Abstract
The aim of this study was to examine whether amantadine (AMA), as a low-affinity noncompetitive N-methyl-d-aspartate (NMDA) receptor antagonist, is able to improve cognitive deficits caused by chronic stress in rats. Male Wistar rats were divided into four groups: control, control + AMA, stress and stress + AMA groups. The chronic stress model combined chronic unpredictable stress (CUS) with isolated feeding. Animals were exposed to CUS continued for 21 days. AMA (25 mg/kg) was administrated p.o. for 20 days from the 4th day of CUS to the 23rd. Weight and sucrose consumption were measured during model establishing period. Spatial memory was evaluated using the Morris water maze (MWM) test. Following MWM testing, both long-term potentiation (LTP) and depotentiation were recorded in the hippocampal CA1 region. NR2B and postsynaptic density protein 95 (PSD-95) proteins were measured by Western-blot analysis. AMA increased weight and sucrose consumption of stressed rats. Spatial memory and reversal learning in stressed rats were impaired relative to controls, whereas AMA significantly attenuated cognitive impairment. AMA also mitigated the chronic stress-induced impairment of hippocampal synaptic plasticity, in which both the LTP and depotentiation were significantly inhibited in stressed rats. Moreover, AMA enhanced the expression of hippocampal NR2B and PSD-95 in stressed rats. The data suggest that AMA may be an effective therapeutic agent for depression-like symptoms and associated cognitive disturbances.
Introduction
Stressful events predispose individuals to a variety of mental disorders, including depression, which is one of the most serious and recurring psychiatric disorders with tremendous personal and socioeconomic consequences. Exposure to aversive experiences induces profound behavioral changes in humans and rodents, including depressive-like symptoms, anxiety and cognitive deficits, which are paralleled by physiological abnormities (Mizoguchi et al., Citation2000; Quan et al., Citation2011a). Based on these observations, the chronic unpredictable stress (CUS) animal model has been developed to mimic the development and progress of clinical depression (Willner, Citation1997). Moreover, there is considerable evidence that cognition deficits are an essential component of major depression, and antidepressant treatment may mitigate cognitive impairments in parallel to mood improvement of depressive patients (Airaksinen et al., Citation2004).
Rodents that are exposed to chronic unpredictable stressors may elicit depression-like symptoms (Willner, Citation2005). When the intensity and duration of stressors on brain structures exceed a certain individual-specific threshold, they can impair hippocampal-dependent spatial memory (Kim & Diamond, Citation2002; McEwen & Magarinos, Citation2001). There is strong evidence that stress-induced effects on cognition are at least in part mediated by the stress-evoked excessive release of glutamate (Sapolsky, Citation2000; Szakacs et al., Citation2012). Meanwhile, synaptic plasticity, including long-term potentiation (LTP) and long-term depression, is impaired by CUS (Alfarez et al., Citation2003; Marsden, Citation2013). Existing studies suggest that synaptic weakening, manifest as depotentiation (defined activity-dependent reversal of previously established LTP) in the hippocampus, is required for cognitive flexibility (An et al., Citation2013a) and may be impacted by stress as well.
Stress-associated cognitive deficits are observed with alterations of protein expression. The NR2B receptor plays an important role in the regulation of LTP (Fox et al., Citation2006). As a major postsynaptic scaffolding protein and principal mediators of the N-methyl-d-aspartate (NMDA) receptor, postsynaptic density protein 95 (PSD-95) is also essential for synaptic plasticity in the hippocampus (Ehrlich et al., Citation2007). It is well known that a reduction of these proteins expression in the synapse can impair plasticity and cognition.
Amantadine (AMA) is a low-affinity noncompetitive NMDA receptor antagonist that is widely used in treatment of Parkinson’s disease. Previous studies indicate that AMA has an antidepressant activity (Huber et al., Citation1999; Moryl et al., Citation1993). Moreover, AMA can enhance the therapeutic effect of antidepressants when included as an adjunct therapy in treatment-resistant depression (Rogoz et al., Citation2007; Stryjer et al., Citation2003). One of our previous investigations reports that memantine and its derivatives exhibit an antidepressant effect and reverse the behavioral and synaptic deficits in the CUS rats, but with side-effects (Quan et al., Citation2011b). Hence, we focused on exploring the potential therapeutic effects of AMA in impeding the cognition deficits and aberrant synaptic plasticity following chronic stress in the rodent. A hypothesis was raised that AMA was able to effectively impede the development of depression-like behavior and the spatial cognitive deficits, and to some extent restore impaired synaptic plasticity in stressed rats. Accordingly, a rat model of chronic stress was established. Both body weight and sucrose consumption were measured. Afterwards, spatial learning and memory were tested with Morris water maze (MWM). The LTP and depotentiation from hippocampal Schaffer collaterals to CA1 region were recorded to examine the bidirectional synaptic plasticity. Finally, the protein expression of NR2B and PSD-95 in the hippocampus was assayed by Western to explore the possible mechanism.
