Abstract
Intraoperative neurophysiological information could increase accuracy of surgical deep brain stimulation (DBS) lead placement. Subsequently, DBS therapy could be optimized by specifically targeting pathological activity. In Parkinson’s disease, local field potentials (LFPs) excessively synchronized in the beta band (13–35 Hz) correlate with akinetic-rigid symptoms and their response to DBS therapy, particularly low beta band suppression (13–20 Hz) and high frequency gamma facilitation (35–250 Hz). In dystonia, LFPs abnormally synchronize in the theta/alpha (4–13 Hz), beta and gamma (60–90 Hz) bands. Phasic dystonic symptoms and their response to DBS correlate with changes in theta/alpha synchronization. In essential tremor, LFPs excessively synchronize in the theta/alpha and beta bands. Adaptive DBS systems will individualize pathological characteristics of neurophysiological signals to automatically deliver therapeutic DBS pulses of specific spatial and temporal parameters.
Accurate implantation of deep brain stimulation (DBS) leads is paramount. Several preoperative and/or intraoperative imaging modalities provide increasingly precise anatomical guidance. Neurophysiological and clinical information obtained by intraoperative microelectrode recordings and stimulation could further increase accuracy.
Directional stimulation is a novel modality that requires segmented DBS leads. This modality allows a more efficient stimulation of regions associated with clinical benefits while avoiding regions associated with side effects. Directional stimulation cannot compensate for misplaced leads but it could optimize outcomes and energy consumption in patients with well-placed or slightly misplaced leads.
Continuous DBS influences normal and abnormal activity of the target circuit. Modulation of abnormal activity translates into motor symptom control but modulation of normal activity may concomitantly result in unwanted and often subtle side effects.
In Parkinson’s disease (PD), local field potentials (LFPs) that are abnormally synchronized in the beta frequency band (13–35 Hz) correlate with the severity of akinetic-rigid symptoms and their response to pharmacological and DBS therapy.
In PD, improvement of akinetic-rigid symptoms is associated with DBS suppression of abnormally synchronized LFPs in the low beta frequency band (13–20 Hz) and facilitation of high frequency gamma synchronization (35–250 Hz).
A novel and commercially available DBS system is able to measure LFPs from DBS targets using inactive contacts while delivering stimulation through active contacts. This system has the potential to reduce the time it takes to optimize DBS in PD by targeting areas with higher amplitude of low beta LFPs.
In dystonia, LFPs are abnormally synchronized in the theta/alpha (4–13 Hz), beta (13–35 Hz) and gamma (60–90 Hz) frequency bands. Phasic dystonic symptoms and their gradual response to DBS therapy correlate with changes in theta/alpha LFP synchronization.
In essential tremor, there is excessive LFP synchronization in the theta/alpha (4–13 Hz) and beta (13–35 Hz) frequency bands.
DBS therapy for movement disorders could be anatomically optimized by directional stimulation and physiologically optimized by targeting pathological LFPs. In the future, adaptive DBS systems will individualize pathological characteristics of measured neurophysiological signals to automatically deliver therapeutic electrical pulses of specific spatial and temporal parameters.
Currently available deep brain stimulation (DBS) systems continuously deliver electrical pulses of certain amplitude, duration and frequency to modulate neurophysiological activity at the circuit level. DBS is safe and effective for the treatment of motor symptoms including motor fluctuations and tremor in Parkinson’s disease (PD), essential tremor (ET) and dystonia. In these conditions, implanted DBS systems target key nodes of basal ganglia-thalamo-cortical circuits, including the sensorimotor regions of the subthalamic nucleus (STN), the globus pallidus parts interna (GPi) and the ventral intermedius (Vim) nucleus of the thalamus. The exact mechanism of action of DBS is still debated, though we can make inferences about its effects based on experience with implantation in more than 160,000 patients worldwide [Citation1].
Precise implantation of DBS leads within the target is essential to optimize benefits and avoid off-target side effects. Using segmented DBS leads, directional stimulation requires less energy and could prevent off-target side effects. This modality cannot compensate for misplaced leads but it could optimize outcomes and energy consumption in patients with well-placed or slightly misplaced leads. Once the leads are in the optimal location, continuous DBS influences both normal and abnormal activity of the target circuit depending on the specific neurophysiological signals modulated by specific pulse parameters delivered during specific timeframes. Modulation of abnormal activity translates into motor symptom control, but modulation of normal activity may concomitantly result in unwanted and often subtle side effects [Citation2]. Individualizing DBS therapy to treat the predominant motor symptoms without causing side effects may be possible by manipulating DBS pulses so that they modulate only abnormal neurophysiological activity. In this article, we review clinical applications and recent advances in our understanding of neurophysiological biomarkers to optimize the treatment of movement disorders with DBS, including PD, ET and dystonia.
Neurophysiological biomarkers & DBS
Neuronal firing patterns
Neuroanatomical structures encountered throughout the surgical trajectories toward the DBS target have characteristic electrophysiological patterns of neuronal cell firing that are present during wakefulness. At most centers, these patterns are evaluated by microelectrode recordings (MER) of extracellular, single-unit neuronal activity prior to surgical implantation of the DBS leads. Neuronal firing patterns include distinctive spike frequencies (firing rates), burst indices, amplitudes and densities of cell firing. By identifying these patterns while advancing a microelectrode through a stereotactically-defined trajectory, intraoperative MER expands the neuroanatomical resolution of pre-surgical imaging studies to optimize such trajectories and targets for final implantation of the DBS leads. Intraoperative microstimulation further expands MER-based mapping to include acute clinical responses and side effects triggered by stimulation at different depths along each trajectory. Thus, microstimulation is able to provide useful physiological information from trajectories and locations where the DBS lead may not be necessarily placed in the end. summarizes common neuronal firing patterns and stimulation-induced clinical effects that the surgical DBS team, including neurosurgeon, neurophysiologist and/or neurologist, may use to optimize the location of DBS lead implantation [Citation3,Citation4].
Table 1. Common neuronal firing patterns and stimulation-induced effects encountered during deep brain stimulation surgery for movement disorders.
Although the addition of MER to imaging-based anatomical targeting likely increases neurosurgical accuracy, the use of MER is still controversial since no prospective trial has directly compared the outcomes of DBS in patients randomized to MER-based surgery while awake versus purely imaging-based surgery while sedated [Citation5–8]. Importantly, interpretation of MER is subjective and varies due to patient characteristics, anesthetic agents, equipment used for the recordings, expertise of the neurophysiologist, etc. Additionally, ‘optimal’ placement may vary across patients, as recent imaging models and connectome-based anatomical and functional studies have identified that different locations within the target nuclei may be better suited to optimize specific motor symptoms [Citation9,Citation10]. Prospective studies correlating imaging-based neuroanatomical targets, neurophysiologically optimized targets and clinical outcomes of DBS are needed to confirm the theoretical value of MER and intraoperative stimulation.
