Abstract
This paper reviews the uses of magnetism and ultrasound in therapeutic delivery applications. Emphasis is placed upon magnetic nanoparticles and microbubble ultrasound contrast agents. The underlying physical principles, history, key developments and limitations of these techniques for drug and gene delivery in vitro and in vivo are explored. The combination of ultrasonic and magnetic techniques is also reviewed with particular focus on magnetic microbubbles as delivery agents with the potential to combine the advantages of both methods whilst addressing many of their limitations. Finally, results are presented from a study of a new magnetic microbubble formulation which shows great applicability as a therapeutic delivery vehicle.
Introduction
The rapid advances in the development of new types of pharmaceutical product have greatly increased demand for more effective delivery systems Citation[1]. In addition, new formulations and delivery methods for existing products are increasingly being sought to mitigate the impact of patent expiration, the so called “patent cliff” Citation[2]. In 2008 the US demand for drug delivery systems was predicted to increase by more than 10% per year reaching $132 billion by 2012 Citation[3]. Conventional delivery methods such as injections and oral administration have significant advantages in terms of convenience and cost, but are unsuitable for several classes of therapeutic compound. For example, administration of poorly soluble drugs and large molecules such as proteins, including DNA, via intravenous or oral routes is largely ineffective Citation[1]. Moreover, systemic administration results in the exposure of all tissues and this can lead to harmful side effects Citation[4]. The most well known examples are perhaps those relating to cancer chemotherapy and compounds such as Paclitaxel, which inhibits the proliferation of cancer cells but is often associated with neutropenia and nausea Citation[5].
A successful drug delivery system should provide maximum therapeutic efficacy with minimal unwanted side effects Citation[6]. To do so it must: (i) protect the therapeutic material to prevent unwanted degradation during its passage through the body, (ii) concentrate the material at the desired location(s) and (iii) promote uptake in the target tissue. The concept of targeted drug delivery was originally put forward in the literature by Paul Erlich (1854–1915) who theorised that if malignant tissue could be selectively targeted then a toxin could also be selectively delivered in a manner akin to a “magic bullet” Citation[7]. The central principle of drug targeting is that the distribution of drug within an organism is manipulated in such a manner that the majority interacts exclusively with the target tissue Citation[8].
The mechanism by which the drug is released at the target site can be active or passive. Passive drug delivery involves diffusion from the drug carrier into the surrounding medium via natural metabolic processes. It takes advantage of human physiology or immune system response to maintain the drug at a given location within the body. The rate of release may be controlled, for example, through appropriate use of a biodegradable polymer to encapsulate the drug Citation[3]. Active drug targeting involves triggering or stimulating the release of the therapeutic compound by exploiting some characteristic of the carrier. This provides much more control over drug distribution and as such is more widely applicable. The stimulus can be the overexpression of receptors on tumour cells or a physical stimulus such as temperature, pH, light, pressure/sound, electric or magnetic fields Citation[3], Citation[9]. Localised drug release must be followed by uptake in the target tissue for successful treatment. For example, for gene delivery to be effective, the gene must reach the cell nucleus in order to be expressed Citation[10]. Uptake may again be achieved passively, e.g. through normal membrane transport processes, or promoted by application of the same or different physical stimuli.
Numerous strategies, from light sensitive polymeric carriers to viruses Citation[3], Citation[11], have been proposed for therapeutic delivery and the development of delivery systems represents a significant research field in its own right. This aim of this paper is to examine two of these strategies, namely ultrasound mediated and magnetically targeted drug delivery, and the combination of both techniques.
Magnetic targeting utilises an externally applied magnetic field to control the location of a magnetically responsive drug carrier in vivo, thereby avoiding many of the disadvantages of systemic administration Citation[12], Citation[13]. As will be explained in the next section, however, the types of magnetic particle suitable for use in vivo cannot be successfully retained in some parts of the body due to their small size Citation[14]. Ultrasound, widely used in imaging on account of its low cost and high patient acceptability, is finding increasing application in therapy. Its ability to be focused into very small volumes provides an excellent means of localising a therapeutic effect Citation[15]. In addition there is strong evidence that exposure to ultrasound at moderate intensities produces temporary and reversible permeabilisation of cell membranes Citation[16]. This “sonoporation” can be significantly amplified by the presence of microbubbles Citation[17], Citation[18]. Originally microbubbles stabilised by a polymeric or surfactant coating were developed as contrast agents for ultrasound imaging, but they have since been intensively investigated as vehicles for drug delivery. In this respect they have a significant advantage over magnetic particles since they can carry much larger payloads. Currently, however, successful methods for concentrating microbubbles at a target site in vivo are lacking and the last part of this paper will examine recent work in the development of magnetic microbubbles and new insights into a magnetic microbubble formulation which has shown excellent transfection efficiency.
Magnetism and drug delivery
The first documented medical application of magnets dates back to the 17th century when magnets were used to remove fragments of iron from the body Citation[19]. In the present day, in addition to their now widespread use in magnetic resonance imaging (MRI), magnetic fields are utilised in several therapeutic applications. This section examines their use in magnetic drug targeting.
Types of magnetic materials
When a magnetic material is placed within a magnetic field the individual atomic moments of the material contribute to the overall response or magnetic induction. The response of a given material to a magnetic field varies with temperature and atomic structure. This can be classified in terms of magnetic volumetric susceptibility, χ Citation[4]. Paramagnetic materials (χ > 0) magnetise very weakly in the direction of the magnetic field whereas diamagnetic materials (χ < 0) magnetise in the opposite direction Citation[20]. Due to the disordered structure of their magnetic moments, neither of these types of material show spontaneous magnetisation in the absence of an external field. Other materials exhibit internal ordered states which remain ordered after the removal of the magnetic field and these include ferri, ferro or antiferromagnets, where the prefix refers to the nature of the coupling interaction between the electrons in the material.
Due to remanent magnetisation, ferri and ferromagnetic materials tend to aggregate. This remanent magnetisation occurs because once a ferro or ferrimagnetic material is magnetically saturated in one direction, the moments tend to preserve the so-induced orientation to a certain extent, depending on the internal structure. This can be seen in a hysteresis curve. The resulting aggregation is undesirable in most biomedical applications.
Some of the interest in using superparamagnetic particles in biomedical applications is due to the fact that they do not exhibit remanent magnetisation. The internal structure of bulk magnetic materials is divided into magnetic domains, grouping moments with the same orientation. Below a certain size, however, formation of domains becomes energetically unfavourable, resulting in particles consisting of a single domain state where all the magnetic moments of the particle are aligned parallel to each other Citation[21]. For single domain particles below a certain critical size – often regarded as the superparamagnetic limit – the magnetic moment of the particle is free to fluctuate in response to thermal energy in the absence of a magnetic field. Therefore these single domain nanoparticles enter into a superparamagnetic regime. Superparamagnetic materials thus provide a compromise between strong magnetisation and avoidance of particle aggregation Citation[20].
Magnetic nanoparticles
As a consequence of the phenomena discussed above, superparamagnetic nanoparticles have become the subject of intensive research in recent years. They have three key additional features making them attractive for biomedical applications: their ability to be visualised under MRI Citation[12], to be localised at a target site by means of a magnetic field gradient Citation[4] and to produce localised heating when exposed to an oscillating magnetic field for hyperthermia and/or to promote drug delivery Citation[22–24].