Materials and methods
Animal and drug treatments
Adult male Wistar rats weighing 220–230 g were purchased from the Laboratory Animal Center, Academy of Military Medical Science of People’s Liberation Army. Before all experiments, animals were allowed 3 days of habituation and kept in groups of four to five under standard laboratory conditions (24 ± 2 °C room temperature, 12 h light/dark cycle with lights on at 7:00 a.m., and freedom to food and drink) in the Medical School of Nankai University. All procedures adhere to the National Institute of Health Guide for the Care and Use of Laboratory Animals, and are approved by the Committee for Animal Care at Nankai University. Every effort has been made to minimize animal suffering and the number of animals.
After the habituation, they were arbitrarily divided into four groups: control group (Con), control with AMA group (Con + AMA), stress group (Str) and stress with AMA group (Str + AMA). The animal model of stress was established by combining CUS and isolated feeding. The animals of both Str and Str + AMA groups were reared in one cage separately with each individual, and the rats of other two groups were kept in groups of four to five. AMA was bought from Jiangsu Peng Harrier Pharmaceutical Corporation, China. The rats treated with AMA in both the Con + AMA and Str + AMA groups were administered daily by the gavage for 20 days at a dose of 25 mg/kg dissolved in water from the 4th day of CUS to the 23rd. At the same time, both Con and CUS animals received vehicles by gavage at the same dose.
Chronic unpredictable stress procedure
The stress procedure was performed as described (Willner, Citation1997) with substantial modification and conducted for 3 weeks. The animal model of stress consists of seven different kinds of stressors: food deprivation (24 h), water deprivation (24 h), noise (40 kHz, 90 dB, 9 min), reversed light/dark cycle (dark: 7:00 am∼19:00 pm; light: 19:00 pm∼next day 7:00 am), ice water swimming (4 °C, 5 min) in a 40-cm-diameter and 50-cm-depth glass tank, tilted cage with their own homecage (45°, 7 h) and tail pinch (1 min) with hemostatic forceps. Each rat received one stressor per day individually, which was sufficient alone to induce long-lasting effects. In order to achieve the unpredictability, the stressors were applied in a different sequence each week to avoid any habituation. One thing to note here was that both food deprivation and water deprivation stressors were not arranged in the day of sucrose intake procedure to avoid continuous hunger. On the other hand, rats in the Con and Con + AMA groups did not receive any stressors and were housed in normal conditions.
Body weight measurement and sucrose preference test
The body weight was measured every 3 days at 9:00 a.m. from the 1st to the 21st days. The sucrose preference test has been slightly modified from the previous description (Casarotto & Andreatini, Citation2007). Prior to the first sucrose preference test in all experiments, all rats in the four groups were trained to habituate sucrose water by placing a bottle of normal water and a bottle of sucrose solution (1%, w/v) with them for 2 days. Additionally, the position of the bottles was changed several times during the period. Afterwards, they were deprived of food and water for 23 h from 10:00 a.m. until 9:00 a.m. on the next day. Each rat was provided with 1% sucrose solution and normal water individually for 1 h, and the weights of sucrose solution and water consumed were recorded accordingly. During the 3 weeks of the CUS process, the sucrose consumption test was carried out at the end of each week in the same way, but without the 2-day habituation. Based on, there were four sucrose consumption tests. Finally, the total fluid intake and the percentage of sucrose consumption were calculated as follows: total fluid intake = sucrose solution + normal water; sucrose consumption percentage =sucrose solution intake/total fluid intake × 100%.