Local field potentials
Rather than individual neuronal firing, local field potentials (LFPs) represent synchronized presynaptic and postsynaptic activity of large neuronal populations. This synchronized activity originates electrophysiological oscillations in a circumscribed region [Citation11]. LFP recordings might be equivalent to MER for DBS lead implantation [Citation12]. Furthermore, disease- and symptom-specific LFPs, reflecting different types of abnormally synchronized oscillations, have been described in the STN, GPi and Vim of patients with PD, ET and dystonia [Citation13]. Besides the specific neuroanatomical location of these LFPs in the target sensorimotor circuit, other characteristics such as the frequency, amplitude, duration and waveform shape of the LFPs appear to be relevant to optimize DBS therapy. For instance, one of the first observations in neuromodulation was that stimulation at the same thalamic target produced opposite effects in tremor control depending on the high (∼100 Hz) or low (∼10 Hz) frequency of the pulses [Citation14]. These initial observations and the widespread use of electroencephalography probably originated the current classification of LFPs based on their frequency bands: delta (0–4 Hz), theta/alpha (4–13 Hz), low beta (13–20 Hz), high beta (20–35 Hz), gamma (35–250 Hz band) and high-frequency oscillations (250–350 Hz) (). Both spatial and temporal relationships between pathological LFPs and DBS pulses may be important determinants of the clinical outcomes of DBS therapy in movement disorders [Citation15].
Table 2. Local field potentials associated with movement disorders.
Neurophysiological biomarkers to optimize DBS in PD
Intraoperative neurophysiology
In PD, neuronal firing patterns observed during MER and stimulation-induced clinical effects can help optimize imaging-based trajectories and targets (). An optimized dorsolateral STN trajectory would include at least 4–5 mm of sensorimotor activity (i.e., neuronal firing modulated by contralateral tremor or limb movement) and anatomically consistent clinical changes induced by stimulation [Citation16,Citation17]. For example, an intraoperative microstimulation distance-threshold product greater than 500 (mm × μA) may predict a postoperative amplitude threshold for capsular side effects of less than 2 V [Citation18]. An optimized ventral posterolateral GPi trajectory would be located approximately 2–3 mm anterior to the posterior limb of the internal capsule, and include sensorimotor activity and anatomically consistent clinical changes induced by stimulation. Intraoperative stimulation helps define the GPi target that is dorsal to the optic tract and appropriate to the sensorimotor homuncular representation, which would theoretically improve outcomes [Citation19–21].
Frequency matters
Experiments in which electrical pulses are delivered at frequencies of 20 Hz or less in patients with PD have demonstrated deterioration of bradykinesia [Citation22,Citation23]. Stimulation at higher frequencies, particularly in the gamma range associated with voluntary movement, has been shown to improve bradykinesia. In practice, DBS at 130 Hz or higher is effective for rigidity, tremor and bradykinesia. However, specific features of movement control could actually worsen. For instance, DBS at greater than 100 Hz has been associated with faster but less accurate movements [Citation24,Citation25]. Similarly, high frequency DBS may reduce speech fluency and intelligibility despite improvement in speech volume [Citation26,Citation27]. This apparently paradoxical phenomenon could result from continuously suppressing neurophysiological activity that is abnormal for certain features (e.g., movement speed) but normal for other features (e.g., movement control) [Citation2].
In clinical practice, low frequency DBS (usually 60–80 Hz) might be useful when patients experience axial motor dysfunction induced by DBS at higher frequencies, including postural instability, gait and speech dysfunction. Interestingly, low frequency STN-DBS has yielded better outcomes when electrical pulses are delivered anteriorly or ventrally. It is still unclear whether these benefits result from location-specific DBS effects or mitigation of DBS-induced side effects. The initially observed benefits of low frequency DBS have been short-lasting and inconsistent in some patients [Citation28,Citation29].
LFPs in the beta band frequency
In patients with PD who experience emergent motor symptoms after withholding dopaminergic medications or during real world OFF periods, LFP recordings from the STN and GPi consistently demonstrate excessively synchronized activity in the beta band frequency (13–30 Hz) [Citation30]. This beta LFP activity correlates with the presence of contralateral akinetic-rigid symptoms. Moreover, dopaminergic and DBS-induced desynchronization of this beta LFP activity correlates with symptom improvement [Citation31]. Interestingly, the beneficial effects of levodopa are associated with changes in low beta (13–20 Hz) more than high beta LFPs (21–30 Hz) [Citation32]. There is no correlation between beta LFPs and resting tremor in PD, possibly indicating different pathophysiological mechanisms involved in tremor generation and akinetic-rigid symptoms in these patients. In fact, tremor has been associated with reduction in beta activity [Citation33].
In patients with PD, there is significant correlation between the optimal therapeutic location of the DBS lead and the spatial distribution of beta LFP activity within the sensorimotor STN. Stimulation of STN regions where beta LFPs are most prominent are associated with greater improvement in akinetic-rigid symptoms [Citation34,Citation35]. Since LFPs in the beta range are measurable and consistently correlate with contralateral akinetic-rigid symptom severity and their response to DBS therapy, they are potential neurophysiological biomarkers to optimize DBS in patients with PD. It must be kept in mind that beta band LFPs are neither sensitive nor specific for PD. Even in patients with PD, beta band LFPs are attenuated by and could potentially disappear during initiation of volitional movements [Citation36]. LFPs in the beta range have been found in other conditions such as dystonia [Citation37] and obsessive-compulsive disorder [Citation38]. Furthermore, specific patterns of beta activity have been reported for certain motor activities in patients with PD [Citation39,Citation40]. Yet, the relatively greater amount of low beta activity (13–20 Hz) that correlates with akinetic-rigid symptom severity could distinguish PD from other conditions. High beta activity (20–35 Hz) correlates less with symptom severity and might result from hyperdirect cortical-subthalamic signals [Citation41].
Amplitude of beta band LFPs in PD
In addition to their characteristic beta frequency, the amplitude of LFP activity is important in PD as it also correlates with the severity of contralateral bradykinesia and rigidity. Similar to levodopa, continuous high frequency DBS reversibly attenuates the amplitude of beta band activity in the targeted motor circuit [Citation42]. DBS-induced amplitude reduction in beta band LFPs also correlates with the degree of acute and chronic response of akinetic-rigid symptoms in PD. Symptom improvement associated with levodopa correlates with amplitude attenuation of LFPs in the low beta (13–20 Hz) more than high beta range (21–30 Hz) [Citation43,Citation44].