Ferrimagnetic iron oxides, maghemite and magnetite, which are able to form superparamagnetic iron oxide nanoparticles (SPIONs) typically below 15 nm, are currently in use as MRI contrast agents Citation[13]. Iron oxides are relatively safe compared to other substances used as MRI contrast agents such as gadolinium Citation[25]. The nanoscale dimensions of the particles allow them to pass not only through the narrowest blood vessel but even through cell membranes Citation[26]. To avoid uptake by the reticuloendothelial system (RES), magnetic carriers typically have two structural configurations; a magnetic core coated by a biocompatible polymer or magnetic nanoparticles inside a porous biocompatible polymer matrix into which drugs, genes or targeting agents can also be incorporated Citation[4], Citation[20], Citation[27]. According to Neuberger et al. magnetic particles smaller than 4 µm are removed by RES cells mainly in the liver and spleen Citation[7], Citation[28]. In general, however, the larger the particle the shorter its plasma half-life Citation[20].
Drug delivery applications
The use of magnetic particles in drug delivery appeared in the literature in 1960 when Freeman et al. proposed that magnetic nanoparticles could be transported through the vasculature and concentrated in a specific part of the body with the aid of a magnetic field Citation[19]. In 1963 Meyers described how magnetic iron particles in the lymphatic and vascular system of a dog could be used as a contrast and isotopic agent Citation[29], Citation[30]. Zimmerman and Pilwat used magnetic erythrocytes (red blood cells) for the delivery of cytotoxic drugs in 1976 Citation[31]. In the 1970s Senyei et al. produced albumin microspheres, incorporating superparamagnetic magnetite, and successfully retained these against in vivo capillary flow conditions using a magnetic field Citation[32], Citation[33]. Widder et al. used magnetic albumin microspheres containing doxorubicin and showed tumour regression in rats when a localised magnetic field was applied and with no occurrences of embolism observed Citation[34].
Novel drug delivery systems have also been developed using polymer matrices containing drugs and magnetic beads. This pulsatile delivery system opens pores to release the drug when an oscillating magnetic field is applied Citation[1], Citation[35]. In 1994 Hafeli et al. prepared biodegradable poly(lactic acid) microspheres that incorporated magnetite and a beta emitter for targeted radiotherapy and successfully applied it to subcutaneous tumours Citation[36], Citation[37]. A ferrofluid (fluid containing a magnetic suspension, in this case magnetic nanoparticles) comprising drugs was used successfully in an in vivo mouse model by Lubbe et al. Citation[38] as an anti-tumour agent with no toxic effects observed. Another successful trial concentrated the ferrofluid Chemicell, bound to mitoxantrone, in murine tumours with the application of a magnetic field Citation[39]. The first phase I clinical trial was performed by Lubbe et al. Each patient tolerated the ferrofluid and the nanoparticles were seen to be concentrated at tumours after the application of a magnetic field Citation[9]. A second phase I/II trial was conducted by a start-up company, FeRx Inc, in 1997 where Carbon coated iron particles (0.5–5 µm) which had adsorbed doxorubicin were localised and retained in target sites Citation[40]. A full review of recent magnetic drug delivery trials in patients may be found in McBain et al. Citation[13].
Gene delivery applications
Magnetic nanoparticles also hold great potential for gene therapy. DNA delivery to cells can induce production of therapeutic proteins to treat malignancies. Use of short interfering RNA can temporarily down-regulate the expression of a gene of choice (gene silencing) and potentially any disease causing gene can be silenced Citation[41]. If simply injected or ingested, however, DNA can degrade and is not able to pass through cellular barriers Citation[42]. There are three primary ways to deliver genes into cells: through the use of viral vectors which is highly efficient but which can have unwanted side effects; electroporation, in which cells are electrically stimulated to promote uptake, which is again very efficient but causes a large number of cell deaths; and non-viral transfection agents which assist nucleic acid entry into cells through other mechanisms and maintain cell viability but tend to be inefficient Citation[25].
The first study of magnetically targeted DNA delivery was performed by Mah et al. in 2000 Citation[43]. A recombinant adeno-associated virus (rAAV), encoding for a green fluorescent protein was coated onto the surface of magnetic microspheres. Under the influence of an applied magnetic field, 1% of the vector-microsphere formulation gave the same transfection efficiency as 100% free vector. This was continued by Hughes et al. to produce “infectious, paramagnetic, retroviral vector particles” using paramagnetic streptavidin particles conjugated to antibodies which the retrovirus associated with Citation[44].
Non-viral vectors are also being developed with an emphasis upon improving nucleic acid transfection efficiency. The association of genes with magnetic nanoparticles for magnetically guided nucleic acid delivery has been termed magnetofection Citation[45]. The principle is similar to that of other transfection agents, such as lipofectine 2000, except plasmid DNA forms complexes with a cationic polymer – usually polyethylenimine (PEI) – which is able to bind and condense DNA coated magnetic nanoparticles to create a “magnetoplex” with magnetically controllable behaviour Citation[46]. The mechanism by which the gene enters the cell with magnetoplexes has so far been found to be no different to that associated with normal transfection reagents Citation[14], Citation[45], Citation[47].
Other novel approaches include that adopted by Cai et al. who used nickel embedded carbon nanotubes coated with DNA to act as “spears” to pierce the cells resulting in high transfection efficiency Citation[48]. Oz Biosciences has designed four different cationic nanoparticle formulations designed for specific applications in regard to gene delivery Citation[49]. Improvements are also being looked into through the use of pulsating fields where Kamau et al. showed an increase in the number of transfected cell relative to static fields Citation[50].
Limitations
Magnetic drug targeting is subject to two main constraints. First, there may be rapid clearance of particulate drug carriers from the circulation. Second, the strength and gradient of the magnetic field that needs to be applied, given the small size of the nanoparticles and hence their reduced magnetic strength, may be very high for successful localisation of a carrier Citation[28] against the hydrodynamic forces produced e.g. by blood flow Citation[4]. Hence there is a demand for magnetic particles with larger diameters but that are still capable of travelling through capillaries without posing a risk of embolism Citation[14]. Set against this, however, is the reduced ability of larger particles to penetrate tissue and cell membranes.
Ultrasound and drug delivery
One of the first investigations into the therapeutic use of ultrasound for drug delivery was performed in the 1950s by Fellinger and Schmid who used ultrasound to enhance the permeation of ointment into inflamed tissue, successfully treating polyarthritis Citation[51]. The first ultrasound assisted transfection in vitro was reported in 1987 when Fechheimer et al. reported low level transfection of murine fibroblasts when exposed to 20 kHz ultrasound from a sonicator Citation[52]. This was followed by the use of ultrasound to assist drug uptake by tumours in mouse models in vivo Citation[53].
