
- CraigJRWallisCBrantWOHotalingJMMyersJBManagement of adults with prior failed hypospadias surgeryTransl Androl Urol20143219620426816767
- MundyARFailed hypospadias repair presenting in adultsEur Urol200649577477616564128
- AndrichDEMundyARWhat is the best technique for urethroplasty?Eur Urol20085451031104118715692
- AndrichDEMundyARNon-transecting anastomotic bulbar urethroplasty: a preliminary reportBJU Int201210971090109421933325
- KuoTLVenugopalSInmanRDChappleCRSurgical tips and tricks during urethroplasty for bulbar urethral strictures focusing on accurate localisation of the stricture: results from a tertiary centreEur Urol201567476477025578622
- LumenNVierstraete-VerlindeSOosterlinckWBuccal versus lingual mucosa graft in anterior urethroplasty: a prospective comparison of surgical outcome and donor site morbidityJ Urol201619511211726241906
- MangeraAPattersonJMChappleCRA systematic review of graft augmentation urethroplasty techniques for the treatment of anterior urethral stricturesEur Urol201159579781421353379
- LumenNOosterlinckWHoebekePUrethral reconstruction using buccal mucosa or penile skin grafts: systematic review and meta-analysisUrol Int201289438739422889835
- BarbagliGVallascianiSRomanoGFabbriFGuazzoniGLazzeriMMorbidity of oral mucosa graft harvesting from a single cheekEur Urol2010581334120106587
- WoodDNAllenSEAndrichDEGreenwellTJMundyARThe morbidity of buccal mucosal graft harvest for urethroplasty and the effect of nonclosure of the graft harvest site on postoperative painJ Urol2004172258058315247736
- BiersSMVennSNGreenwellTJThe past, present and future of augmentation cystoplastyBJU Int201210991280129322117733
- CodyJDNabiGDublinNUrinary diversion and bladder reconstruction/replacement using intestinal segments for intractable incontinence or following cystectomyCochrane Database Syst Rev20122CD003306
- LeeRKAbol-EneinHArtibaniWUrinary diversion after radical cystectomy for bladder cancer: options, patient selection, and outcomesBJU Int20141131112324330062
- GreenwellTJVennSNMundyARAugmentation cystoplastyBJU Int200188651152511678743
- SteinRKamalMMRubenwolfPZieselCSchroderAThuroffJWBladder augmentation using bowel segments (enterocystoplasty)BJU Int201211071078109422954030
- SteinRSchröderAThüroffJWBladder augmentation and urinary diversion in patients with neurogenic bladder: non-surgical considerationsJ Pediatr Urol20128214515221493159
- SteinRSchröderAThüroffJWBladder augmentation and urinary diversion in patients with neurogenic bladder: surgical considerationsJ Pediatr Urol20128215316122264521
- KnightRBHudakSJMoreyAFStrategies for open reconstruction of upper ureteral stricturesUrol Clin North Am201340335136123905933
- Ali-el-DeinBGhoneimMABridging long ureteral defects using the Yang-Monti principleJ Urol200316931074107712576849