Duration of beta band LFPs in PD
Recent studies have demonstrated that the duration of excessive beta synchronization across different motor circuit nodes may be more specific for akinetic-rigid symptoms in PD. In these studies, untreated akinetic-rigid symptoms in PD correlated with longer bursts of beta synchronization in both the STN and the GPi, whereas shorter episodes correlated with treated akinetic-rigid symptoms in PD and dystonia. Longer bursts of beta LFPs were also associated with increased local and interhemispheric synchronization that resulted in slowness of movement [Citation45–47]. Consequently, continuous DBS-induced beta suppression that does not distinguish between shorter and longer-lasting beta activity could limit its benefits by producing deleterious effects [Citation48]. Importantly, the amplitude of beta activity progressively tends to increase along with its duration.
LFPs in the gamma and other high frequency bands
Abnormal LFP synchronization in the beta range may be considered a neurophysiological marker because it is measurable and consistently correlates with Parkinsonism severity and response to treatment. In addition to changes in beta band LFPs, dopaminergic treatment and DBS have also been associated with increased activity in the gamma (60–90 Hz) and high frequency (250–350 Hz) ranges [Citation49,Citation50]. As opposed to beta oscillations, gamma and high frequency oscillations seem to be associated with movement facilitation. In fact, gamma oscillations increase synchronously in the motor cortex during normal voluntary movements [Citation24,Citation51]. After administration of levodopa, the peaks of gamma and high frequency oscillations are not attenuated but they actually shift toward higher frequencies within these ranges when symptom improvement is evident [Citation50,Citation51]. As previously mentioned, resting tremor does not reliably correlate with changes in beta band LFPs. However, a similar frequency shift from lower (∼250 Hz) toward higher (∼350 Hz) frequencies may be a reliable marker of tremor in PD [Citation49]. Interestingly, amplitude attenuation in low gamma (31–45 Hz) and high frequency oscillations have been associated with tremor reduction [Citation52,Citation53].
LFPs in the theta and alpha frequency bands
Excessively synchronized LFP activity in the 3–10 Hz range has been reported in the GPi and STN of animal models and patients with PD during levodopa-associated dyskinesia [Citation54]. Consistent with these findings, dyskinesia has been induced by stimulation of the STN at 5 Hz in patients with PD [Citation55]. Similar to what occurs during volitional movements, some studies have evidenced suppression of the beta band LFPs during levodopa-associated dyskinesia. In this case, the dopaminergic-induced increase in gamma activity evidenced in patients without dyskinesia was absent [Citation56]. Remarkably, other studies have shown an increase in gamma activity (60–90 Hz) during dyskinesia [Citation57]. In seven patients with PD and diphasic dyskinesia, STN beta activity disappeared and theta and gamma activities slightly increased at the onset of the dyskinesia [Citation56].
Hemispheric-specific biomarkers
Levodopa and other dopaminergic medications affect both cerebral hemispheres in a presumably similar (symmetric) pattern. In fact, there is strong interhemispheric coupling with local phasic synchronization and long beta bursts after levodopa withdrawal in patients with PD. After administration of levodopa, this interhemispheric synchronization decreases along with coupling of shorter bursts of beta LFPs [Citation58]. As opposed to medications, DBS offers the opportunity to differentiate the type of electrical pulses delivered to each hemisphere [Citation59]. Worsening of axial motor function, including speech and gait, has been observed despite improvement of other motor symptoms after bilateral STN-DBS in PD [Citation60,Citation61]. In a study of 16 patients with PD, beta band LFPs from the left and right STN were found to alternate with each contralateral step cycle during normal gait [Citation62]. During normal steps, the STN beta band in patients with PD and freezing of gait has smaller amplitude and is less predictable compared with patients with PD without freezing. Prolonged bursts of beta band synchronization are observed during freezing episodes [Citation48,Citation63]. Since continuous, bilateral STN-DBS might be detrimental for gait control in PD, neurophysiological information specifically obtained from each hemisphere has potential therapeutic implications. Adjusting bilateral STN-DBS in an asymmetric, lateralized, alternating or adaptive fashion according to neurophysiological signals present in each hemisphere could be used to avoid stimulation-induced side effects in patients with PD [Citation64].
Other potential neurophysiological biomarkers in PD
Magnetoencephalography and electrocorticography recordings from the motor cortex, obtained concomitantly with STN LFPs, have revealed exaggerated beta-gamma phase-amplitude coupling as a dynamic cortical marker of akinetic-rigid symptoms in PD [Citation65,Citation66]. Additionally, the specific shape of the beta LFP waveform was found to be of potential relevance [Citation67]. Similar to what occurs in STN and GPi LFPs, DBS-induced symptom improvement has also been associated with reduction in this cortical phase-amplitude coupling [Citation68]. Electrophysiological signals obtained from the motor cortex have been used to test adaptive DBS paradigms in patients with PD [Citation69]. A recent small study showed that levodopa-associated dyskinesia is associated with strongly coherent 60–90 Hz gamma oscillations in the motor cortex and the STN [Citation57].
Optimizing DBS for PD in clinical practice
Improvement in the akinetic-rigid symptoms of PD are associated with neutralizing abnormal low frequency beta synchronization and facilitating normal high frequency gamma synchronization [Citation50,Citation70]. The vast majority of initial LFP studies in PD had been performed immediately after implantation of DBS leads in patients with externalized electrode connectors. This approach limited generalizability to clinical practice, in which a fundamentally different methodology is employed to implant and use DBS systems in patients with PD. Further studies using a new implantable pulse generator (IPG) model consistently and reliably demonstrated the presence of LFPs in patients with PD who had undergone DBS surgery many years before [Citation71]. Further advances in DBS technology allowed measuring LFPs and delivering stimulation at the same time but using different contacts of the DBS lead [Citation72]. Using this novel DBS technology for longer periods allowed to replicate correlations of beta band LFPs with the severity of akinetic-rigid symptoms and their response to pharmacological and DBS therapy [Citation73,Citation74]. This novel IPG system has been recently approved and is being implemented in Europe and North America. Long-term DBS suppression of pathological beta activity could remain for as long as 12 months but it tends to attenuate over time [Citation75,Citation76]. Currently, initial DBS programming consists of monopolar testing of all lead contacts to define the therapeutic window for each contact in the dopaminergic medication OFF state. After this initial session, patients usually require multiple follow-up visits to optimize the DBS settings. Since the delivery of electrical pulses will not fundamentally change with the novel IPG system, it is likely that long-term clinical outcomes will remain similar. However, this DBS system has the potential to reduce the time it takes to reach those outcomes by targeting areas with higher amplitude of low beta LFPs ().