Basic physical principles
Ultrasound can be defined as “acoustic waves propagating within a medium at frequencies exceeding the auditory band” Citation[54]. In diagnostic imaging, it is the scattering of these waves from regions of differing density and compressibility which is used to produce the images. In addition to being scattered, however, ultrasound undergoes three other types of interaction with tissue and it is these which underlie its effect in therapeutic applications. First, a fraction of the energy in the propagating wave is also absorbed by tissue, resulting in local heating. If the intensity is sufficient, tissue ablation will occur through protein denaturation and/or boiling Citation[15], Citation[55], Citation[56]. At lower intensities, effects such as increased perfusion and/or stimulated release from drug delivery vehicles may be realised Citation[55]. Second, ultrasound may produce cavitation. Acoustic cavitation is defined as the formation and activity of gas and/or vapour filled bubbles, of natural or artificial origin, in a medium exposed to sound Citation[57]. The motion of the bubbles can temporarily increase tissue and individual cell membrane permeability Citation[58], termed “sonoporation,” including temporary opening of the blood brain barrier Citation[59]. At higher intensities it can contribute to cell death through a variety of mechanisms associated with the violent collapse of the bubbles. Third, transfer of momentum from the ultrasound wave to the liquid produces streaming motions and associated shear stresses on tissue interfaces. In the presence of a bubble oscillating under ultrasound excitation, microstreaming Citation[60] occurs through a similar mechanism and this may in fact be one of the main contributors to sonoporation Citation[61], Citation[62].
Microbubbles
Microbubbles are widely used in ultrasound imaging as contrast agents Citation[63], Citation[64]. They consist of a gas core surrounded by a coating normally of a lipid, protein or polymer. The coating reduces surface tension and inhibits gas diffusion, thereby increasing the lifetime of the microbubble Citation[65]. The high compressibility of the microbubbles compared with the surrounding tissue gives rise to a large acoustic impedance difference and causes the bubbles to undergo volumetric oscillations producing nonlinear acoustic radiation at moderate ultrasound intensities Citation[64]. It is these phenomena which provide the contrast enhancement between regions with and without bubbles in ultrasound images Citation[66], Citation[67]. Microbubbles are also finding application in therapeutic applications, however. They can be used as nuclei to increase cavitation activity Citation[57] and as vehicles for drug delivery/gene therapy by incorporation of therapeutic material into their coatings which can subsequently be released by destroying the microbubbles with a high amplitude ultrasound pulse Citation[68], Citation[69].
Drug delivery applications
Ultrasound has been shown to increase the thrombolytic efficacy of urokinase Citation[70]. This is most likely due to localised cavitation which can weaken the clot surface and/or improve penetration of the drug; and this process has been shown to be enhanced by the presence of microbubbles Citation[71], Citation[72]. Similarly, exposure to ultrasound in the presence of microbubbles has been shown to reversibly produce pores on the surfaces of cells Citation[6], Citation[73]. Unger et al. obtained similar results with microbubbles containing a drug filled layer of soy bean oil and the drug paclitaxel Citation[69]. Guzmen et al. quantified molecular uptake of celcein in prostate cancer and aortic smooth muscle cells, examining the heterogeneity of cavitation and factors that influence drug uptake Citation[74], Citation[75]. Microbubbles have also been used with cells for uptake of Doxorubicin and colloidal particles.Citation[76], Citation[77]
Targeting
Albumin and lipid coated microbubbles have been found to persist in areas of inflammation. This is due to leukocytes, from an inflammatory response, attaching to the microbubble Citation[78]. Attaching targeting agents to the surface of microbubbles should allow them to be retained at other malignant sites within the body and as such, microbubbles with monoclonal antibodies attached have been formulated Citation[79]. The radiation force from a propagating ultrasound wave can translate a microbubble and assist in targeting Citation[55]. It has also been shown that ultrasound radiation force is able to deposit nanoparticles bound to microbubbles against the side of a capillary tube under flow conditions Citation[80].
Gene delivery applications
In 1996 Kim et al. used ultrasound to transfect a variety of cells with plasmid DNA. They found that the ultrasound power, duration, ambient pressure and the occurrence of cavitation were critical and all positively correlated with transfection efficiency Citation[81]. The same authors also injected albumin microbubbles with plasmid DNA into rat knees and observed transfection under ultrasound Citation[81]. Greenleaf et al., continuing the work by Kim et al., demonstrated an increase in the transfection rate of DNA in vitro with ultrasound in the presence of Albumin bubbles Citation[82]. Lawrie et al. reported that exposure to ultrasound in the presence of Optison® achieved approximately 300 fold higher transgene expression in vitro than plasmid DNA alone Citation[83]. However low transfection efficiency still remains a major limitation and the same mechanisms that cause drug uptake can also lead to cell death Citation[84]. The physical parameters affecting gene delivery with ultrasound and microbubbles were investigated by Rahim et al. who determined optimal combinations of pulse amplitude, exposure time and bubble concentration Citation[85]. Matsumura's group has administered gelatin coated microspheres binding a gene to rat livers with the application of ultrasound Citation[86]. Transfection with microbubbles and ultrasound has been performed in the lungs and hearts of mice and the hearts of rats Citation[17], Citation[87], Citation[88]. Comparisons between different ultrasound contrast agents have been performed in vivo to find which gave the best cellular uptake of genes Citation[84], Citation[89].
Conjugation strategies
Microbubbles can be used to improve cell permeation if the drug is co-administered at the target location avoiding the problems of attachment, although this limits efficacy to malignancies reached by injection Citation[86]. As the gas core of a microbubble cannot hold organic compounds and the lipid coating is thought to be too thin, therapeutic compounds must be attached to the shell Citation[16]. Electrostatic interactions allow charged drugs to be bound to the surface of cationic lipid coated microbubbles. DNA is a polyanion, due to the negative sugar phosphate groups on the molecule, and as such easily binds to cationic microbubbles. Drugs can also be incorporated into microbubbles in a layer of oil beneath the lipid outer layer and this can further stabilise the outer surface of microbubbles Citation[86]. Lum et al. bound polystyrene nanoparticles to the microbubble shell through an avidin-biotin “bridge” which could be replaced by nanoparticles loaded with genes or drugs Citation[80]. Liposomes have also been loaded onto microbubbles Citation[90] and Cochrane et al. produced Paclitaxel and Doxurubicin loaded polymer microbubbles which cavitate under ultrasound forming polymer nanoparticles which enter cells and slowly release drugs Citation[91].
Limitations
Close proximity between microbubbles and target cells is required for therapeutic effects to be realised at moderate ultrasound intensities and without unwanted damage Citation[85]. The concentration of microbubbles at a target site needs to be optimised as adequate numbers are needed both for sonoporation and to achieve the required dose of a drug; but increased microbubble concentration poses a potential risk of embolism and may also produce excessive ultrasound scattering or “shielding” preventing exposure of the target tissue. Currently, repeatable, efficient bio-chemical targeting of microbubbles is still proving to be a challenge in vivo Citation[92]. Surface architecture designed to maximise ligand exposure to the target tissue also runs the risk of boosting presentation of an immunogenic compound that leads to early particle clearance or worse a hypersensitivity response Citation[16].
Ultrasound and magnetism
As described above, magnetic and ultrasonic drug delivery have both been examined and show great potential but both suffer from certain limitations. These limitations can to some extent be addressed by combining the two techniques and the remainder of this review will examine developments in this field.
