Abstract
Aim: The clinical application of sorafenib is limited because of its hydrophobicity, low bioavailability and unsatisfying treatment effect. Therefore, sorafenib-loaded PEG-poly (ε-caprolactone) micelles (SF micelles) were fabricated for sorafenib delivery. Materials & methods:In vitro assays investigated the solubility, dispersity, stability, cytotoxicity and uptake capacity of SF micelles. In vivo biodistribution and therapeutic effects were studied using HepG2-Luc tumor-bearing mice. Results: SF micelles had a regular spherical structure with good water solubility. In vivo imaging results showed PEG-poly (ε-caprolactone) micelles could elevate the sorafenib concentration in tumor tissues. Meanwhile, SF micelles exhibited higher tumor growth inhibition in vivo. Conclusion: SF micelles might be a potential drug delivery system, which could enhance the therapeutic effects of sorafenib.
Hepatocellular carcinoma (HCC) is one of the most common malignant tumors, and most HCC patients are diagnosed at an advanced stage [Citation1–3]. However, there was no effective treatment for the patients with advanced HCC until sorafenib was approved [Citation4]. Sorafenib as an oral tablet has a poor water solubility of approximately 1.7 μg/ml [Citation5], which leads to low oral bioavailability. The bioavailability is extremely low, about 38–49%, relative to an oral solution, and it is also seriously affected by the diet [Citation5,Citation6]. Furthermore, as with most small-molecule drugs, sorafenib may distribute throughout the whole body and be rapidly metabolized in the body, leading to a range of serious adverse reactions, including hand-foot syndrome, diarrhea, rash, fatigue and nausea [Citation7]. These drawbacks have largely restricted the therapeutic effects. Therefore, effective strategies to enhance the anticancer activity of this drug are urgently needed.
Sorafenib is a multikinase inhibitor that inhibits the activities of tyrosine kinases, including PDGF and VEGF receptors and blocks the signaling pathway of serine/threonine kinases Raf-1 and B-Raf [Citation8]. Its administration has been proven to be beneficial for the survival of advanced HCC patients [Citation9,Citation10]. To date, sorafenib is the only clinically approved molecule-targeting drug as the standard treatment for advanced HCC [Citation11,Citation12]. In recent years, the therapeutic effects of sorafenib were considered to be not very satisfactory. Therefore, some researchers have focused on developing new formulations and administration methods of sorafenib to improve its efficacy. Zhang et al. developed sorafenib and gadolinium co-loaded liposomes (SF/Gd-liposomes) for MRI-guided HCC therapy, which could accumulate in the tumor tissues and exhibited higher antitumor efficacy in vivo [Citation13]. Shao et al. reported that mesoporous silica nanoparticles were modified with chitosan-lactobionic acid to co-deliver sorafenib and ursolic acid for the prevention of metastatic HCC [Citation14]. In the past few decades, a variety of nanotechnology-based drug delivery systems, such as liposomes [Citation15,Citation16], dendrimers [Citation17,Citation18], micelles [Citation19,Citation20] and inorganic nanoparticles [Citation21,Citation22] have emerged to improve the properties of small-molecule drugs. These materials can improve the solubility and stability of insoluble drugs, prolong blood circulation time, decrease distribution in normal tissues and increase drug concentration in tumor lesions through the enhanced permeation and retention (EPR) effect [Citation23]. Consequently, the use of these drug delivery systems has improved the therapeutic effects of drugs in different types of cancers [Citation24–27]. As a drug carrier, PEG-poly (ε-caprolactone) [PEG-PCL] micelles have many advantages over other drug carriers. First, PEG and PCL are biocompatible and both have been approved by the US FDA [Citation28,Citation29]. Second, PEG-PCL micelles are easy to prepare, which can self-assemble into a core-shell structure in water. The outer PEG shell can confer the nanoparticles with a ‘stealth’ state, minimizing nonspecific cellular uptake by macrophages to evade the rapid clearance by the reticuloendothelial system (RES) [Citation30]. Third, the low critical micelle concentration (CMC) of the PEG-PCL copolymer can allow the micelles to maintain high stability after intravenous injection [Citation31].
Accordingly, in this study, we used the amphiphilic copolymer PEG-PCL to carry sorafenib for the treatment of advanced HCC. Sorafenib-loaded PEG-PCL micelles (SF micelles) were prepared and characterized, and the in vitro cellular uptake and cytotoxicity studies were tested on HepG2 and BEL-7402 cells. The in vivo fluorescence molecular imaging (FMI) and photoacoustic imaging (PAI) were performed to evaluate the biodistribution and penetration of PEG-PCL micelles in tumor tissues. The therapeutic efficacy and safety evaluation of SF micelles were monitored using the HepG2-Luc tumor models during the treatment for 21 days.
Materials & methods
Materials
Sorafenib tosylate was purchased from Adamas (Shanghai, China). PEG2k-PCL5k was obtained from DaiGang Biotechnology Co., Ltd (Jinan, Shandong, China). Pyrene was purchased from Alfa Aesar (Beijing, China). Coumarin-6 (C-6) and dimethyl sulfoxide were obtained from Acros Organics (Shanghai, China). 1,1′-Dioctadecyl-3,3,3′,3′-tetramethylindotricarbocyanine iodide (DiR) was provided by Biotium (CA, USA). Acetonitrile and methanol for HPLC were provided by Beijing InnoChem Science & Technology Co., Ltd (Beijing, China). 3-(4,5-Dimethylthiazol-2-yl)-5-(3-carboxymethoxyphenyl)-2-(4-sulfophenyl)-2H-tetrazolium (MTS) was purchased from Promega (WI, USA). 4′,6-Diamidino-2-phenylindole (DAPI) and phalloidin-tetramethylrhodamine B isothiocyanate were obtained from Sigma–Aldrich (MO, USA). Other solvents were of analytical grade.