Conventional monopolar review based on acute clinical responses during initial DBS programming yielded equivalent therapeutic windows for contacts E0 and E1. Consistent with the monopolar review, the LFP survey demonstrated low beta (13–20 Hz) frequency peaks of higher amplitude in the regions between contacts (A) E0 and E1, (C) E0 and E2 and (D) E0 and E3. There were no significant beta peaks in the regions between contacts (B) E1 and E2, (E) E1 and E3 and (F) E2 and E3. Thus, initial DBS settings were programmed using contact E0 since the three regions with low beta LFPs shared contact E0 and the highest amplitude (1.89 μVp) was found in the region between contacts (D) E0 and E1. LFP measurements were obtained in the dopaminergic medication OFF state by the Percept DBS system (Medtronic).
DBS: Deep brain stimulation; LFP: Local field potential.
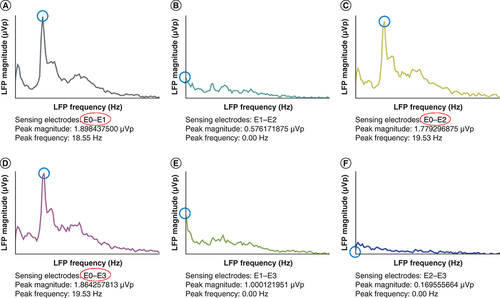
Neurophysiological biomarkers to optimize DBS in dystonia & ET
Intraoperative neurophysiology in dystonia
Neurophysiological GPi activity differs in patients with PD and dystonia [Citation19]. An optimized ventral posterolateral GPi trajectory for dystonia is located approximately 4 mm anterior to the posterior limb of the internal capsule. Similar to PD, the GPi target must be dorsal to the optic tract and implantation at the site of the appropriate sensorimotor homuncular representation would theoretically improve outcomes [Citation20,Citation21]. Similar to PD, both GPi and STN have been found to be equally effective targets for dystonia [Citation77,Citation78].
Intraoperative neurophysiology in ET
The main DBS target for the treatment of ET is the thalamic Vim nucleus. An optimized thalamic Vim DBS trajectory is located approximately 2–3 mm anterior to the tactile region of the ventral caudal nucleus of the thalamus at the appropriate homuncular representation, defined by intraoperative stimulation [Citation79]. In a recent MER study of ten patients with severe ET, the neuronal activity with the maximum power and coherence with tremor activity on electromyography (EMG) was found in the inferior part and border of the posterior ventrolateral thalamus (which partially corresponds to the Vim). In these regions, neuronal populations with efferent tremor activity were predominant and their suppression correlated with tremor reduction [Citation80].
Local field potentials in dystonia
In dystonia, GPi LFPs consistently demonstrate abnormal synchronization in the theta/alpha (4–13 Hz) frequency bands [Citation81–86]. This theta/alpha activity is more prominent in electrode contacts positioned in the GPi compared with contacts positioned in the globus pallidus externus [Citation80]. In a few studies, LFP activity was also increased in the beta (13–35 Hz) [Citation83,Citation85] and gamma band (60–90 Hz) [Citation84]. Although GPi beta oscillations were identified, statistical comparisons confirmed they were less prominent in dystonia than in PD [Citation85]. Fewer studies have evaluated LFP activity in the STN of patients with dystonia, but these trials also showed significant power spectra in the theta/alpha [Citation87], beta [Citation88] and gamma frequency ranges [Citation87].
An important question is whether the theta/alpha LFP activity correlates with clinical symptoms. Multiple studies have shown high signal coherence between low frequency pallidal LFP activity and simultaneously recorded surface EMG from the contralateral muscles [Citation81,Citation82,Citation89]; changes in EMG activity and clinical exam corresponded to phasic rather than tonic symptoms [Citation89]. In cervical dystonia patients, mean theta or alpha GPi activity correlated with symptom severity and was greatest in the side contralateral to affected muscles [Citation37]. Another study of GPi LFPs in dystonic patients performing voluntary upper extremity movements revealed desynchronization of beta 20–30 Hz signals just prior to movement, and then decreased alpha 8–12 Hz power during the movement [Citation90]. This suggests that excessive alpha synchronization could impede normal movement [Citation90,Citation91].
Similarly, GPi-DBS decreased alpha predominant signals, but the trials only evaluated changes over minutes to hours. In a study of 12 patients separated into predominantly phasic versus tonic symptoms, recorded GPi LFPs 1–3 days after DBS macroelectrode implantation revealed prominent 4–12 Hz signals for patients with both tonic and phasic dystonia [Citation81]. GPi stimulation for 150 s reduced the frequency band to 4.4–7.3 Hz for the phasic group, but patients with tonic symptoms did not show significant signal changes. Similarly, GPi-DBS for 180 s suppressed alpha and beta oscillatory activity with corresponding improvement in motor performance [Citation92]. At third study randomized seven dystonia patients undergoing DBS implantation or battery replacement to 15 min of continuous stimulation, active stimulation, or no stimulation immediately after new DBS placement or battery replacement [Citation93]. Active stimulation was delivered for 2–4 min whenever high amplitude alpha oscillations (4–12 Hz) were detected. No significant difference was found across the three groups when evaluating for suppression of alpha oscillations. However, in patients whose dystonia symptoms had been effectively treated with chronic DBS and were undergoing battery replacement, alpha oscillations at rest were lower compared with the newly implanted patients. This correlates with observed clinical improvement in dystonia, and suggests that changes in alpha activity occur gradually in response to GPi-DBS.
Two other studies have investigated how chronic DBS changes LFP activity. In one of them [Citation94], an investigational IPG provided long-term DBS to nine patients with focal-segmental or generalized dystonia for 6–51 months. Stimulation was turned off for 5–7 h and GPi LFPs were sampled at five different time points. This study found progressive increase in pallidal low frequency 3–12 Hz activity, which correlated with increased Burke-Fahn-Marsden Dystonia Rating Scale scores. Beta peaks were also identified, but they were not consistently present at every time point like the alpha activity. Another study similarly evaluated signal changes after turning off chronic GPi or STN DBS for up to 90 min [Citation95]. In this study, the authors did not have the capability of recording LFPs and instead used EEG recordings from the primary motor cortex, which correlates with GPi low frequency activity [Citation81,Citation86]. Yet, it was shown that EEG alpha activity gradually increased during the DBS washout period and that it decreased again when DBS was restarted. To date, there have been no published studies that prospectively evaluate pallidal signal changes over months from the initiation of DBS therapy.
LFPs in ET
In ET, there is excessive LFP synchronization in the theta and beta bands [Citation96,Citation97]. Reduction in this beta activity (14–30 Hz) and increase in gamma oscillations (55–80 Hz) have been reported during volitional movements and have been proposed for adaptive DBS [Citation98,Citation99]. In the future, detecting thalamic beta oscillations may guide DBS therapy as they appear to be stronger within the dentate-rubro-thalamic tract, in a location possibly associated with better tremor control over time [Citation100].