High intensity focused ultrasound surgery
High intensity focused ultrasound (HIFU) and MRI, were combined in 1991 with the latter used for the purposes of treatment monitoring. Since then, both animal experiments and human trials have been performed. A commercial device has been created and FDA approved, with further clinical systems currently undergoing trials Citation[93]. In 1993, a lipid coated microbubble incorporating paramagnetic particles was patented for use as an ultrasound and MRI contrast agent. It was also proposed as an agent for mediating therapeutic effects through cavitation nucleation Citation[94]. More recently Smith et al. showed that magnetite nanoparticle agglomerates could be used to promote cavitation in HIFU treatments which could be used for drug release Citation[95].
Stimulated release
Ultrasound has also been used to improve drug delivery from magnetic particles. Saravan et al. found that the application of ultrasound enhanced the release kinetics of gelatin magnetic microspheres Citation[96]. Kaminski et al. (2008) showed that ultrasound increased enzyme release from magnetic microcarriers, but temperature rises within the carrier could denature the enzyme Citation[97].
Magnetic microbubbles
Magnetic nanoparticles can be incorporated into microbubbles in order to form magnetic microbubbles. These have been synthesised for use in ultrasound and magnetically mediated drug delivery and as dual purpose MRI and ultrasound contrast agents.
Magnetic microbubbles as dual contrast agents
Even in the absence of magnetic nanoparticles, the gas-liquid interface in microbubbles induces large local magnetic susceptibility differences. This was demonstrated experimentally in vivo with Optison® microbubbles in rat liver Citation[98]. This then prompted in 2008 the use of emulsion stabilised particles of varying size with inclusions of superparamagnetic iron oxide nanoparticles as dual modality (MRI and Ultrasound) contrast agents. The SPION loaded bubbles were shown to improve the contrast of both ultrasound and MR images Citation[99]. Yang et al. improved upon this by preparing a larger microbubble construct loaded with both gas and SPIONs Citation[100]. Park et al. also produced stable microbubbles to which could be attached numerous nanoparticles, magnetic and non-magnetic, for use in image contrast enhancement Citation[101].
Magnetic microbubbles for drug delivery
In 2000, Soetanto et al. attached magnetic micro-particles to microbubbles to enable remote manipulation of the microbubbles for both imaging and therapeutic applications. The microbubbles were formed from charged stearates and the microparticles were also coated in charged stearate and a calcium ion was used to bind them to the microbubble. The magnetic microbubbles responded to a magnet with a 2 kg equivalent force Citation[102], Citation[103]. This technique has not undergone any further testing according to the literature, however and it is unknown what effect ions in the blood would have upon the electrostatic interaction between the microbubbles and the microparticles. The large size of the microbubble-microparticle aggregates would also be of concern for in vivo applications.
The use of a different type of magnetic microbubble for ultrasound mediated delivery was published by Stride et al. in 2009. Various magnetic bubble preparations, the main formulation is shown in , were synthesised and their efficacy as transfection reagents under the influence of a magnetic field and/or ultrasound was tested in vitro in chinese hamster ovary cells. Each bubble formulation was co-injected with naked plasmid DNA encoding for luciferase. Exposure of the bubble formulations to either ultrasound or a magnetic field alone had very little effect but a combination of ultrasound and a magnetic field showed a significant increase in transfection as measured using bioluminescence imaging as shown in . A mixture of non-magnetic microbubbles and magnetic micelles (containing magnetic nanoparticles but no gas) in fact showed slightly higher transfection efficiency upon exposure to both ultrasound and a magnetic field. It was hypothesised that this could be due to aggregation of the micelles and microbubbles or a sensitisation of the cell surface by the magnetic particles perhaps related to the mechanisms responsible for magnetofection. These hypotheses are discussed further later Citation[104]. A similar experiment was subsequently conducted in a preliminary in vivo study in a mouse model with magnetic microbubbles and plasmid DNA again co-injected. A small magnet was positioned above right lung and both in vivo and ex vivo bioluminescence imaging demonstrated that the transfection was confined to this organ and only observed with exposure to both ultrasound and the magnetic field Citation[105].
Figure 1. Theoretical structure of a magnetic microbubble based upon the formulation by Stride et al. The gas core is surrounded by a ferrofluid and stabilized by an outer coating of L-α-phosphatidylcholine. The average size is about 2 µm.
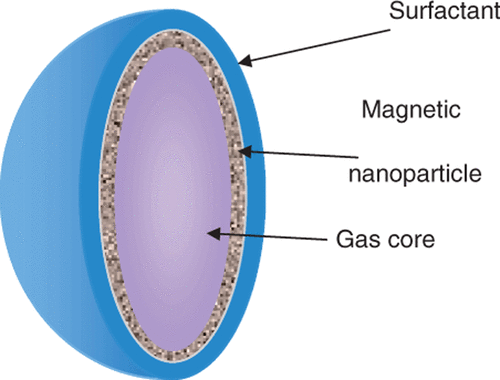
Figure 2. Bioluminescence imaging data for transfection enhancement of Chinese hamster ovary cells by magnetic microbubbles under different exposure conditions (101). A = non-magnetic microbubbles; B = magnetic micelles; C(i) = magnetic microbubbles. US = ultrasound; MF = magnetic field. Up/down refers to the surface on of the exposure chamber on which the cells were located and hence whether cell/bubble contact would be promoted by buoyancy.
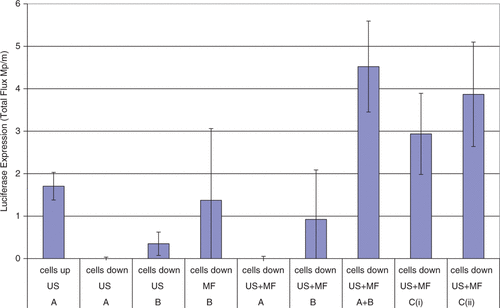
In 2010, Vlaskou et al. published a study on magnetically and acoustically active lipospheres Citation[42], Citation[106]. Lipospheres were synthesised based on a technique by Unger et al. who produced liposheres with soy bean oil incorporating paclitaxel Citation[69]. The lipospheres are synthesised by shaking a mixture of soybean oil, a cationic lipid, magnetic nanoparticles, DNA, aqueous buffer and a perfluoropropane gas in a sealed vial, optical and fluorescence images can be seen in . The lipospheres showed lower contrast to tissue ratios than for ultrasound contrast agents that have been approved for clinical use unlike those developed by Stride et al.
Figure 3. (A) Phase contrast and (B) fluorescence (measured at a wavelength of 490/509 nm) microscopy images of the Magnetic and acoustically active liposomes composed of fluidMAG-Tween-60 magnetic nanoparticles and the luciferase plasmid pBLuc, which was fluorescently labelled with the intercalated dye YOYO-1. Reproduced with kind permission from John Wiley & Sons from of reference Citation[106] © 2010 WILEY-VDH Verlag GmbH & Co. KGaA, Weinheim.