Preparation of SF micelles
SF micelles were prepared using a dialysis method [Citation32]. Briefly, 2 mg sorafenib and 10 mg PEG-PCL copolymer were dissolved in 1 ml DMF under stirring for 30 min at 30°C. Next, 5 ml of deionized water was added, and stirring was continued for another 30 min. Thereafter, the mixture was dialyzed against deionized water using a dialysis bag (molecular weight cut-off = 3.5 kDa) to remove DMF. Finally, SF micelles were filtered through a 0.22-μm membrane to remove the unencapsulated sorafenib and sterilize the micellar solution. Then, the prepared samples were stored at 4°C for further use. C-6- and DiR-loaded PEG-PCL micelles (C-6 and DiR micelles, respectively) were obtained using a similar method.
Determination of the CMC
The CMC value was measured using pyrene as a fluorescent probe with a fluorescence spectrophotometer [Citation33]. A predetermined volume of pyrene acetone solution was added in a series of sample bottles, and all bottles were put in a freeze dryer overnight to remove acetone. Then, precalculated volumes of micelle solution and deionized water were added to the sample bottles to obtain a range of micelle solutions with different concentrations (from 0.5 to 10-5 mg/ml), and the concentration of pyrene in each bottle was maintained at 6.0 × 10-7 M. All bottles were then shaken for 24 h, and an F-7000 FL spectrophotometer (Hitachi, Tokyo, Japan) was used for spectral measurement at an emission wavelength of 390 nm.
Characterization of SF micelles
The morphology of SF micelles was photographed using transmission electron microscopy (HT7700, Hitachi). All samples were dripped on a copper grid and negatively stained with 2% phosphotungstic acid (w/v). The particle size, polydispersity index (PDI) and ζ-potential of SF micelles were characterized by dynamic light scattering (DLS, Zetasizer Nano ZS90, Malvern, UK). To assess the stability of SF micelles, the prepared samples were stored at 4 and 37°C, and the particle size, PDI and ζ-potential were measured every day for 1 week.
The drug loading (DL) and entrapment efficiency (EE) were analyzed by HPLC [Citation28]. SF micelles were freeze-dried, of which 2 mg was dissolved in methanol and analyzed using HPLC (Shimadzu, Japan) with a C-18 column (4.6 × 250 mm, 5 μm; Shimadzu, Kyoto, Japan). The mobile phase, comprising methanol: acetonitrile: acetic acid (1%) at 38:35:27 (v/v/v), was used to elute at a flow rate of 1.0 ml/min under 264 nm and the column temperature was set at 37°C. The DL and EE of SF micelles were obtained using the following equations:
In vitro sorafenib release
The release of sorafenib from SF micelles was performed using a dialysis method. Briefly, 1 ml solution of SF micelles was added to a dialysis bag (molecular weight cut-off = 3500 Da) and put into 40 ml PBS (pH 7.4 and 6.0) with 1% sodium dodecyl sulfate to obtain sink conditions. All samples were shaken at 100 r.p.m. and 37°C in an orbital shaking incubator. At specific time intervals, 1 ml original release medium was withdrawn and 1 ml fresh medium was added. Finally, the amount of released sorafenib was quantified by HPLC.
Cell culture & tumor model
Luciferase-expressing HepG2 and BEL-7402 cells (HepG2-Luc and BEL-7402-Luc) were cultured in DMEM (Life Technologies Co.) supplemented with 10% fetal bovine serum (Life Technologies Co.) and 1% penicillin-streptomycin solution (M&C Gene Technology Co., Ltd) at 37°C in a humidified incubator with an atmosphere containing 5% CO2.
Male BALB/C nude mice (4–6 weeks old) were purchased from Vital River Laboratory Animal Technology Co., Ltd (Beijing, China). All animal experiments were performed in accordance with the protocol approved by the Institutional Animal Care and Use Committee of Peking University. A total of 100 μl of HepG2-Luc cells (2 × 106) dispersed in PBS/Matrigel matrix was injected subcutaneously into the back of a mouse. When the tumor volume was about 100 mm3, the animal model could be used for experiments.
Cellular uptake study
C-6 was used as a substitute of the hydrophobic sorafenib to evaluate the uptake capacity of HepG2 and BEL-7402 cells [Citation34]. The cells (1 × 105 cells/well) were seeded on round glass slides in 12-well plates. After 24 h, the medium was removed and fresh medium containing free C-6 or C-6 micelles was added at a C-6 concentration of 1 μg/ml. After C-6 or C-6 micelles were removed and washed three-times with PBS 4 h later, the cells were fixed with 4% paraformaldehyde for 15 min, and then treated with 0.1% Triton for 5 min to increase cell membrane penetrability. The cytoskeleton was then stained with rhodamine phalloidin (red), and nuclei were stained with DAPI (blue). The uptake behavior was observed using laser scanning confocal microscopy.
Cytotoxicity study
The cytotoxicity of empty PEG-PCL micelles, free sorafenib and SF micelles in HepG2 and BEL-7402 cells was evaluated using MTS assays. Briefly, the cells (1 × 104 cells/well) were seeded into 96-well plates and adhered to the wells overnight at 37°C. Different concentrations of empty PEG-PCL micelles, free sorafenib and SF micelles were added to the wells. After 24, 48 or 72 h, MTS solution was added and then incubated for another 4 h. The absorbance of each well was detected on a BioTek Synergy HT Universal Microplate Reader (Bio Tek, VT, USA) to evaluate the cell viability.
Apoptosis assay
For cell apoptosis assays, HepG2 and BEL-7402 cells were cultured in a six-well plate overnight and treated with free sorafenib (5 μM) and SF micelles (5 μM) and empty micelles (with sorafenib concentration equivalent to 5 μM) for 48 h. Untreated cells served as the control group. Subsequently, the cells were washed, trypsinized, collected and incubated with Annexin V-FITC and propidium iodide (PI) in the dark. Finally, the cells were examined using FACS.