Other potential neurophysiological biomarkers
Similar to PD, electrocorticographic signals from the motor cortex have been used to pilot adaptive DBS paradigms in ET by reducing cortical phase-amplitude coupling [Citation101]. Studies using peripheral measures, such as accelerometry and EMG, have shown that extremity tremor and abnormally synchronized thalamic LFPs are both in the theta range (4–8 Hz). However, studies of thalamo-muscular coherence have shown that EMG activity starts approximately 200 ms after onset of tremor activity in the Vim. This delay would make EMG an unreliable marker for closed-loop, adaptive DBS systems [Citation102]. However, peripheral measures of tremor are important for pathophysiological studies. For instance, correlating thalamic neuronal activity with tremor activity measured by EMG allowed identification of specific neuronal populations and their relationship with the outcomes of DBS [Citation80].
Future perspective
Emerging neuroimaging, stereotactic neurosurgical and electrophysiological techniques that combine sophisticated pre-surgical and post-surgical neuroanatomical information with intraoperative neurophysiological information will allow for optimization of DBS therapy by improving the accuracy of surgical DBS lead implantation. This multi-modal information from the targeted region could be used during post-operative programming when using conventional or directional DBS. Additional DBS technology that measures post-operative neurophysiological information from the implanted neuroanatomical location, particularly LFPs, allows delivering DBS to pathophysiologically relevant regions of the targeted anatomical region. The use of post-operative neurophysiological information might reduce the time it takes to reach optimized parameters for chronic, continuous DBS and thus increase the time that patients have the highest possible quality of life. Currently, DBS therapy for movement disorders could be physiologically optimized by targeting pathological LFPs and anatomically optimized by directional stimulation to avoid side effects. This combination of multi-modal pre-operative and post-operative information could potentially improve the outcomes of DBS ().
As we continue to refine our knowledge of the clinical relevance of specific characteristics of neurophysiological signals, there is a tremendous opportunity to utilize this information for adaptive DBS to treat movement disorders [Citation73,Citation91]. The ideal adaptive DBS system would be able to differentiate and individualize specific characteristics of the measured neurophysiological signals in real time, to then automatically deliver therapeutic electrical pulses of specific parameters for a specific amount of time. Targeting specific characteristics of individualized neurophysiological signals (frequency, amplitude, duration, shape, etc.) from anatomically and physiologically optimized targets would be more likely to improve motor symptoms with the least amount of energy and without causing side effects [Citation103]. For example, adaptive STN-DBS will treat akinetic-rigid symptoms in PD by specifically suppressing low beta band LFPs of longer duration at the corresponding hemisphere only while the symptoms last [Citation45,Citation56]. At least two limitations of the currently available DBS technology need to be overcome before adaptive DBS becomes a reality: LFP measurement and delivery of DBS pulses cannot occur simultaneously, and theta/alpha, beta and gamma band LFPs are measurable but not high frequency oscillations, which may be more relevant for tremor. Another potential obstacle of adaptive DBS is that promoting prokinetic and inhibiting antikinetic signals may increase the risk of hyperkinetic complications. There is a burst of exciting information coming along with a wavy and possibly spiky road ahead but neurophysiological biomarkers have great potential to optimize DBS and move the field toward adaptive DBS modalities.
(A) During surgical implantation of the left STN DBS lead, microelectrode recordings and stimulation defined a physiologically optimized trajectory and target in the dorsolateral region of the anatomically defined left STN. (B) During the initial programming session described in , contact E0 was chosen to deliver DBS to the region with the highest peak of low beta LFPs. Upon subsequent DBS amplitude increase, the patient developed subtle side effects due to lateral spread of stimulation toward the IC, which limited the benefits of DBS. (C) With directional stimulation using segmented leads, this lateral spread of stimulation could be theoretically avoided and DBS therapy could be further optimized. Reconstruction of neuroanatomical structures and DBS lead localization were performed by merging pre-operative brain MRI and post-operative CT using BrainLab.
CT: Computed tomography; DBS: Deep brain stimulation; IC: Internal capsule; LFP: Local field potential; STN: Subthalamic nucleus.
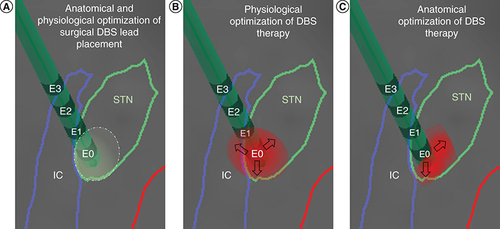
Acknowledgments
The authors would like to thank Laura Rotolo, Michael Williams and Cassandra June for their support in the care of patients treated with deep brain stimulation at the Motor Physiology and Neuromodulation Program at the University of Rochester Medical Center, Rochester, NY, USA.
Financial & competing interests disclosure
KJ Lizarraga and C Zimmerman have been reimbursed for travel to educational activities sponsored by Abbott, Boston Scientific and Medtronic. KJ Lizarraga has received an educational grant by Medtronic. The authors have no other relevant affiliations or financial involvement with any organization or entity with a financial interest in or financial conflict with the subject matter or materials discussed in the manuscript apart from those disclosed.
No writing assistance was utilized in the production of this manuscript.
References
- Lozano AM , LipsmanN, BergmanHet al. Deep brain stimulation: current challenges and future directions. Nat. Rev. Neurol., 15(3), 148–160 (2019).
- Chen CC , BrückeC, KempfFet al. Deep brain stimulation of the subthalamic nucleus: a two-edged sword. Curr. Biol., 16(22), R952–953 (2006).
- Gross RE , KrackP, Rodriguez-OrozMC, RezaiAR, BenabidAL. Electrophysiological mapping for the implantation of deep brain stimulators for Parkinson’s disease and tremor. Mov. Disord., 21(Suppl. 14), S259–S283 (2006).
- Eisenstein SA , KollerJM, BlackKDet al. Functional anatomy of subthalamic nucleus stimulation in Parkinson disease. Ann. Neurol., 76(2), 279–295 (2004).
- Burchiel KJ , McCartneyS, LeeA, RaslanAM. Accuracy of deep brain stimulation electrode placement using intraoperative computed tomography without microelectrode recording. J. Neurosurg., 119(2), 301–306 (2013).
- Montgomery EB Jr . Letter to the editor: deep brain stimulation without microelectrode recording. J. Neurosurg., 120(6), 1497–1498 (2014).
- Lozano CS , RanjanM, BoutetAet al. Imaging alone versus microelectrode recording-guided targeting of the STN in patients with Parkinson’s disease. J. Neurosurg., 130(6), 1847–1852 (2019).
- Yin Z , LuoY, JinYet al. Is awake physiological confirmation necessary for DBS treatment of Parkinson’s disease today? A comparison of intraoperative imaging, physiology, and physiology imaging-guided DBS in the past decade. Brain Stimul., 12(4), 893–900 (2019).