![Figure 3. (A) Phase contrast and (B) fluorescence (measured at a wavelength of 490/509 nm) microscopy images of the Magnetic and acoustically active liposomes composed of fluidMAG-Tween-60 magnetic nanoparticles and the luciferase plasmid pBLuc, which was fluorescently labelled with the intercalated dye YOYO-1. Reproduced with kind permission from John Wiley & Sons from Figure 2 of reference Citation[106] © 2010 WILEY-VDH Verlag GmbH & Co. KGaA, Weinheim.](/cms/asset/56a3cc63-5800-4d1f-a279-6561c4cae42f/ihyt_a_668639_f0003_b.gif)
As therapeutic delivery agents the lipospheres did achieve nucleic acid delivery comparable to commercially available transfection reagents when a magnetic field was applied. When ultrasound and a magnetic field were applied, however, there was no improvement in transfection efficiency in vitro. The reason was thought to be due to the soybean oil reducing the acoustic responsiveness of the microbubbles. Using a skin fold chamber on a mouse, injections of lipospheres with ultrasound and a magnetic field did achieve a large deposition of fluorescent material, but no direct observation or quantitative data were obtained for the transfection Citation[106].
The lipospheres were then substituted by Vlaskou et al. for lipid shelled microbubbles conjugated with positively charged magnetic nanoparticles through electrostatic interactions. These microbubbles had a much greater response to ultrasound and the ultrasound contrast to tissue ratio of the magnetic microbubbles was found to be higher than Sonovue and a combination of ultrasound and magnetic exposure improved transfection efficiency in vitro Citation[107].
New magnetic micelle/microbubbles formulation
To understand why the physical mixture of magnetic micelles and non-magnetic microbubbles produced the highest transfection efficiency in the previous in vitro study, a series of experiments was devised. First, the microbubble/micelle mixture was injected into a 200 µm capillary tube at a flow rate of ∼1 mm/s and observed both optically and acoustically under pulse echo imaging. The majority of the particles were seen to move towards a 1.5 T FeNdB permanent magnet positioned below the capillary. This implied there had been some association between the micelles and the bubbles to produce magnetic microbubbles. To study the interaction further, magnetic micelles incorporating the fluorophore boron-dipyrromethene (BoDIPY) C10 were synthesised and mixed with non-fluorescent non-magnetic microbubbles. This was performed by half filling a syringe (1 ml) with magnetic micelles, then filling the rest with microbubbles. As the microbubbles float, they pass through the micelle mixture. The syringe was then inverted three times and the mixture used directly. The microbubbles were then observed to fluoresce as if surrounded by the micelles as shown in . The microbubbles still fluoresced after being centrifuged, indicating a strong conjugation or aggregation and apparently producing a higher yield of magnetically responsive microbubbles than the direct fabrication method. It is this effective increase in concentration that is thought to have been responsible for the improved transfection performance in the previous experiments.
Figure 4. Magnetic microbubbles prepared by mixing non-magnetic microbubbles with fluorescently labelled magnetic micelles. (A) retention against flow in a capillary tube following application of a magnetic field (B) fluorescent microbubble obtained by mixing non-fluorescent microbubbles with fluorescent magnetic micelles.
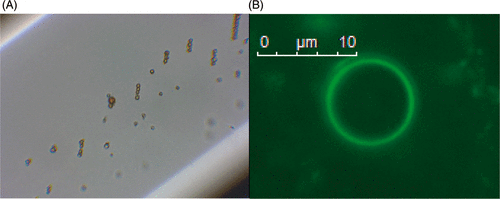
Conclusions and outlook
Magnetic nanoparticles have great potential for drug delivery showing tolerance and efficacy in human trials. Magnetofection also has been shown to be a safe efficient method of gene therapy. As the nanoparticles can be controlled with an external magnetic field they present excellent targeting capabilities. However retention against blood flow at a target site within the body is a significant challenge on account of their small size.
Microbubbles, already used in ultrasound contrast enhancement, have been shown to be effective drug delivery vehicles. Microbubbles under ultrasound excitation can promote sonoporation reversibly increasing the permeability of nearby cells and tissue membranes and thereby, increasing drug uptake. Microbubbles have also been formulated to carry therapeutic agents attached to the shell or in an inner solvent layer. However, efficient targeting of microbubbles in vivo is still limited.
The advantages of magnetic and ultrasound drug delivery have been combined in a number of ways. In particular, combining magnetic nanoparticles and microbubbles to form magnetic microbubbles has been shown to produce a controllable, versatile delivery vehicle that can be visualised by MRI and ultrasound. They have been successfully used for localised transfection in vitro and promising results from in vivo studies have recently been obtained.
In terms of application, magnetic microbubbles could, in principle, be used across the range of applications for which both non-magnetic microbubbles and magnetic microparticles have been proposed as described above. The most immediate application would perhaps be for targeted delivery where magnetic localisation could be used to increase the dwell time of microbubbles in a target volume, whilst specificity could be provided by biochemical targeting. Passive extravasation and/or tissue penetration would be limited for bubbles in the micrometre size range. As has been discussed earlier, however, exposure to both ultrasound and magnetic fields can temporarily increase tissue permeability and uptake. It would be possible, moreover, both to load microbubbles with smaller therapeutic particles which could be released following microbubble disruption and to produce magnetically and acoustically responsive particles that were large compared with conventional nanoparticles but smaller than 1 µm. Perfluorocarbon droplets, which are initially a few hundred nanometres in diameter but which expand following ultrasound exposure have for example have been utilised for contrast enhancement Citation[55].
The maximum depth at which magnetic localisation can be achieved remains an open question and depends on numerous factors including the nature of the particles being confined, the generation of the magnetic field and potential safety considerations and the physiological environment e.g. shear stresses experienced within a vessel. As described above, successful localisation of magnetic microparticles has been demonstrated in vivo including at tissue depths greater than 10 cm Citation[108] and work is currently underway by the authors to quantify the conditions under which localisation of magnetic microbubbles can be achieved.
Acknowledgements
The authors would like to thank the University of Oxford, University College London and the ESPRC for supporting this research and The Royal Institution of Great Britain for use of the live cell imager. They would also like to thank Dr Daniel Ortega and Cristina Blanco-Andujar for technical support.
Declaration of interest: The authors report no conflicts of interest. The authors alone are responsible for the content and writing of the paper.