In vivo biodistribution & tumor penetrability
For in vivo biodistribution, the hydrophobic near-infrared (NIR) dye DiR was used as a model to visualize the biodistribution of polymeric micelles [Citation35]. HepG2-Luc tumor-bearing mice were grouped randomly and intravenously administered 200 μl of free DiR solution and DiR micelles (0.5 mg DiR/kg). At 2, 4, 6, 8, 12, 24 and 48 h postinjection, the fluorescent signals of DiR in vivo were obtained using a NIR fluorescence imaging system (ex = 748 nm, em = 780 nm). Finally, the mice were sacrificed, and the tumors and major organs (heart, liver, spleen, lung and kidney) were dissected 48 h after administration for ex vivo imaging.
For in vivo tumor penetrability, 200 μl of free DiR solution and DiR micelles (0.5 mg DiR/kg) were intravenously administered to HepG2-Luc tumor-bearing mice. Photoacoustic images were acquired using a multispectral photoacoustic tomography system at 24 h after injection with six excitation wavelengths (680, 700, 740, 750, 760 and 770 nm). During tumor imaging, the equipment scanned the mice from the upper limbs to the liver with a step width of 0.3 mm.
In vivo therapeutic effects
To investigate the therapeutic effects of SF micelles in vivo, the HepG2-Luc tumor-bearing mice were randomly separated into three groups (n = 5) when the tumor volume was about 100 mm3. During the treatment, mice were intravenously administered with PBS, free sorafenib or SF micelles every 3 days (5 mg sorafenib/kg). Changes in tumor volume and body weight were monitored every 2 days, and the tumor volume was computed using the following formula: volume = 0.5 × length × width2. All mice were sacrificed after 21 days of treatment, and tumors were dissected and weighed to evaluate the antitumor efficacy.
Statistical analysis
The data were presented as mean ± standard deviation. The level of the statistical significance of the experimental results was evaluated by Student's t-test, and p < 0.05 was regarded statistically significant.
Results
Preparation & characterization of micelles
In order to improve the solubility and bioavailability of sorafenib, and further increase its therapeutic effect, we prepared water-soluble SF micelles. In vitro and in vivo experiments were carried out to explore the bioavailability and therapeutic effect of SF micelles. Amphiphilic copolymer PEG-PCL self-assembled into spherical micelles in water and sorafenib was encapsulated into the hydrophobic core through hydrophobic interactions, forming a homogeneous solution, as shown in A. We prepared a series of micelles with different sorafenib/PEG-PCL mass ratios to determine the optimal formulation, and some physicochemical properties of these micelles were characterized. The results were listed in Supplementary Table 1. The DL of SF micelles increased from 0 to 20.8% (wt%), with sorafenib/PEG-PCL mass ratios from 0/20 to 6/20, and the EE of all samples was more than 90%. At the same time, the average hydrodynamic diameters increased from 58.95 ± 0.38 to 111.73 ± 7.88 nm, and the PDI also exhibited an increasing tendency. A large number of sorafenib molecules entered the hydrophobic core when more sorafenib was added during the preparation process, thus resulting in an increase in particle size.
(A) Schematic diagram of the preparation of SF micelles with a core-shell structure. (B) Critical micelle concentration of PEG-PCL micelles determined by fluorescence spectrophotometer using pyrene as a fluorescent probe. (C) Dynamic light scattering analysis and (D) Transmission electron microscopy images of SF micelles. (E) Photographs of (1) sorafenib in water; (2) SF micelles in water; (3) Tyndall effects of SF micelle solution.
PCL: Poly (ε-caprolactone); SF micelle: Sorafenib-loaded PEG-poly (ε-caprolactone) micelle
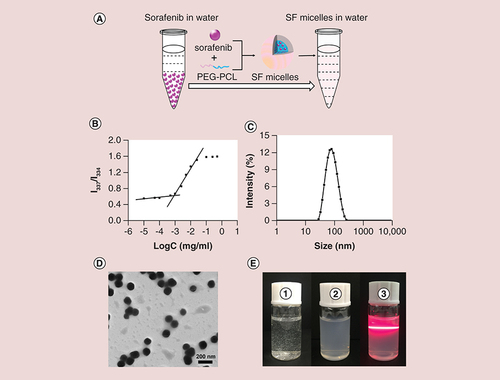
Considering the size, PDI and DL of SF micelles, S4 (4/20) was selected as the optimal formulation for the further study. The CMC of PEG2k-PCL5k is an important parameter in biological applications. Notably, the CMC of PEG2k-PCL5k was 0.867 mg/l (B), which was low enough to ensure the structural stability of the micelles during systemic circulation [Citation33]. Sorafenib was loaded into the PEG-PCL micelles with a remarkable high EE of 92.56% and DL of about 15.43%. The DL of the SF micelles was higher than that of the other nanocarriers used for sorafenib delivery [Citation13,Citation35,Citation36]. The prepared SF micelles with a sorafenib loading of 15.43% exhibited greater therapeutic effects in vivo, which indicated that the DL was sufficient for animal studies. The average size and PDI measured by DLS were 73.32 ± 0.12 and 0.188 ± 0.013 nm, respectively. (C). Transmission electron microscopy results for SF micelles were consistent with the DLS results, which showed SF micelles were a spherical structure with a narrow size distribution and favorable dispersity in water (D). The ζ-potential of SF micelles was slightly negative with the value of -6.52 mV.
As shown in E, sorafenib was almost insoluble in water, which formed a white turbid solution, whereas, SF micelles showed a uniform and opalescent appearance and had an obvious Tyndall effect, indicating that SF micelles had good solubility and dispersity. The solubility of the prepared SF micelles could reach 500 μg/ml, which was much higher than that of free sorafenib in water (1.7 μg/ml).
Stability of SF micelles
The stability of SF micelles was investigated at 4 and 37°C for a week. As shown in A, there was no change in the appearance of SF micelles when they were stored in 4 and 37°C for 1 week. Besides, both the particle size and ζ-potential showed no significant variations during the storage time (B & D), and the PDI values varied slightly, but still remained below 0.3 (C). The excellent storage stability of SF micelles ensured its storage at different temperatures and blood circulation through the body until reaching the tumor site.