- Horn A , ReichM, VorwerkJet al. Connectivity predicts deep brain stimulation outcome in Parkinson disease. Ann. Neurol., 82(1), 67–78 (2017).
- Akram H , DayalV, MahlknechtPet al. Connectivity derived thalamic segmentation in deep brain stimulation for tremor. Neuroimage Clin., 18, 130–142 (2018).
- Priori A , FoffaniG, PesentiAet al. Rhythm-specific pharmacological modulation of subthalamic activity in Parkinson’s disease. Exp. Neurol., 189(2), 369–379 (2004).
- Ozturk M , TelkesI, Jimenez-ShahedJet al. Randomized, double-blind assessment of LFP versus SUA guidance in STN-DBS lead implantation: a pilot study. Front. Neurosci., 14, 611 (2020).
- Brown P , EusebioA. Paradoxes of functional neurosurgery: clues from basal ganglia recordings. Mov. Disord., 23(1), 12–20 (2008).
- Blomstedt P , HarizMI. Deep brain stimulation for movement disorders before DBS for movement disorders. Parkinsonism Relat. Disord., 16(7), 429–433 (2010).
- Gratwicke J , ZrinzoL, KahanJet al. Bilateral deep brain stimulation of the nucleus basalis of meynert for parkinson disease dementia: a randomized clinical trial. JAMA Neurol., 75(2), 169–178 (2018).
- Starr PA , TheodosopoulosPV, TurnerR. Surgery of the subthalamic nucleus: use of movement-related neuronal activity for surgical navigation. Neurosurgery, 53(5), 1146–1149 (2003).
- Montgomery EB Jr . Microelectrode targeting of the subthalamic nucleus for deep brain stimulation surgery. Mov. Disord., 27(11), 1387–1391 (2012).
- Mehanna R , MachadoAG, ConnettJE, AlsaloumF, CooperSE. Intraoperative microstimulation predicts outcome of postoperative macrostimulation in subthalamic nucleus deep brain stimulation for Parkinson’s disease. Neuromodulation, 20(5), 456–463 (2017).
- Vitek JL , ChockkanV, ZhangJYet al. Neuronal activity in the basal ganglia in patients with generalized dystonia and hemiballismus. Ann. Neurol., 46(1), 22–35 (1999).
- Lozano A , HutchisonW, KissZ, TaskerR, DavisK, DostrovskyJ. Methods for microelectrode-guided posteroventral pallidotomy. J. Neurosurg., 84(2), 194–202 (1996).
- Baker KB , LeeJY, MavinkurveGet al. Somatotopic organization in the internal segment of the globus pallidus in Parkinson’s disease. Exp. Neurol., 222(2), 219–225 (2010).
- Chen CC , LitvakV, GilbertsonTet al. Excessive synchronization of basal ganglia neurons at 20 Hz slows movement in Parkinson’s disease. Exp. Neurol., 205(1), 214–221 (2007).
- Eusebio A , ChenCC, LuCSet al. Effects of low-frequency stimulation of the subthalamic nucleus on movement in Parkinson’s disease. Exp. Neurol., 209(1), 125–130 (2008).
- Agostino R , DinapoliL, ModugnoN, IezziE, RomanelliP, BerardelliA. Effects of unilateral subthalamic deep brain stimulation on contralateral arm sequential movements in Parkinson’s disease. J. Neurol. Neurosurg. Psychiatry, 79(1), 76–78 (2008).
- Tsang EW , HamaniC, MoroEet al. Subthalamic deep brain stimulation at individualized frequencies for Parkinson disease. Neurology, 78(24), 1930–1938 (2012).
- Pinto S , GentilM, KrackPet al. Changes induced by levodopa and subthalamic nucleus stimulation on parkinsonian speech. Mov. Disord., 20(11), 1507–1515 (2005).
- Wojtecki L , TimmermannL, JorgensS, SudmeyerM, MaaroufM, TreuerH. Frequency-dependent reciprocal modulation of verbal fluency and motor functions in subthalamic deep brain stimulation. Arch. Neurol., 63(9), 1273–1276 (2006).
- Khoo HM , KishimaH, HosomiKet al. Low-frequency subthalamic nucleus stimulation in Parkinson’s disease: a randomized clinical trial. Mov. Disord., 29(2), 270–274 (2014).
- di Biase L , FasanoA. Low-frequency deep brain stimulation for Parkinson’s disease: great expectation or false hope?Mov. Disord., 31(7), 962–967 (2016).
- Hammond C , BergmanH, BrownP. Pathological synchronization in Parkinson’s disease: networks, models and treatments. Trends Neurosci., 30(7), 357–364 (2007).
- Kuhn AA , KupschA, SchneiderGH, BrownP. Reduction in subthalamic 8–35 Hz oscillatory activity correlates with clinical improvement in Parkinson’s disease. Eur. J. Neurosci., 23(7), 1956–1960 (2006).
- Neumann WJ , DegenK, SchneiderGHet al. Subthalamic synchronized oscillatory activity correlates with motor impairment in patients with Parkinson’s disease. Mov. Disord., 31(11), 1748–1751 (2016).
- Hirschmann J , SchoffelenJM, SchnitzlerA, van GervenMAJ. Parkinsonian rest tremor can be detected accurately based on neuronal oscillations recorded from the subthalamic nucleus. Clin. Neurophysiol., 128(10), 2029–2036 (2017).
- Lu CW , ChouKL, PatilPG. Correspondence of optimal stimulation and beta power varies regionally in STN DBS for Parkinson disease. Parkinsonism Relat. Disord., 78, 124–128 (2020).
- Horn A , NeumannWJ, DegenK, SchneiderGH, KühnAA. Toward an electrophysiological “sweet spot” for deep brain stimulation in the subthalamic nucleus. Hum. Brain Mapp., 38(7), 3377–3390 (2017).
- Cassidy M , MazzoneP, OlivieroAet al. Movement-related changes in synchronization in the human basal ganglia. Brain, 125(6), 1235–1246 (2002).
- Neumann WJ , HornA, EwertSet al. A localized pallidal physiomarker in cervical dystonia. Ann. Neurol., 82(6), 912–924 (2017).
- Wojtecki L , HirschmannJ, ElbenSet al. Oscillatory coupling of the subthalamic nucleus in obsessive compulsive disorder. Brain, 140(9), e56 (2017).
- Storzer L , ButzM, HirschmannJet al. Bicycling suppresses abnormal beta synchrony in the Parkinsonian basal ganglia. Ann. Neurol., 82(4), 592–601 (2017).
- Fischer P , ChenCC, ChangYJet al. Alternating modulation of subthalamic nucleus beta oscillations during stepping. J. Neurosci., 38(22), 5111–5121 (2018).