References
- Langer R. Drug delivery and targeting. Nature 1998; 392(6679 Suppl.)5–10
- Harrison C. The patent cliff steepens. Nature Reviews Drug Discovery 2011; 10(1)12–3
- Arruebo M. Drug delivery from structured porous inorganic materials. Wiley Interdisciplinary Reviews: Nanomedicine and Nanobiotechnology 2011; 4(1)16–30
- Pankhurst QA, Connolly J, Jones SK, Dobson J. Applications of magnetic nanoparticles in biomedicine. Journal of Physics D: Applied Physics 2003; 36(13)R167–R81
- Jackson JK, Pirmoradi FN, Wan CPL, Siu T, Chiao M, Burt HM. Increased accumulation of paclitaxel and doxorubicin in proliferating capillary cells and prostate cancer cells following ultrasound exposure. Ultrasonics 2011; 51(8)932–9
- Schlicher RK, Radhakrishna H, Tolentino TP, Apkarian RP, Zarnitsyn V, Prausnitz MR. Mechanism of intracellular delivery by acoustic cavitation. Ultrasound Med Biol 2006; 32(6)915–24
- Arruebo M, Fernández-Pacheco R, Ibarra MR, Santamaría J. Magnetic nanoparticles for drug delivery. Nano Today 2007; 2(3)22–32
- Lubbe AS, Alexiou C, Bergemann C. Clinical applications of magnetic drug targeting. Journal of Surgical Research Feb, 2001; 95(2)200–6
- Lubbe AS, Bergemann C, Riess H, Schriever F, Reichardt P, Possinger K, et al. Clinical experiences with magnetic drag targeting: A phase I study with 4′-epidoxorubicin in 14 patients with advanced solid tumors. Cancer Research 1996; 56(20)4686–93
- Forbes SJ, Hodgson HJF. Review article: Gene therapy in gastroenterology and hepatology. Alimentary Pharmacology and Therapeutics 1997; 11(5)823–36
- Verma IM, Somia N. Gene therapy – Promises, problems and prospects. Nature 1997; 389(6648)239–42
- Sun C, Lee JSH, Zhang M. Magnetic nanoparticles in MR imaging and drug delivery. Advanced Drug Delivery Reviews 2008; 60(11)1252–65
- McBain SC, Yiu HHP, Dobson J. Magnetic nanoparticles for gene and drug delivery. International Journal of Nanomedicine 2008; 3(2)169–80
- Plank C, Zelphati O, Mykhaylyk O. Magnetically enhanced nucleic acid delivery. Ten years of magnetofection-Progress and prospects. Advanced Drug Delivery Reviews 2011; 63(14-15)1300–31
- Deckers R, Rome C, Moonen CTW. The role of ultrasound and magnetic resonance in local drug delivery. Journal of Magnetic Resonance Imaging 2008; 27(2)400–9
- Ferrara K, Pollard R, Borden M. Ultrasound microbubble contrast agents: Fundamentals and application to gene and drug delivery. Annual Reviews in Biomedical Engineering 2007; 9: 415–47
- Xenariou S, Griesenbach U, Liang HD, Zhu J, Farley R, Somerton L, et al. Use of ultrasound to enhance nonviral lung gene transfer in vivo. Gene Therapy 2007; 14(9)768–74
- Tachibana K, Uchida T, Ogawa K, Yamashita N, Tamura K. Induction of cell-membrane porosity by ultrasound. Lancet 1999; 353(9162)1409
- Freeman MW, Arrott A, Watson JHL. Magnetism in medicine. Journal of Applied Physics 1960; 31(5)S404–S5
- Polyak B, Friedman G. Magnetic targeting for site-specific drug delivery: Applications and clinical potential. Expert Opinion on Drug Delivery 2009; 6(1)53–70
- Andrä W, Nowak H. Magnetism in Medicine: A Handbook2nd. Wiley-VCH, Weinheim 2007
- Durán JDG, Arias JL, Gallardo V, Delgado AV. Magnetic colloids as drug vehicles. Journal of Pharmaceutical Sciences 2008; 97(8)2948–83
- Barry SE. Challenges in the development of magnetic particles for therapeutic applications. International Journal of Hyperthermia 2008; 24(6)451–66
- Thiesen B, Jordan A. Clinical applications of magnetic nanoparticles for hyperthermia. International Journal of Hyperthermia 2008; 24(6)467–74
- Kami D, Takeda S, Itakura Y, Gojo S, Watanabe M, Toyoda M. Application of magnetic nanoparticles to gene delivery. International Journal of Molecular Sciences 2011; 12(6)3705–22
- Willard MA, Kurihara LK, Carpenter EE, Calvin S, Harris VG. Chemically prepared magnetic nanoparticles. International Materials Reviews 2004; 49(3-4)125–70
- Hans ML, Lowman AM. Biodegradable nanoparticles for drug delivery and targeting. Current Opinion in Solid State and Materials Science 2002; 6(4)319–27
- Neuberger T, Schöpf B, Hofmann H, Hofmann M, Von Rechenberg B. Superparamagnetic nanoparticles for biomedical applications: Possibilities and limitations of a new drug delivery system. Journal of Magnetism and Magnetic Materials 2005; 293(1)483–96
- Meyers PH, Cronic F, Nice CM, Jr. Experimental approach in the use and magnetic control of metallic iron. The American Journal of Roentgenology, Radium Therapy, and Nuclear 1963; 90: 1068–77
- Medeiros SF, Santos AM, Fessi H, Elaissari A. Stimuli-responsive magnetic particles for biomedical applications. International Journal of Pharmaceutics 2011; 403(1–2)139–61
- Zimmerman U, Scheurich P, Pilwat G, Benz R. Cells with manipulated functions: New perspectives for cell biology, medicine, and technology. Angewandte Chemie – International Edition in English 1981; 20(4)325–44
- Senyei A, Widder K, Czerlinski G. Magnetic guidance of drug-carrying microspheres. Journal of Applied Physics 1978; 49(6)3578–83
- Senyei AE, Reich SD, Gonczy C, Widder KJ. In vivo kinetics of magnetically targeted low-dose doxorubicin. Journal of Pharmaceutical Sciences 1981; 70(4)389–91
- Widder KJ, Morris RM, Poore GA, Howard DP, Senyei AE. Selective targeting of magnetic albumin microspheres containing low-dose doxorubicin – total remission in Yoida Srcoma-bearing rats. European Journal of Cancer & Clinical Oncology. [Article] 1983; 19(1)135–9
- Edelman ER, Brown L, Taylor J, Langer R. In vitro and in vivo kinetics of regulated drug release from polymer matrices by oscillating magnetic fields. Journal of Biomedical Materials Research 1987; 21(3)339–53
- Hafeli UO, Sweeney SM, Beresford BA, Sim EH, Macklis RM. Magnetically directed poly(lactic acid) 90Y-microspheres: Novel agents for targeted intracavitary radiotherapy. Journal of Biomedical Materials Research 1994; 28(8)901–8
- Hafeli UO, Sweeney SM, Beresford BA, Humm JL, Macklis RM. Effective targeting of magnetic radioactive 90Y-microspheres to tumor cells by an externally applied magnetic field. Preliminary in vitro and in vivo results. Nuclear Medicine and Biology 1995; 22(2)147–55
- Lübbe AS, Bergemann C, Huhnt W, Fricke T, Riess H, Brock JW, et al. Preclinical experiences with magnetic drug targeting: Tolerance and efficacy. Cancer Research 1996; 56(20)4694–701
- Alexiou C, Arnold W, Klein RJ, Parak FG, Hulin P, Bergemann C, et al. Locoregional cancer treatment with magnetic drug targeting. Cancer Research 2000; 60(23)6641–8