Morris water maze test
After the last day of gavage, the MWM test was performed to monitor the spatial cognition. The MWM consists of a metal pool (150 cm in diameter and 60 cm in height) filled with tap water that dissolved black nontoxic ink (22 ± 2 °C, 45 cm deep). As described in our previous papers (An et al., Citation2013b; Quan et al., Citation2011b), the maze is divided into four quadrants with two imaginary perpendicular lines crossing in the center of the tank. The end of each line demarcates four cardinal points: North (N), South (S), East (E) and West (W). A 10-cm-diameter platform that could be moved is positioned in the middle of one quadrant, and its top is submerged 2 cm below the water surface. The rat had to learn to use distal cues to navigate a direct path to this hidden platform and escape from the water. In the maze, the rat’s movement is monitored by a CCD camera connected to a personal computer, through which data are collected and analyzed (Ethovision 2.0, Noldus, Wagenigen, Netherlands). The four start locations of S, E, NE and SW were chosen, as these were the distal start locations that were closer to being equal in length with regard to distance from the platform (Vorhees & Williams, Citation2006).
The MWM test is composed of four consecutive stages: initial training (IT), space-exploring test (SET), reversal training (RT) and reversal exploring test (RET). Firstly, the IT stage is conducted for 5 days with two sessions every day. Each session consists of four trials from the four start locations described earlier, which is carried out at 10 a.m. and 6 p.m., respectively. In each trial, the rats were given 60 s to find the hidden platform that was placed in the northwest (NW) quadrant before the start of the test. The rat was taken to the cage if it found the platform and stayed on it for ∼3 s. However, rats would be guided to the platform and stayed for at least 10 s when they failed to find the platform. The intervals among trials lasted for 8–10 min. At this stage, the escape latency and swimming speed were recorded. Secondly, the SET stage was performed using one trial without the platform after the last session of the IT stage ∼24 h later. The rats were released individually into the water from one of the starting points and allowed to swim freely for 60 s. Two parameters were recorded: platform crossings and NW quadrant dwell time (the percentage of time spent in the target quadrant). Thirdly, the RT stage was conducted for 3 days from the seventh day in the same way and with the same parameters in the IT stage. The difference was that the platform was moved into the opposite quadrant in the center of the SE quadrant. Finally, in the RET stage, the method used and the parameters recorded were the same as those in the SET stage.
In vivo LTP and depotentiation recordings
Hippocampal synaptic plasticity between Schaffer collaterals and CA1 pyramidal neurons, including LTP and depotentiation, was measured using in vivo electrophysiological techniques after the MWM test. The protocol was adopted and modified on the basis of our previous study (An et al., Citation2013a). Rats were administered with 30% urethane anesthesia (4 ml/kg, i.p., Sigma-Aldrich, St. Louis, MO). After the anesthesia, the animals were placed in stereotaxic frames (Narishige, Japan) for surgery. An incision of ∼2 cm long was made and a small hole in the skull was drilled on the left side of the brain for both the recording and stimulating electrodes. According to Paxinos and Watson coordinates (Paxinos & Watson, Citation2005), the recording electrode was implanted into the hippocampal CA1 region (3.5 mm posterior to the bregma, 2.5 mm lateral to midline, 2.0–2.2 mm ventral below the dura), and the bipolar stimulating electrode was implanted into the hippocampus Schaffer collaterals region (4.2 mm posterior to the bregma, 3.5 mm lateral to midline, 2.3–2.6 mm ventral below the dura). The optimal stimulating intensity (range 0.3–0.5 mA) that could evoke a response of 50% of its maximum amplitude was delivered at single-pulse stimulation (stimulus pulse with 0.2 ms, at 0.03 Hz) to record a 20 min baseline. After the baseline, a theta burst stimulation (TBS), consisting of 30 trains of 12 pulses (200 Hz) at 5 Hz, was applied to induce LTP. Following TBS, the single-pulse stimulation was resumed to record the evoked response every 60 s for 1 h at the baseline intensity (Scope Software, PowerLab; AD Instruments, New South Wales, Australia). Subsequently, low-frequency stimulation (LFS, 900 pulses of 1 Hz for 15 min) was delivered to induce depotentiation, and the same recording method was used and continued for 60 min. The last stabilized 15 min of LTP were normalized and used as the baseline of depotentiation. All of the initial data measurements were carried out in Clampfit 10.0 (Molecular Devices, Sunnyvale, CA).