- Neumann WJ , SchrollH, deAlmeida Marcelino ALet al. Functional segregation of basal ganglia pathways in Parkinson’s disease. Brain, 141(9), 2655–2669 (2018).
- Bronte-Stewart H , BarberiniC, KoopMM, HillBC, HendersonJM, WingeierB. The STN beta-band profile in Parkinson’s disease is stationary and shows prolonged attenuation after deep brain stimulation. Exp. Neurol., 215(1), 20–28 (2009).
- Kuhn AA , KupschA, SchneiderGH, BrownP. Reduction in subthalamic 8–35 Hz oscillatory activity correlates with clinical improvement in Parkinson’s disease. Eur. J. Neurosci., 23(7), 1956–1960 (2006).
- Beudel M , OswalA, JhaAet al. Oscillatory beta power correlates with akinesia-rigidity in the Parkinsonian subthalamic nucleus. Mov. Disord., 32(1), 174–175 (2017).
- Tinkhauser G , PogosyanA, TanH, HerzDM, KuhnAA, BrownP. Beta burst dynamics in Parkinson’s disease OFF and ON dopaminergic medication. Brain, 140(11), 2968–2981 (2017).
- Deffains M , IskhakovaL, KatabiS, IsraelZ, BergmanH. Longer β oscillatory episodes reliably identify pathological subthalamic activity in Parkinsonism. Mov. Disord., 33(10), 1609–1618 (2018).
- Lofredi R , NeumannWJ, BrückeCet al. Pallidal beta bursts in Parkinson’s disease and dystonia. Mov. Disord., 34(3), 420–424 (2019).
- Anidi C , O’DayJJ, AndersonRWet al. Neuromodulation targets pathological not physiological beta bursts during gait in Parkinson’s disease. Neurobiol. Dis., 120, 107–117 (2018).
- Özkurt TE , ButzM, HomburgerMet al. High frequency oscillations in the subthalamic nucleus: a neurophysiological marker of the motor state in Parkinson’s disease. Exp. Neurol., 229(2), 324–331 (2011).
- Foffani G , PrioriA, EgidiMet al. 300-Hz subthalamic oscillations in Parkinson’s disease. Brain, 126(10), 2153–2163 (2003).
- Litvak V , EusebioA, JhaAet al. Movement-related changes in local and long-range synchronization in Parkinson’s disease revealed by simultaneous magnetoencephalography and intracranial recordings. J. Neurosci., 32(31), 10541–10553 (2012).
- Hirschmann J , ButzM, HartmannCJet al. Parkinsonian rest tremor is associated with modulations of subthalamic high-frequency oscillations. Mov. Disord., 31(10), 1551–1559 (2016).
- Beudel M , LittleS, PogosyanAet al. Tremor reduction by deep brain stimulation is associated with gamma power suppression in Parkinson’s disease. Neuromodulation, 18(5), 349–354 (2015).
- Alonso-Frech F , ZamarbideI, AlegreMet al. Slow oscillatory activity and levodopa-induced dyskinesias in Parkinson’s disease. Brain, 129(7), 1748–1757 (2006).
- Liu X , Ford-DunnHL, HaywardGNet al. The oscillatory activity in the Parkinsonian subthalamic nucleus investigated using the macro-electrodes for deep brain stimulation. Clin. Neurophysiol., 113(11), 1667–1672 (2002).
- Alegre M , Lopez-AzcarateJ, Alonso-FrechFet al. Subthalamic activity during diphasic dyskinesias in Parkinson’s disease. Mov. Disord., 27(9), 1178–1181 (2012).
- Swann NC , de HemptinneC, MiocinovicSet al. Gamma oscillations in the hyperkinetic state detected with chronic human brain recordings in Parkinson’s disease. J. Neurosci., 36(24), 6445–6458 (2016).
- Tinkhauser G , PogosyanA, LittleSet al. The modulatory effect of adaptive deep brain stimulation on beta bursts in Parkinson’s disease. Brain, 140(4), 1053–1067 (2017).
- Lizarraga KJ , NaghibzadehM, BoutetA, EliasGJB, FasanoA. Management of Pisa syndrome with lateralized subthalamic stimulation. J. Neurol., 265(10), 2442–2444 (2018).
- St George RJ , NuttJG, BurchielKJ, HorakFB. A meta-regression of the long-term effects of deep brain stimulation on balance and gait in PD. Neurology, 75(14), 1292–1299 (2010).
- Fasano A , AquinoCC, KraussJK, HoneyCR, BloemBR. Axial disability and deep brain stimulation in patients with Parkinson disease. Nat. Rev. Neurol., 11(2), 98–110 (2015).
- Fischer P , ChenCC, ChangYJet al. Alternating modulation of subthalamic nucleus beta oscillations during stepping. J. Neurosci., 38(22), 5111–5121 (2018).
- Syrkin-Nikolau J , KoopMM, PrietoTet al. Subthalamic neural entropy is a feature of freezing of gait in freely moving people with Parkinson’s disease. Neurobiol. Dis., 108, 288–297 (2017).
- Lizarraga KJ , LucaCC, DeSalles A, GorgulhoA, LangAE, FasanoA. Asymmetric neuromodulation of motor circuits in Parkinson’s disease: the role of subthalamic deep brain stimulation. Surg. Neurol. Int., 8, 261 (2017).
- Lofredi R , NeumannWJ, BockAet al. Dopamine-dependent scaling of subthalamic gamma bursts with movement velocity in patients with Parkinson’s disease. Elife, 7, e31895 (2018).
- Hirschmann J , HartmannCJ, ButzMet al. A direct relationship between oscillatory subthalamic nucleus-cortex coupling and rest tremor in Parkinson’s disease. Brain, 136(12), 3659–3670 (2013).
- Crowell AL , Ryapolova-WebbES, OstremJLet al. Oscillations in sensorimotor cortex in movement disorders: an electrocorticography study. Brain, 135(2), 615–630 (2012).
- Cole SR , vander Meij R, PetersonEJ, de HemptinneC, StarrPA, VoytekB. Nonsinusoidal beta oscillations reflect cortical pathophysiology in Parkinson’s disease. J. Neurosci., 37(18), 4830–4840 (2017).
- Swann NC , de HemptinneC, ThompsonMCet al. Adaptive deep brain stimulation for Parkinson’s disease using motor cortex sensing. J. Neural. Eng., 15(4), 046006 (2018).
- Meissner W , LebloisA, HanselDet al. Subthalamic high frequency stimulation resets subthalamic firing and reduces abnormal oscillations. Brain, 128(10), 2372–2382 (2005).
- Abosch A , LanctinD, OnaranI, EberlyL, SpaniolM, InceNF. Long-term recordings of local field potentials from implanted deep brain stimulation electrodes. Neurosurgery, 71(4), 804–814 (2012).