- Koda J, Venook A, Walser E, Goodwin S. A multicenter, phase I/II trial of hepatic intra-arterial delivery of doxorubicin hydrochloride adsorbed to Magnetic Targeted Carriers in patients with hepatocellular carcinoma. Eur J Cancer. [Meeting Abstract] 2002; 38: S18
- Dykxhoorn DM, Lieberman J. Running interference: Prospects and obstacles to using small interfering RNAs as small molecule drugs. Annual Review of Biomedical Engineering 2006; 8: 377–402
- Mykhaylyk O, Vlaskou D, Tresilwised N, Pithayanukul P, Möller W, Plank C. Magnetic nanoparticle formulations for DNA and siRNA delivery. Journal of Magnetism and Magnetic Materials 2007; 311(1 SPEC. ISS.)275–81
- Mah C, Fraites TJ, Jr, Zolotukhin I, Song S, Flotte TR, Dobson J, et al. Improved method of recombinant AAV2 delivery for systemic targeted gene therapy. Molecular Therapy 2002; 6(1)106–12
- Hughes C, Galea-Lauri J, Farzaneh F, Darling D. Streptavidin paramagnetic particles provide a choice of three affinity-based capture and magnetic concentration strategies for retroviral vectors. Molecular Therapy 2001; 3(4)623–30
- Scherer F, Anton M, Schillinger U, Henke J, Bergemann C, Krüger A, et al. Magnetofection: Enhancing and targeting gene delivery by magnetic force in vitro and in vivo. Gene Therapy 2002; 9(2)102–9
- Abdallah B, Hassan A, Benoist C, Goula D, Behr JP, Demeneix BA. A powerful nonviral vector for in vivo gene transfer into the adult mammalian brain: Polyethylenimine. Human Gene Therapy 1996; 7(16)1947–54
- Plank C, Schillinger U, Scherer F, Bergemann C, Rémy JS, Krötz F, et al. The magnetofection method: Using magnetic force to enhance gene delivery. Biological Chemistry 2003; 384(5)737–47
- Cai D, Mataraza JM, Qin ZH, Huang Z, Huang J, Chiles TC, et al. Highly efficient molecular delivery into mammalian cells using carbon nanotube spearing. Nature Methods 2005; 2(6)449–54
- Bonetta L. The inside scoop – Evaluating gene delivery methods. Nature Methods 2005; 2(11)875–82
- Kamau SW, Hassa PO, Steitz B, Petri-Fink A, Hofmann H, Hofmann-Amtenbrink M, et al. Enhancement of the efficiency of non-viral gene delivery by application of pulsed magnetic field. Nucleic Acids Research 2006; 34(5)e.40
- Ng KY, Liu Y. Therapeutic ultrasound: Its application in drug delivery. Medicinal Research Reviews 2002; 22(2)204–23
- Newman CM, Lawrie A, Brisken AF, Cumberland DC. Ultrasound gene therapy: On the road from concept to reality. Echocardiography 2001; 18(4)339–47
- Yuh EL, Shulman SG, Mehta SA, Xie J, Chen L, Frenkel V, et al. Delivery of systemic chemotherapeutic agent to tumors by using focused ultrasound: Study in a murine model. Radiology 2005; 234(2)431–7
- Azhari H. Basics of Biomedical ultrasound for engineers1st. John Wiley and sons, Inc., New Jersey 2010
- Ferrara KW. Driving delivery vehicles with ultrasound. Advanced Drug Delivery Reviews 2008; 60(10)1097–102
- Bailey MR, Khokhlova VA, Sapozhnikov OA, Kargl SG, Crum LA. Physical mechanisms of the therapeutic effect of ultrasound (a review). Acoustical Physics 2003; 49(4)369–88
- Apfel RE. Sonic effervescence: A tutorial on acoustic cavitation. Journal of the Acoustical Society of America 1997; 101(3)1227–37
- Iwanaga K, Tominaga K, Yamamoto K, Habu M, Maeda H, Akifusa S, et al. Local delivery system of cytotoxic agents to tumors by focused sonoporation. Cancer Gene Therapy 2007; 14(4)354–63
- Meairs S, Alonso A. Ultrasound, microbubbles and the blood-brain barrier. Progress in Biophysics and Molecular Biology 2007; 93(1-3)354–62
- Nyborg WL. Ultrasonic microstreaming and related phenomena. British Journal of Cancer 1982; 45(Suppl. 5)156–60
- Marmottant P, Hilgenfeldt S. Controlled vesicle deformation and lysis by single oscillating bubbles. Nature 2003; 423(6936)153–6
- O'Neill BE, Li KCP. Augmentation of targeted delivery with pulsed high intensity focused ultrasound. International Journal of Hyperthermia 2008; 24(6)506–20
- Meltzer RS, Tickner EG, Sahines TP, Popp RL. The source of ultrasound contrast effect. Journal of Clinical Ultrasound 1980; 8(2)121–7
- Harvey CJ, Pilcher JM, Eckersley RJ, Blomley MJK, Cosgrove DO. Advances in ultrasound. Clinical Radiology 2002; 57(3)157–77
- Nanda NC. History of echocardiographic contrast agents. Clinical Cardiology 1997; 20(10 SUPPL.)I7–I11
- Klibanov AL. Ligand-carrying gas-filled microbubbles: Ultrasound contrast agents for targeted molecular imaging. Bioconjugate Chem. [Article] 2005; 16(1)9–17
- Quaia E. Contrast Media in Ultrasonography: Basic principles and clinical applications. Berlin: Springer-Verlag. 2005
- Unger EC, Hersh E, Vannan M, McCreery T. Gene delivery using ultrasound contrast agents. Echocardiography 2001; 18(4)355–61
- Unger EC, McCreery TP, Sweitzer RH, Caldwell VE, Wu YQ. Acoustically active lipospheres containing paclitaxel – A new therapeutic ultrasound contrast agent. Investigative Radiology 1998; 33(12)886–92
- Tachibana K, Tachibana S. Albumin microbubble echo-contrast material as an enhancer for ultrasound accelerated thrombolysis. Circulation 1995; 92(5)1148–50
- Lindner JR, Kaul S. Delivery of drugs with ultrasound. Echocardiography 2001; 18(4)329–37
- Wu Y, Unger EC, McCreery TP, Sweitzer RH, Shen D, Wu G, et al. Binding and lysing of blood clots using MRX-408. Investigative Radiology 1998; 33(12)880–5
- Prentice P, Cuschierp A, Dholakia K, Prausnitz M, Campbell P. Membrane disruption by optically controlled microbubble cavitation. Nature Physics 2005; 1(2)107–10
- Guzmán HR, Nguyen DX, Khan S, Prausnitz MR. Ultrasound-mediated disruption of cell membranes. I. Quantification of molecular uptake and cell viability. Journal of the Acoustical Society of America 2001; 110(1)588–96
- Guzmán HR, Nguyen DX, Khan S, Prausnitz MR. Ultrasound-mediated disruption of cell membranes. II. Heterogeneous effects on cells. Journal of the Acoustical Society of America 2001; 110(1)597–606
- Price RJ, Skyba DM, Kaul S, Skalak TC. Delivery of colloidal particles and red blood cells to tissue through microvessel ruptures created by targeted microbubble destruction with ultrasound. Circulation 1998; 98(13)1264–7
- Lentacker I, Geers B, Demeester J, De Smedt SC, Sanders NN. Design and evaluation of doxorubicin-containing microbubbles for ultrasound-triggered doxorubicin delivery: Cytotoxicity and mechanisms involved. Molecular Therapy 2010; 18(1)101–8