In vitro sorafenib release
The release behavior of sorafenib in vitro was analyzed in PBS at pH 6.0 and 7.4, which represented the pH of the tumor microenvironment and physiological environment at 37°C, respectively. There were no significant differences in release rates during the first 12 h at the different pH values. In general, the sorafenib release rate at pH 6.0 was slightly faster than that at pH 7.4, achieving cumulative sorafenib release of 61.1% at pH 7.4 and 66.7% at pH 6.0 after 240 h (). This is probably because of the tendency of the polymeric micelles to be degraded at an acidic pH [Citation37], and the higher solubility of sorafenib under acidic conditions compared with that in the physiological environment [Citation38].
In vitro cellular uptake, cytotoxicity & apoptosis study
The uptake capacity of the polymeric micelles by HepG2 and BEL-7402 cells was assessed using laser scanning confocal microscopy. C-6, as a substitute of sorafenib, was loaded into PEG-PCL micelles to form C-6 micelles. After incubating for 4 h with free C-6 and C-6 micelles, the green fluorescent signals of C-6 in cells were much higher than that of free C-6 in both HepG2 cells (A) and BEL-7402 cells (B). In other words, PEG-PCL micelles could enhance the uptake of sorafenib by the HepG2 and BEL-7402 cells, and help to raise the bioavailability of sorafenib.
Confocal laser scanning microscopy of HepG2 cells (A) and BEL-7402 cells (B) after incubation with free C-6 or C-6 micelles for 4 h at 37°C. Cell nuclei and cytoskeletal F-actin were counterstained with DAPI (blue) and rhodamine phalloidin (red), respectively. The scale bar was 10 μm. In vitro cell viability of HepG2 and BEL-7402 cells incubated with empty micelles, free sorafenib and SF micelles at different concentrations for 24 h (C & F), 48 h (D & G) and 72 h (E & H) at 37°C.
C-6: Coumarin-6; C-6 micelle: Coumarin-6-loaded PEG-poly (ε-caprolactone) micelle; SF micelle: Sorafenib-loaded PEG-poly (ε-caprolactone) micelle.
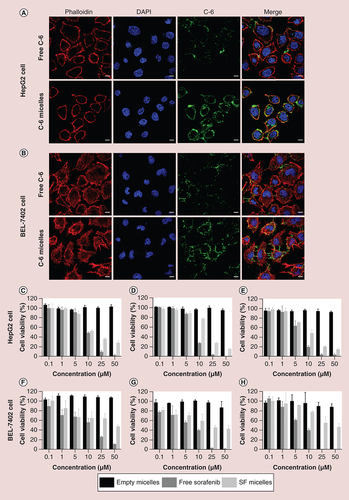
The antiproliferative activity of free sorafenib and SF micelles and the biocompatibility of empty micelles were evaluated by MTS assays. The empty micelles showed no toxicity to HepG2 and BEL-7402 cells, even after incubation for 72 h at the maximum micelle concentration of 50 μM. SF micelles and free sorafenib showed concentration-dependent and time-dependent behavior. However, the cytotoxicity of SF micelles against HepG2 and BEL-7402 cells was lower than that of free sorafenib after 24, 48 and 72 h culture (C–H). Subsequently, we calculated the half-maximal inhibitory concentration (IC50) of free sorafenib and SF micelles after 24, 48 and 72 h of incubation (). The IC50 value of free sorafenib was higher than that of SF micelles against HepG2 and BEL-7402 cells at different time points. This difference was mainly due to the moderate release rate of sorafenib from the micelles after being taken up by the cells, thus reducing toxicity [Citation39].
Table 1. The half-maximal inhibitory concentration of free sorafenib and sorafenib-loaded PEG-poly (ε-caprolactone) micelles in HepG2 and BEL-7402 cells after 24, 48 and 72 h incubation.
In order to investigate the cell death mechanism after treatment with free sorafenib and SF micelles with a sorafenib concentration of 5 μM for 48 h, HepG2 and BEL-7402 cells were stained with Annexin V-FITC and PI, and the cell count of early or late apoptosis cells was measured. As shown in , we found that free sorafenib and SF micelles could induce early apoptosis and late apoptosis. However, it is worth noting that free sorafenib and SF micelles could induce more late apoptosis than early apoptosis. Compared with the free sorafenib, SF micelles could induce a higher proportion of apoptotic cells. The superior apoptosis induced by SF micelles was ascribed to the efficient cellular uptake of SF micelles and sustained release of sorafenib from SF micelles.
In vivo biodistribution & tumor penetrability
To explore the biodistribution of PEG-PCL micelles in vivo, DiR, a NIR dye was loaded into PEG-PCL micelles to visualize the behavior of micelles in vivo. FMI was used for real-time monitoring of the distribution of a probe in vivo with high sensitivity and high optical contrast. The FMI results suggested that the DiR micelles could significantly accumulate in the tumor tissue within 48 h after intravenous administration, confirming the intratumoral EPR effect. However, for free DiR, only very low fluorescence signals were observed at the tumor site (A & B). After 48 h, all HepG2-Luc tumor-bearing mice were ultimately sacrificed, and the major organs, including the heart, liver, spleen, lung and kidney, along with the tumors, were dissected for ex vivo fluorescence imaging (C). The average fluorescence intensity of DiR micelles in tumors was 6.67-times stronger than that of free DiR (D). The RES, including the liver and spleen, expressed a high uptake of nanoparticles, resulting in strong fluorescence signal in the liver and spleen.
(A) In vivo fluorescence images of the mice at different time points; and (B) quantitative analysis of fluorescence intensity at different points. The tumors were circled with a broken black line; (C)ex vivo fluorescence images of major organs (heart, liver, spleen, lung and kidney) and tumor tissues at 48 h postinjection; (D) quantitative analysis of fluorescence intensity of free DiR and DiR micelles in each organ and tumor. (E) The distribution and (F) quantitative analysis of photoacoustic signals from free DiR and DiR micelles in tumors at a depth of 4.5 mm at 24 h postinjection. The tumors were circled in red.
BLI: Bioluminescence imaging; DiR: 1,1′-Dioctadecyl-3,3,3′,3′-tetramethylindotricarbocyanine iodide; DiR micelles: DiR-loaded PEG-poly (ε-caprolactone) micelles; FMI: Fluorescence molecular imaging; PA: Photoacoustic.