Antibodies
Rabbit polyclonal anti-NR2B antibodies (primary antibody, working dilution 1:2000, Abcam, Cambridge, MA); Rabbit polyclonal anti-PSD-95 antibodies (primary antibody, working dilution 1:2000, Abcam, Cambridge, MA); Mouse monoclonal β-actin antibodies (primary antibody, working dilution 1:1000, Santa Cruz Biotechnology, Shang Hai); Anti-mouse IgG, AP-linked Antibody (secondary antibody, working dilution 1:5000, Cell Signaling Technology, Beverly, MA); Anti-rabbit IgG, AP-linked antibody (secondary antibody, working dilution 1:3000, Cell Signaling Technology, Beverly, MA).
Western-blotting assay
After the electrophysiological experiment, the rats were sacrificed by cervical dislocation, and then the hippocampus was dissected from rat’s brains and immediately stored at −80 °C until needed. The method of Western-blotting assay was modified on the basis of previous studies (Xu et al., Citation2012). Firstly, each hippocampus was mashed with a grinder and 200 μl lysis buffer (Beyotime Biotechnology, Haimen, China) containing a proteinase inhibitor cocktail (1:100 dilutions). The lysates were centrifuged at 12,000 r/min for 20 min at 4 °C and the supernatant was moved to a 1.5 ml tube. The protein concentration was determined using the BCA Protein Assay Kits according to the manufacturer’s instructions (Beyotime Biotechnology, Haimen, China). Secondly, the same amount of protein (50 µg) was electrophoresed by SDS–PAGE 10% gels and then proteins were electrotransferred onto 0.45-μm polyvinylidene difluoride (PVDF) membranes (Milli Pore Corporation). After that, the membrane was blocked in Tris-buffered saline (TBS) including 5% skimmed milk for 1.5 h at room temperature, and then incubated with primary antibody(Anti-NR2B and PSD-95) diluted in blocking buffer overnight at 4 °C. On the next day, the PVDF membranes were washed 3 × 10 min with Tris-buffered saline/Tween-20 (TBST) and subsequently incubated with secondary antibody. After 4 × 10 min TBST washes, the membranes were used to detect the protein band intensities by using chemiluminescent HRP substrate (Immobilon western). Finally, the quantitation analysis was performed by Photoshop 8.0.1 and compared to the loading control proteins β-actin.
Data and statistical analysis
All data were presented as mean ± SEM. A three-way repeated measures ANOVA was employed using drug and stress as factors to measure the time-course data, including body weight, sucrose consumption percentage and MWM escape latencies. Other data, obtained from the SET/RET stage, electrophysiological experiments and Western, were analyzed by two-way factorial ANOVA for multiple comparisons with stress or drug. To detect significant differences between groups, ANOVAs were supported by post hoc tests. All analyses were performed using SPSS 20 software (IBM Corp., Armonk, NY). A p value of <0.05 was taken to indicate statistical significance.
Results
Effects of AMA administration on body weight and sucrose test in stressed rats
As shown in , the body weights in all groups were increased during the 3 weeks, however, a three-way repeated measures ANOVA showed that there were statistical differences for day [F(6,21) = 279.970, p < 0.001], day × stress interaction [F(6,21) = 13.695, p < 0.001], day × drug interaction [F(6,21) = 4.129, p < 0.001] and day × stress × drug interaction [F(6,21) = 4.897, p < 0.001]. Additionally, there were significant differences of the stress effect [F(1,26) = 25.382, p < 0.001], the drug effect [F(1,26) = 4.482, p < 0.05] and the stress × drug interaction effect [F(1,26) = 7.6, p < 0.05] on the body weight. LSD post hoc analysis showed that the rat’s weight was statistically lower in the Str group compared to the Con group (p < 0.01 on the 6th day; p < 0.001 from the 9th–21st day). Moreover, the weight was significantly enhanced by AMA in the Str + AMA group compared to the Str group (p < 0.05 on the 6th day and the 9th day; p < 0.01 from the 12th–18th day; p < 0.001 on the 21st day).
Figure 1. The effects of chronic unpredictable stress and amantadine treatment on body weight (A), sucrose consumption percentage (B) and totail fliud intake (C). Data represent mean ± SEM. Error bars indicate SEM. *p < 0.05, **p < 0.01, ***p < 0.001, significant difference between the Con group and the Str group; #p < 0.05, ##p < 0.01, ###p < 0.001 significant difference between the Str group and the Str + AMA group. Con, control group (n = 6); Str, stress group (n = 9); Str + AMA, stress + amantadine treatment group (n = 9); Con + AMA, control + amantadine treatment group (n = 9).