- Lindner JR, Coggins MP, Kaul S, Klibanov AL, Brandenburger GH, Ley K. Microbubble persistence in the microcirculation during ischemia/reperfusion and inflammation is caused by integrin- and complement- mediated adherence to activated leukocytes. Circulation 2000; 101(6)668–75
- Villanueva FS, Jankowski RJ, Klibanov S, Pina ML, Alber SM, Watkins SC, et al. Microbubbles targeted to intercellular adhesion molecule-1 bind to activated coronary artery endothelial cells. Circulation 1998; 98(1)1–5
- Lum AFH, Borden MA, Dayton PA, Kruse DE, Simon SI, Ferrara KW. Ultrasound radiation force enables targeted deposition of model drug carriers loaded on microbubbles. Journal of Controlled Release 2006; 111(1–2)128–34
- Kim HJ, Greenleaf JF, Kinnick RR, Bronk JT, Bolander ME. Ultrasound-mediated transfection of mammalian cells. Human Gene Therapy 1996; 7(11)1339–46
- Greenleaf WJ, Bolander ME, Sarkar G, Goldring MB, Greenleaf JF. Artificial cavitation nuclei significantly enhance acoustically induced cell transfection. Ultrasound in Medicine and Biology 1998; 24(4)587–95
- Lawrie A, Brisken AF, Francis SE, Cumberland DC, Crossman DC, Newman CM. Microbubble-enhanced ultrasound for vascular gene delivery. Gene Therapy 2000; 7(23)2023–7
- Li T, Tachibana K, Kuroki M. Gene Transfer with Echo-enhanced Contrast Agents: Comparison between Albunex, Optison, and Levovist in Mice – Initial Results. Radiology 2003; 229(2)423–8
- Rahim A, Taylor SL, Bush NL, ter Haar GR, Bamber JC, Porter CD. Physical parameters affecting ultrasound/microbubble-mediated gene delivery efficiency in vitro. Ultrasound in Medicine and Biology 2006; 32(8)1269–79
- Unger EC, Hersh E, Vannan M, Matsunaga TO, McCreery T. Local drug and gene delivery through microbubbles. Progress in Cardiovascular Diseases 2001; 44(1)45–54
- Shohet RV, Chen S, Zhou YT, Wang Z, Meidell RS, Unger RH, et al. Echocardiographic destruction of albumin microbubbles directs gene delivery to the myocardium. Circulation 2000; 101(22)2554–6
- Tsunoda S, Mazda O, Oda Y, Iida Y, Akabame S, Kishida T, et al. Sonoporation using microbubble BR14 promotes pDNA/siRNA transduction to murine heart. Biochemical and Biophysical Research Communications 2005; 336(1)118–27
- Kodama T, Aoi A, Watanabe Y, Horie S, Kodama M, Li L, et al. Evaluation of transfection efficiency in skeletal muscle using nano/microbubbles and ultrasound. Ultrasound Med Biol 2010; 36(7)1196–205
- Geers B, Lentacker I, Sanders NN, Demeester J, Meairs S, De Smedt SC. Self-assembled liposome-loaded microbubbles: The missing link for safe and efficient ultrasound triggered drug-delivery. Journal of Controlled Release 2011; 152(2)249–56
- Cochran MC, Eisenbrey J, Ouma RO, Soulen M, Wheatley MA. Doxorubicin and paclitaxel loaded microbubbles for ultrasound triggered drug delivery. International Journal of Pharmaceutics 2011; 414(1–2)161–70
- Thanh NTK, Magnetic Nanoparticles: From Fabrication to Clinical Applications. CRC Press, Taylor and Francis Group, Boca Raton 2011
- Hynynen K. MRIgHIFU: A tool for image-guided therapeutics. Journal of Magnetic Resonance Imaging 2011; 34(3)482–93
- D'Arrigo JS, Method for the production of medical-grade lipid-coated microbubbles, paramagnetic labeling of such microbubbles and therapeutic uses of microbubbles. US Patent US5215680. 1993
- Smith MJ, Ho VHB, Darton NJ, Slater NKH. Effect of Magnetite Nanoparticle Agglomerates on Ultrasound Induced Inertial Cavitation. Ultrasound Med Biol 2009; 35(6)1010–4
- Saravanan M, Bhaskar K, Maharajan G, Pillai KS. Ultrasonically controlled release and targeted delivery of diclofenac sodium via gelatin magnetic microspheres. International Journal of Pharmaceutics 2004; 283(1–2)71–82
- Kaminski MD, Xie Y, Mertz CJ, Finck MR, Chen H, Rosengart AJ. Encapsulation and release of plasminogen activator from biodegradable magnetic microcarriers. European Journal of Pharmaceutical Sciences 2008; 35(1–2)96–103
- Wong KK, Huang I, Kim YR, Tang H, Yang ES, Kwong KK, et al. In vivo study of microbubbles as an MR susceptibility contrast agent. MagnResonMed 2004; 52(3)445–52
- Yang F, Li L, Li Y, Chen Z, Wu J, Gu N. Superparamagnetic nanoparticle-inclusion microbubbles for ultrasound contrast agents. Physics in Medicine and Biology 2008; 53(21)6129–41
- Yang F, Li Y, Chen Z, Zhang Y, Wu J, Gu N. Superparamagnetic iron oxide nanoparticle-embedded encapsulated microbubbles as dual contrast agents of magnetic resonance and ultrasound imaging. Biomaterials 2009; 30(23–24)3882–90
- Park JI, Jagadeesan D, Williams R, Oakden W, Chung S, Stanisz GJ, et al. Microbubbles loaded with nanoparticles: A route to multiple imaging modalities. ACS Nano 2010; 4(11)6579–86
- Soetanto K, Watarai H. Development of magnetic microbubbles for drug delivery system (DDS). Japanese Journal of Applied Physics, Part 1: Regular Papers and Short Notes and Review Papers 2000; 39(5 B)3230–2
- Soetanto K, Watarai H, Ferromagnetic Ultrasound Microbubbles Contrast Agent. Proceedings of the 25th Annual International Conference of the IEEE Engineering in Medicine and Biology Society. 2003;2:1226–29
- Stride E, Porter C, Prieto AG, Pankhurst Q. Enhancement of Microbubble Mediated Gene Delivery by Simultaneous Exposure to Ultrasonic and Magnetic Fields. Ultrasound in Medicine & Biology 2009; 35(5)861–8
- Mulvana H, Eckersley RJ, Browning R, Hajnal JV, Stride E, Barrack T, Tang, M. Pankhurst, Q. Wells, D. editors. Enhanced gene transfection in vivo using magnetic localisation ofultrasound contrast agents: Preliminary results. Proceedings of the IEEE Ultrasonics Symposium 2010; 670-73
- Vlaskou D, Mykhaylyk O, Krötz F, Hellwig N, Renner R, Schillinger U, Gleich B, Heidsieck A, Schmitz G, Hensel K, Plank C. Magnetic and Acoustically Active Lipospheres for Magnetically Targeted Nucleic Acid Delivery. Advanced Functional Materials 2010; 20(22)3881–94
- Vlaskou D, Pradhan P, Bergemann C, Klibanov AL, Hensel K, Schmitz G, Plank C, Mykhaylyk O, editors. Magnetic microbubbles: Magnetically targeted and ultrasound-triggered vectors for gene delivery in vitro. AIP Conference Proceedings 8th International conference on the scientific and clinical applications of magnetic carriers. 2010; 1311:485–94
- Allen LM, Kent T, Wolfe C, Ficco C, Johnson J. A magnetically targetable drug carrier for paclitaxel. Scientific and Clinical Applications of Magnetic Carriers, Häfeli Urs, Schütt Wolfgang, Teller Joachim, Zborowski Maciej. Plenum Press, New York 1997; 481–494