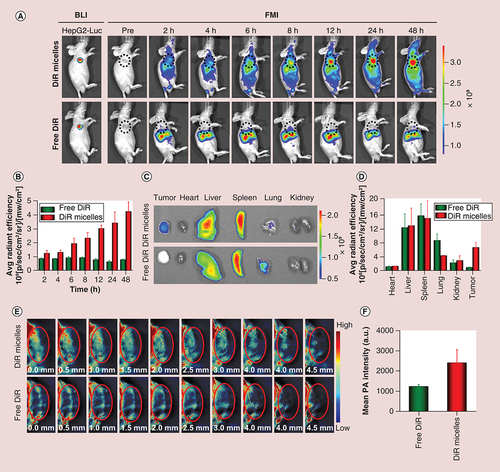
The tumor penetrability of SF micelles was investigated through PAI. Compared with FMI, PAI has attracted considerable attention in biomedical imaging owing to its high resolution and superior imaging depth. The photoacoustic signals of DiR micelles at the tumor site was detected using the multispectral optoacoustic tomographic imaging system. After the whole tumor tissue was scanned, a range of cross-sectional images with a depth of 4.5 mm was captured. More photoacoustic signals from DiR micelles were detected than those from free DiR (E & F). The signals of DiR micelles in different transverse sections of tumor tissues indicated uneven distribution. Moreover, SF micelles could penetrate into the interior of the tumor. In other words, more sorafenib could be delivered to the interior of the tumor tissue, thereby helping to improve its bioavailability and efficacy.
In vivo therapeutic effects
In vivo therapeutic effects of SF micelles were studied using HepG2-Luc tumor-bearing nude mice. The experimental mice were separated into three groups and were intravenously administered with PBS, free sorafenib or SF micelles. No obvious change was observed in the body weight of mice in the three groups during the treatment, implying that SF micelles possessed optimum biocompatibility (A). For the free sorafenib and SF micelle groups, sorafenib was administered at 5 mg/kg every 3 days. As illustrated in B & C, free sorafenib and SF micelle groups inhibited the tumor growth compared with PBS group to some extent after 21 days of treatment. Additionally, SF micelles exhibited highest anticancer activity and the tumor volume and tumor growth rate of the SF micelle group were significantly lower than those of free sorafenib group. In addition, the photograph (D) and tumor weight (E) of excised tumors are evidence of the fact that SF micelles could enhance the therapeutic effects of sorafenib.
Mice were treated intravenously with PBS, free sorafenib or SF micelles at a sorafenib dose of 5 mg/kg. (A) Body weights and (B) tumor growth curves of mice in the three groups at different time points during the 3-week treatment. (C) Tumor growth rates, (D) tumor images, (E) tumor weights and (F) H&E and TUNEL staining of tumor sections at the end of the treatment. Brown color indicates TUNEL-positive apoptotic cells. Data were given as mean ± standard deviation (n = 5).
*p < 0.05;
**p < 0.01.
H&E: Hematoxylin and eosin; PBS: Phosphate-buffered saline; SF micelle: Sorafenib-loaded PEG-poly (ε-caprolactone) micelle; TUNEL: Terminal deoxynucleotidyl transferase dUTP nick-end labeling.
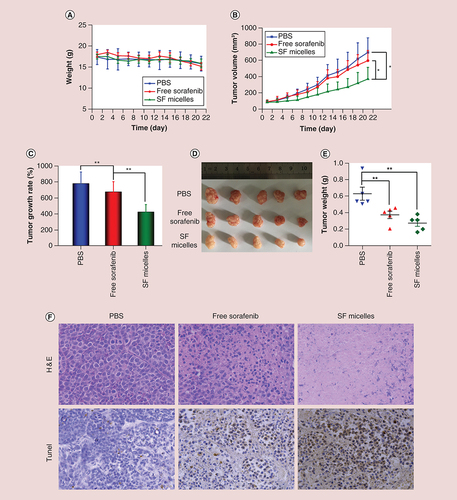
The therapeutic effects of SF micelles were further verified by histological examination of tumor sections at the end of the treatment. Hematoxylin and eosin staining showed that most tumor cells were damaged after treatment with SF micelles, whereas little or no damage to tumor cells was observed in the other two groups. Similarly, terminal deoxynucleotidyl transferase dUTP nick-end labeling (TUNEL) assays indicated that the highest level of TUNEL-positive apoptotic tumor cells was observed in the SF micelle group (F). The above results illustrated that SF micelles could improve the therapeutic effects of HCC compared with free sorafenib.
Discussion
Polymeric micelles, as a common form of drug delivery system, have attracted enormous attention in biomedical and nanotechnological fields in recent years [Citation40]. They have several outstanding advantages, such as structural stability, biocompatibility and biodegradability. Polymeric micelles have a core-shell structure and could load insoluble drugs into the hydrophobic core. In this study, we used FDA approved biocompatible polymer PEG and PCL to encapsulate sorafenib, which significantly improved the water solubility and bioavailability of sorafenib, and suppressed the growth of HepG2-Luc HCC tumors in nude mice. We prepared a series of SF micelles with different sorafenib/PEG-PCL mass ratios to choose the optimal formulation. In consideration of the size, PDI and DL, sorafenib/PEG-PCL (4/20) was chosen for the further study. SF micelles were soluble and stable in water, with suitable size, and high DL and EE. The low CMC value of PEG2k-PCL5k also insured the stability of SF micelles in blood circulation. Generally, nanoparticles smaller than 200 nm in size are more efficient in entering tumor tissue via EPR effect, and nanoparticles larger than 200 nm are quickly cleared from the bloodstream by the spleen [Citation41,Citation42]. Song et al. delved deeply into the optimal size of nanocarriers. They developed a series of nanoparticles with different sizes (40, 90, 130 and 180 nm). The 90 nm nanoparticles showed the highest antitumor efficacy, indicating that the nanoparticles with 90 nm or close to 90 nm could be the ideal size for drug delivery [Citation43]. Therefore, our prepared SF micelles with a size of 73.32 ± 0.12 nm were suitable for sorafenib delivery and they have a slight negative charge, which could prolong the circulation time in the blood [Citation44]. In addition, the SF micelles could be stable after a week storage at 4 and 37°C, of which the size, PDI and ζ-potential had changed little, and no participation and aggregation were observed during the storage time. The photographs of sorafenib in water and the Tyndall effect of SF micelles could intuitively indicate that SF micelles could form a homogeneous and stable solution in water, which could increase the solubility of sorafenib by 300-times.