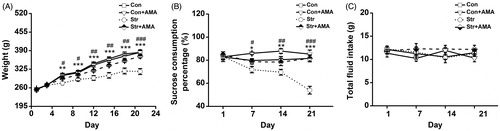
shows the effects of CUS + isolated feeding and AMA treatment on the sucrose consumption percentage. A three-way repeated measures ANOVA showed that there were statistical differences for day [F(3,36) = 4.930, p < 0.05], day × stress interaction [F(3,36) = 5.805, p < 0.01], day × drug interaction [F(3,36) = 4.421, p < 0.05] and day × stress × drug interaction [F(3,36) = 3.904, p > 0.05]. Moreover, there were significant differences of the stress effect [F(1,38) = 42.29, p < 0.001], the drug effect [F(1,38) = 39.263, p < 0.001] and the stress × drug interaction effect [F(1,38) = 7.431, p < 0.01] on the sucrose consumption percentage. LSD post hoc analysis showed that the sucrose consumption was significantly lower in the Str group than that in the Con group (p < 0.05 on the 7th day; p < 0.01 on the 14th day; p < 0.001 on the 21st day). Furthermore, it was significantly increased by AMA in the Str + AMA group compared to the Str group (p < 0.05 on the 7th day; p < 0.01 on the 12th day; p < 0.001 on the 21st day). There was no significant difference in the sucrose consumption percentage between the Con and the Con + AMA groups (p > 0.05). Moreover, it was found that there was no significant difference of total fluid intake among these 4 groups (, p > 0.05).
Effects of AMA administration on the MWM in stressed rats
and show the data obtained from the MWM test in all groups on each test day. During the IT stage, the escape latencies were visibly decreased with training (). A three-way repeated measures ANOVA showed that there were no statistical differences of day × stress interaction [F(4,37) = 0.884, p > 0.05], day × drug interaction [F(4,37) = 1.551, p > 0.05] and day × stress × drug interaction [F(4,37) = 0.814, p > 0.05], except for day [F(4,37) = 237.926, p < 0.05] as these four groups improved over the 5 days of training. However, it was found that there was a significant difference for the effect of stress × drug interaction [F(1,40) = 12.687, p < 0.01] on the escape latencies. Subsequent LSD post hoc analysis showed that there were significant differences of the escape latencies between the Str group and the Con group (; p < 0.05 on the 2nd day; p < 0.01 on the 3rd day; p < 0.001 on the 4th day; p < 0.01 on the 5th day), indicating that rats in the Str group needed more time to find the platform compared to that in the Con group. However, the escape latencies were considerably reduced in the Str + AMA group compared to that in the Str group (; p < 0.05 on the 2nd day; p < 0.01 on the 3rd day; p < 0.001 on the 4th day; p < 0.01 on the 5th day). Additionally, there was no significant difference of swimming speed among these 4 groups (, p > 0.05).
Figure 2. The performance of rats in the initial training (IT) stage and the space-exploring test (SET) stage of MWM experiment. (A) Mean escape latency was calculated for each day in the initial training. (B) Mean swimming speed in the IT stage. (C) Mean number of platform area crossings in the SET stage. (D) Mean percentage of time spent in target (NW) quadrant in the SET stage. Data represent mean ± SEM. Error bars indicate SEM. *p < 0.05, **p < 0.01, ***p < 0.001 comparison between the Con group and the Str group; #p < 0.05, ##p < 0.01, ###p < 0.001 comparison between the Str group and the Str + AMA group; the Con and stress groups, n = 12; the Str + AMA, n = 14; the Con + AMA, n = 6.
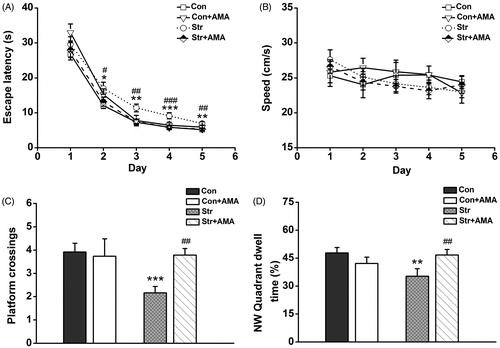
Figure 3. The performance of animals in the reversal training (RT) stage and the reversal exploring test (RET) stage of MWM experiment. (A) Mean escape latency was calculated for each day in reversal training. (B) Mean swimming speed in the RT stage. (C) Mean number of platform crossings in the RET stage. (D) Mean percentage of time spent in new target (SE) quadrant in the SET stage. Data represent mean ± SEM. Error bars indicate SEM. *p < 0.05, **p < 0.01, ***p < 0.001 comparison between the Con group and the Str group; #p < 0.05, ##p < 0.01, ###p < 0.001 comparison between the Str group and the Str + AMA group; the Con and Str groups, n = 12; the Str + AMA group, n = 14; the Con + AMA group, n = 6.