- Rossi L , FoffaniG, MarcegliaS, BracchiF, BarbieriS, PrioriA. An electronic device for artefact suppression in human local field potential recordings during deep brain stimulation. J. Neural. Eng., 4, 96–106 (2007).
- Arlotti M , MarcegliaS, FoffaniGet al. Eight-hours adaptive deep brain stimulation in patients with Parkinson disease. Neurology, 90(11), e971–e976 (2018).
- Neumann WJ , Staub-BarteltF, HornAet al. Long term correlation of subthalamic beta band activity with motor impairment in patients with Parkinson’s disease. Clin. Neurophysiol., 128(11), 2286–2291 (2017).
- Trager MH , KoopMM, VelisarAet al. Subthalamic beta oscillations are attenuated after withdrawal of chronic high frequency neurostimulation in Parkinson’s disease. Neurobiol. Dis., 96, 22–30 (2016).
- Chen Y , GongC, TianYet al. Neuromodulation effects of deep brain stimulation on beta rhythm: a longitudinal local field potential study. Brain Stimul., 13(6), 1784–1792 (2020).
- Lizarraga KJ , Al-ShorafatD, FoxS. Update on current and emerging therapies for dystonia. Neurodegener. Dis. Manag., 9(3), 135–147 (2019).
- Ostrem JL , RacineCA, GlassGAet al. Subthalamic nucleus deep brain stimulation in primary cervical dystonia. Neurology, 76(10), 870–878 (2011).
- Benabid AL , PollakP, GaoDet al. Chronic electrical stimulation of the ventralis intermedius nucleus of the thalamus as a treatment of movement disorders. J. Neurosurg., 84(2), 203–214 (1996).
- Pedrosa DJ , BrownP, CagnanHet al. A functional micro-electrode mapping of ventral thalamus in essential tremor. Brain, 141(9), 2644–2654 (2018).
- Barow E , NeumannWJ, BrückeCet al. Deep brain stimulation suppresses pallidal low frequency activity in patients with phasic dystonic movements. Brain, 137(11), 3012–3024 (2014).
- Chen CC , KühnAA, HoffmannKTet al. Oscillatory pallidal local field potential activity correlates with involuntary EMG in dystonia. Neurology, 66(3), 418–420 (2006).
- Lee JR , KissZH. Interhemispheric difference of pallidal local field potential activity in cervical dystonia. J. Neurol. Neurosurg. Psychiatry, 85(3), 306–310 (2014).
- Singh A , KammermeierS, PlateA, MehrkensJH, IlmbergerJ, BötzelK. Pattern of local field potential activity in the globus pallidus internum of dystonic patients during walking on a treadmill. Exp. Neurol., 232(2), 162–167 (2011).
- Weinberger M , HutchisonWD, AlaviMet al. Oscillatory activity in the globus pallidus internus: comparison between Parkinson’s disease and dystonia. Clin. Neurophysiol., 123(2), 358–368 (2012).
- Yokochi F , KatoK, IwamuroHet al. Resting-state pallidal-cortical oscillatory couplings in patients with predominant phasic and tonic dystonia. Front. Neurol., 9, 375 (2018).
- Geng X , ZhangJ, JiangYet al. Comparison of oscillatory activity in subthalamic nucleus in Parkinson’s disease and dystonia. Neurobiol. Dis., 98, 100–107 (2017).
- Wang DD , de HemptinneC, MiocinovicSet al. Subthalamic local field potentials in Parkinson’s disease and isolated dystonia: an evaluation of potential biomarkers. Neurobiol. Dis., 89, 213–222 (2016).
- Liu X , YianniJ, WangS, BainPG, SteinJF, AzizTZ. Different mechanisms may generate sustained hypertonic and rhythmic bursting muscle activity in idiopathic dystonia. Exp. Neurol., 198(1), 204–213 (2006).
- Brücke C , HueblJ, SchöneckerTet al. Scaling of movement is related to pallidal γ oscillations in patients with dystonia. J. Neurosci., 32(3), 1008–1019 (2012).
- Liu X , WangS, YianniJet al. The sensory and motor representation of synchronized oscillations in the globus pallidus in patients with primary dystonia. Brain, 131(6), 1562–1573 (2008).
- Zhu GY , ZhangRL, ChenYCet al. Characteristics of globus pallidus internus local field potentials in generalized dystonia patients with TWNK mutation. Clin. Neurophysiol., 131(7), 1453–1461 (2020).
- Piña-Fuentes D , BeudelM, Van ZijlJCet al. Low-frequency oscillation suppression in dystonia: implications for adaptive deep brain stimulation. Parkinsonism Relat. Disord., 79, 105–109 (2020).
- Scheller U , LofrediR, van WijkBCet al. Pallidal low-frequency activity in dystonia after cessation of long-term deep brain stimulation. Mov. Disord., 34(11), 1734–1739 (2019).
- Miocinovic S , MillerA, SwannNC, OstremJL, StarrPA. Chronic deep brain stimulation normalizes scalp EEG activity in isolated dystonia. Clin. Neurophysiol., 129(2), 368–376 (2018).
- Kane A , HutchisonWD, HodaieM, LozanoAM, DostrovskyJO. Enhanced synchronization of thalamic theta band local field potentials in patients with essential tremor. Exp. Neurol., 217(1), 171–176 (2009).
- Yousif N , MaceM, PaveseN, BorisyukR, NandiD, BainP. A network model of local field potential activity in essential tremor and the impact of deep brain stimulation. PLoS Comput. Biol., 13(1), e1005326 (2017).
- Basha D , DostrovskyJO, LopezRios AL, HodaieM, LozanoAM, HutchisonWD. Beta oscillatory neurons in the motor thalamus of movement disorder and pain patients. Exp. Neurol., 261, 782–790 (2014).
- Tan H , DebarrosJ, HeSet al. Decoding voluntary movements as postural tremor based on thalamic LFPs as a basis for closed-loop stimulation for essential tremor. Brain Stimul., 12(4), 858–867 (2019).
- Sandoe C , KrishnaV, BashaDet al. Predictors of deep brain stimulation outcome in tremor patients. Brain Stimul., 11(3), 592–599 (2018).
- Kondylis ED , RandazzoMJ, AlhouraniAet al. Movement-related dynamics of cortical oscillations in Parkinson’s disease and essential tremor. Brain, 139(8), 2211–2223 (2016).
- Pedrosa DJ , QuatuorEL, ReckCet al. Thalamomuscular coherence in essential tremor: hen or egg in the emergence of tremor? J. Neurosci., 34(43), 14475–14483 (2014).
- Wiest C , TinkhauserG, PogosyanAet al. Local field potential activity dynamics in response to deep brain stimulation of the subthalamic nucleus in Parkinson’s disease. Neurobiol. Dis., 143, 105019 (2020).