Subsequently, in vitro cell experiments and in vivo imaging experiments were carried out to demonstrate that the polymer micelles could improve the bioavailability of sorafenib. We investigated the cellular uptake efficiency of PEG-PCL micelles, and the results suggested that more PEG-PCL micelles could be taken up by HCC cells, which meant that the polymeric micelles could enhance the uptake capacity of sorafenib. The antiproliferative activity of SF micelles was lower than that of free sorafenib after incubating for 24, 48 and 72 h. This result was consistent with the study on enhanced sorafenib delivery using poly(lactic-co-glycolic acid) nanoparticles, which was mainly due to the moderate release rate of sorafenib from the micelles after being taken up by the cells [Citation39]. We further explored the mechanism of cell death through apoptosis assays. In the apoptosis experiments, the proportion of living cells, early apoptosis cells, late apoptosis cells and dead cells were analyzed using FACS with Annexin V-FITC and PI [Citation45]. In our results, free sorafenib and SF micelles could induce more late apoptosis and little early apoptosis, while SF micelles could induce more cell apoptosis compared with free sorafenib at a sorafenib concentration of 5 μM. Moreover, DiR micelles were used as a dual-modality probe for in vivo FMI/PAI imaging to further investigate the metabolic behavior of PEG-PCL micelles in vivo and to check whether PEG-PCL micelles could passively target to the tumor site due to the EPR effect. A large number of PEG-PCL micelles accumulated and penetrated into the interior of the tumor tissues, allowing more sorafenib molecules to be carried into the tumor site. PEG-PCL micelles as a drug carrier increased the concentration of sorafenib in HCC cells and HCC tumor tissues in a targeted manner and improved the bioavailability of sorafenib.
Importantly, through in vivo antitumor experiments, the therapeutic effects were assessed based on the tumor volume changes, tumor growth rate and tumor weight at the end of the treatment. SF micelles exhibited higher tumor inhibition than free sorafenib and PBS. This was because SF micelles significantly increased the sorafenib concentration in the tumor tissues through the EPR effect, which had already been validated by the FMI and PAI imaging. Additionally, there was no obvious weight loss in the three groups, implying that all formulations showed almost no systemic toxicity at a sorafenib dose of 5 mg/kg. The therapeutic effects were further verified by hematoxylin and eosin staining and TUNEL assays. A large number of apoptotic cells appeared in the sections of SF micelles treated tumor, while there were a few of apoptotic cells observed in PBS and free sorafenib groups.
Conclusion
We successfully fabricated a biocompatible micelle to enhance the water-solubility of sorafenib, and further improve its bioavailability and curative effect. SF micelles had several advantages, including: higher biocompatibility and easy preparation; high sorafenib loading content, small size and narrow size distribution; high stability, ensuring that SF micelles maintained the micellar structure without decomposition; perfect solubility, and prolonged circulation in blood and minimal nonspecific cellular uptake by the RES due to the PEG shell; and higher tumor accumulation, effective intratumoral penetration and excellent curative effects. Considering these advantages, our study demonstrated the potential of SF micelles to serve as a therapeutic agent for treatment of advanced HCC.
Future perspective
PEG and PCL are biocompatible and both have been approved by FDA. In this study, SF micelles were prepared using a simple method. PEG-PCL micelles could improve sorafenib solubility, and enhance the concentration of sorafenib in tumor tissues. Consequently, SF micelles exhibited superior antitumor activity in vivo. Therefore, these conclusions reflect the clinical potential of SF micelles for the treatment of advanced HCC.
To improve the water-solubility and bioavailability of sorafenib, and further increase its therapeutic effect, sorafenib-loaded PEG-poly (ε-caprolactone) micelles (SF micelles) were prepared using a dialysis method.
SF micelles could form a homogeneous solution in water, increasing the solubility of sorafenib by 300-times.
SF micelles had a spherical structure with suitable size, and high drug loading and entrapment efficiency.
SF micelles could remain stable at 4 and 37°C for a week, ensuring its stability during storage at different temperatures and during circulation in blood throughout the body until reaching the tumor site.
The cellular uptake study showed that PEG-PCL micelles could enhance the uptake of sorafenib by the HepG2 and BEL-7402 cells, and further help to raise the bioavailability of sorafenib.
PEG-PCL micelles could enhance the accumulation and penetrability of sorafenib in the tumor tissues observed through fluorescence molecular imaging and photoacoustic imaging.
SF micelles could significantly inhibit tumor growth with good biocompatibility in vivo.
This study showed that SF micelles would be an effective carrier to deliver sorafenib for hepatocellular carcinoma therapy.
Authors’ contributions
Each co-author listed above participated sufficiently in the work to take responsibility for the content, and that all those who qualify are listed. Authorship credit should be based on: substantial contributions to the conception or design of the work, or the acquisition, analysis or interpretation of data for the work; drafting the work or revising it critically for important intellectual content; final approval of the version to be published; and agreement to be accountable for all aspects of the work in ensuring that questions related to the accuracy or integrity of any part of the work are appropriately investigated and resolved. In addition to the listed co-authors, M Zhan contributed to the experimental design. W Shang and S Fu assisted with revising the work critically for important intellectual content.
Ethical disclosure
The authors state that they have obtained appropriate institutional review board approval or have followed the principles outlined in the Declaration of Helsinki for all human or animal experimental investigations. In addition, for investigations involving human subjects, informed consent has been obtained from the participants involved.
Supplemental Text 1
Download MS Word (18.3 KB)Supplementary data
To view the supplementary data that accompany this paper please visit the journal website at:www.tandfonline.com/doi/full/10.2217/epi-2016-0184
Financial & competing interests disclosure
This study is supported by the National Key Research and Development Program of China under Grant number 2017YFA0205200, 2016YFC0103702 and 2016YFA0201401, the National Natural Science Foundation of China under Grant number 81571785, 81771957, 81227901, 61231004, 61671449, 21604025, 51273072 and 81701771, the Natural Science Foundation of Guangdong Province, China under Grant number 2016A030311055, 2016A030313770, 2014B090907004 and 2016A030310461, Science and Technology Foundation of Guangdong Province, China under Grant number 2013B021800180. The authors have no other relevant affiliations or financial involvement with any organization or entity with a financial interest in or financial conflict with the subject matter or materials discussed in the manuscript apart from those disclosed.
No writing assistance was utilized in the production of this manuscript.
References
- Maluccio M Covey A . Recent progress in understanding, diagnosing, and treating hepatocellular carcinoma . CA Cancer J. Clin.62 ( 6 ), 394 – 399 ( 2012 ).
- Torre LA Bray F Siegel RL Ferlay J Lortet-Tieulent J Jemal A . Global cancer statistics, 2012 . CA Cancer J. Clin.65 ( 2 ), 87 – 108 ( 2015 ).
- Siegel RL Miller KD Jemal A . Cancer statistics, 2018 . CA Cancer J. Clin.68 ( 1 ), 7 – 30 ( 2018 ).
- Forner A Llovet JM Bruix J . Hepatocellular carcinoma . The Lancet379 ( 9822 ), 1245 – 1255 ( 2012 ).
- Liu C Chen Z Chen Y et al. Improving oral bioavailability of sorafenib by optimizing the ‘spring’ and ‘parachute’ based on molecular interaction mechanisms . Mol. Pharm.13 ( 2 ), 599 – 608 ( 2016 ).
- Ranieri GG-CG Goffredo V Patruno R et al. Sorafenib (BAY 43–9006) in hepatocellular carcinoma patients: from discovery to clinical development . Curr. Med. Chem.19 , 938 – 944 ( 2012 ).
- Ganten TM Stauber RE Schott E et al. Sorafenib in patients with hepatocellular carcinoma-results of the observational INSIGHT study . Clin. Cancer Res.23 ( 19 ), 5720 – 5728 ( 2017 ).
- Potenza N Mosca N Zappavigna S et al. MicroRNA-125a-5p is a downstream effector of sorafenib in its antiproliferative activity toward human hepatocellular carcinoma cells . J. Cell Physiol.232 ( 7 ), 1907 – 1913 ( 2017 ).
- Cheng AL Kang YK Chen ZD et al. Efficacy and safety of sorafenib in patients in the Asia–pacific region with advanced hepatocellular carcinoma: a Phase III randomised, double-blind, placebo-controlled trial . Lancet Oncol.10 ( 1 ), 25 – 34 ( 2009 ).
- Josep M. Llovet Sergio Ricci Vincenzo Mazzaferro et al. Sorafenib in advanced hepatocellular carcinoma . N. Engl. J. Med.359 ( 4 ), 378 – 390 ( 2008 ).
- Lang L . FDA approves sorafenib for patients with inoperable liver cancer . Gastroenterology134 ( 2 ), 379 ( 2008 ).
- Ma MKF Lau EYT Leung DHW et al. Stearoyl-CoA desaturase regulates sorafenib resistance via modulation of ER stress-induced differentiation . J. Hepatol.67 ( 5 ), 979 – 990 ( 2017 ).
- Xiao YA Liu YJ Yang SM et al. Sorafenib and gadolinium co-loaded liposomes for drug delivery and MRI-guided HCC treatment . Colloids Surf. B Biointerfaces141 , 83 – 92 ( 2016 ).
- Zhao R Li T Zheng G Jiang K Fan L Shao J . Simultaneous inhibition of growth and metastasis of hepatocellular carcinoma by co-delivery of ursolic acid and sorafenib using lactobionic acid modified and pH-sensitive chitosan-conjugated mesoporous silica nanocomplex . Biomaterials143 , 1 – 16 ( 2017 ).
- Silva JO Fernandes RS Lopes SC et al. pH-sensitive, long-circulating liposomes as an alternative tool to deliver doxorubicin into tumors: a feasibility animal study . Mol. Imaging Biol.18 ( 6 ), 898 – 904 ( 2016 ).
- Feng L Gao M Tao D et al. Cisplatin-prodrug-constructed liposomes as a versatile theranostic nanoplatform for bimodal imaging guided combination cancer therapy . Adv. Funct. Mater.26 ( 13 ), 2207 – 2217 ( 2016 ).
- Gupta L Sharma AK Gothwal A et al. Dendrimer encapsulated and conjugated delivery of berberine: a novel approach mitigating toxicity and improving in vivo pharmacokinetics . Int. J. Pharm.528 ( 1–2 ), 88 – 99 ( 2017 ).
- Wei T Chen C Liu J et al. Anticancer drug nanomicelles formed by self-assembling amphiphilic dendrimer to combat cancer drug resistance . Proc. Natl Acad. Sci. USA112 ( 10 ), 2978 – 2983 ( 2015 ).
- Zhu MH Chen SC Hua LB et al. Self-targeted salinomycin-loaded DSPE-PEG-methotrexate nanomicelles for targeting both head and neck squamous cell carcinoma cancer cells and cancer stem cells . Nanomedicine12 ( 4 ), 295 – 315 ( 2017 ).
- Yu L Lin C Zheng Z Li Z Wang X . Self-assembly of pH-responsive biodegradable mixed micelles based on anionic and cationic polycarbonates for doxorubicin delivery . Colloids Surf. B Biointerfaces145 , 392 – 400 ( 2016 ).
- Yao X Niu X Ma K et al. Graphene quantum dots-capped magnetic mesoporous silica nanoparticles as a multifunctional platform for controlled drug delivery, magnetic hyperthermia, and photothermal therapy . Small13 ( 2 ), 1602225 ( 2017 ).
- Wang HY Zhang MJ Zhang LY et al. Near-infrared light and pH-responsive Au@carbon/calcium phosphate nanoparticles for imaging and chemo-photothermal cancer therapy of cancer cells . Dalton Trans.46 ( 43 ), 14746 – 14751 ( 2017 ).
- Xu X Ho W Zhang X Bertrand N Farokhzad O . Cancer nanomedicine: from targeted delivery to combination therapy . Trends Mol. Med.21 ( 4 ), 223 – 232 ( 2015 ).
- Jin H Pi J Zhao Y et al. EGFR-targeting PLGA-PEG nanoparticles as a curcumin delivery system for breast cancer therapy . Nanoscale9 ( 42 ), 16365 – 16374 ( 2017 ).
- Jin Y Ma X Zhang S et al. A tantalum oxide-based core/shell nanoparticle for triple-modality image-guided chemo-thermal synergetic therapy of esophageal carcinoma . Cancer Lett397 , 61 – 71 ( 2017 ).
- Yang YC Cai J Yin J Zhang J Wang KL Zhang ZT . Heparin-functionalized pluronic nanoparticles to enhance the antitumor efficacy of sorafenib in gastric cancers . Carbohydr. Polym.136 , 782 – 790 ( 2016 ).
- Depalo N Iacobazzi RM Valente G et al. Sorafenib delivery nanoplatform based on superparamagnetic iron oxide nanoparticles magnetically targets hepatocellular carcinoma . Nano Res.10 ( 7 ), 2431 – 2448 ( 2017 ).
- Grossen P Witzigmann D Sieber S Huwyler J . PEG-PCL-based nanomedicines: a biodegradable drug delivery system and its application . J. Control. Rel.260 , 46 – 60 ( 2017 ).
- Cui C Yu P Wu M et al. Reduction-sensitive micelles with sheddable PEG shells self-assembled from a Y-shaped amphiphilic polymer for intracellular doxorubicine release . Colloids Surf. B Biointerfaces129 , 137 – 145 ( 2015 ).
- Li D-D Ma Y Du J et al. Tumor acidity/NIR controlled interaction of transformable nanoparticle with biological systems for cancer therapy . Nano lett.17 ( 5 ), 2871 – 2878 ( 2017 ).
- Wang Q Jiang J Chen W Jiang H Zhang Z Sun X . Targeted delivery of low-dose dexamethasone using PCL-PEG micelles for effective treatment of rheumatoid arthritis . J. Control. Rel.230 , 64 – 72 ( 2016 ).
- Lin W Yao N Qian L et al. pH-responsive unimolecular micelle-gold nanoparticles-drug nanohybrid system for cancer theranostics . Acta Biomater.58 , 455 – 465 ( 2017 ).
- Yao C Liu J Wu X et al. Reducible self-assembling cationic polypeptide-based micelles mediate co-delivery of doxorubicin and microRNA-34a for androgen-independent prostate cancer therapy . J. Control. Rel.232 , 203 – 214 ( 2016 ).
- Mi Y Mu C Wolfram J et al. A micro/nano composite for combination treatment of melanoma lung metastasis . Adv. Healthc. Mater.5 ( 8 ), 936 – 946 ( 2016 ).
- Zhang J Wang TQ Mu SJ Olerile LD Yu XY Zhang N . Biomacromolecule/lipid hybrid nanoparticles for controlled delivery of sorafenib in targeting hepatocellular carcinoma therapy . Nanomedicine12 ( 8 ), 911 – 925 ( 2017 ).
- Yang SM Zhang B Gong XW Wang TQ Liu YJ Zhang N . In vivo biodistribution, biocompatibility, and efficacy of sorafenib-loaded lipid-based nanosuspensions evaluated experimentally in cancer . Int. J. Nanomed.11 , 15 ( 2016 ).
- Hu Q Gao X Kang T et al. CGKRK-modified nanoparticles for dual-targeting drug delivery to tumor cells and angiogenic blood vessels . Biomaterials34 ( 37 ), 9496 – 9508 ( 2013 ).
- Thapa RK Choi JY Poudel BK et al. Multilayer-coated liquid crystalline nanoparticles for effective sorafenib delivery to hepatocellular carcinoma . ACS Appl. Mater. Interfaces7 ( 36 ), 20360 – 20368 ( 2015 ).
- Lin Ts T Gao DY Liu YC et al. Development and characterization of sorafenib-loaded PLGA nanoparticles for the systemic treatment of liver fibrosis . J. Control. Rel.221 , 62 – 70 ( 2016 ).
- Nishiyama N Matsumura Y Kataoka K . Development of polymeric micelles for targeting intractable cancers . Cancer Sci.107 ( 7 ), 867 – 874 ( 2016 ).
- Xu Y Liang X Bhattarai P et al. Enhancing therapeutic efficacy of combined cancer phototherapy by ultrasound-mediated in situ conversion of near-infrared cyanine/porphyrin microbubbles into nanoparticles . Adv. Funct. Mater.27 ( 48 ), 1704096 ( 2017 ).
- Wu C Chen H Wu X et al. The influence of tumor-induced immune dysfunction on immune cell distribution of gold nanoparticles in vivo . Biomater. Sci.5 ( 8 ), 1531 – 1536 ( 2017 ).
- Liang S Yang X-Z Du X-J et al. Optimizing the size of micellar nanoparticles for efficient siRNA delivery . Adv. Funct. Mater.25 ( 30 ), 4778 – 4787 ( 2015 ).
- Gu G Xia H Hu Q et al. PEG-co-PCL nanoparticles modified with MMP-2/9 activatable low molecular weight protamine for enhanced targeted glioblastoma therapy . Biomaterials34 ( 1 ), 196 – 208 ( 2013 ).
- Zhu X Sun Y Chen D et al. Mastocarcinoma therapy synergistically promoted by lysosome dependent apoptosis specifically evoked by 5-Fu@nanogel system with passive targeting and pH activatable dual function . J. Control. Rel.254 , 107 – 118 ( 2017 ).