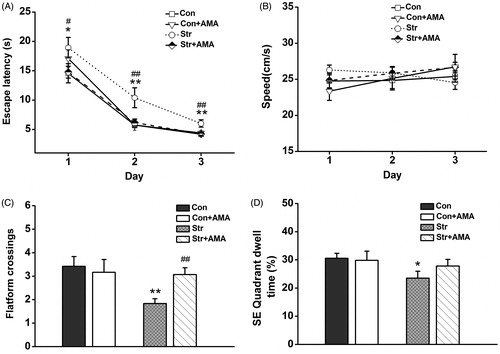
In the SET stage, the reference memory was evaluated. A two-way factorial ANOVA revealed that there were significant differences for the stress effect [F(1,40) = 5.343, p < 0.05], the drug effect [F(1,40) = 3.899, p < 0.05] and the stress × drug interaction effect [F(1,40) = 4.791, p < 0.05] on the platform crossings. It was further found that there was a significant difference for the effect of stress × drug interaction [F(1,40) = 7.262, p < 0.01] on the NW quadrant dwell time. The LSD post hoc test showed that both platform crossings (p < 0.001, ) and NW quadrant dwell time (p < 0.01, ) were significantly decreased in the Str group compared to the Con group. However, the above indicators were remarkably enhanced by administration of AMA (, p < 0.01).
With respect to the RT stage, the platform was moved into the contralateral quadrant to examine the learning flexibility. Average escape latencies are visibly decreased in all the groups (), indicating that animals learnt to find the new platform position following 3 days of training. A three-way repeated measures ANOVA showed that there were no statistical differences for day × stress interaction [F(2,39) =1.045, p > 0.05], day × drug interaction [F(2,39) = 0.695, p > 0.05] and day × stress × drug interaction [F(2,39) =13.496, p > 0.05], except for day [F(2,39) = 183.601, p < 0.001]. However, it was found that there was a significant difference for the effect of stress × drug interaction [F(1,40) = 5.979, p < 0.05] on the escape latencies. Subsequent LSD post hoc analysis showed that the escape latencies were longer in the Str group than that in the Con group (; p < 0.05 on the 1st day; p < 0.01 on the 2nd day; p < 0.01 on the 3rd day). However, the rats in the Str + AMA group spent a much shorter time finding the platform than those in the Str group (p < 0.05 on the 1st day; p < 0.01 on the 2nd day; p < 0.01 on the 3rd day). Similarly, no differences in swimming speed were observed between these groups (, p > 0.05).
During the RET stage, a two-way factorial ANOVA showed that there were significant differences for the effect of stress [F(1,40) = 5.319, p < 0.05] and the effect of stress × drug interaction [F(1,40) = 4.181, p < 0.05] on the platform crossings. In addition, there was only a significant difference for the effect of stress [F(1,40) = 3.253, p < 0.05] on SE quadrant dwell time. The LSD post hoc analysis showed that the both platform crossings (p < 0.01, ) and SE quadrant dwell time (p < 0.05, ) were significantly decreased in the Str group compared to those in the Con group. However, the platform crossings in the Str + AMA group were significantly increased (p < 0.01) compared to those in the Str group, while there was no significant difference for quadrant dwell time between these two groups. Additionally, there were no significant differences of behavior measurements between the Con group and the Con + AMA group in MWM test (p > 0.05).
Effects of AMA administration on the LTP and depotentiation in stressed rats
In the LTP test, stimulation of Schaffer collaterals evoked basal field excitatory postsynaptic potentials (fEPSPs) in the hippocampal CA1 region, while TBS stimulation induced LTP of the stimulated synapses for 1 h. The time course of fEPSPs slopes that has been normalized to the 20 min baseline period is shown in left). It could be seen that the fEPSPs slopes were increased immediately after stimulation, and then more or less stabilized to a level above the baseline period. The last 15 min data were analyzed by two-way factorial AVONA. The results showed that there were significant differences for the effect of stress [F(1,21) = 12.365, p < 0.01], drug [F(1,21) = 6.293, p < 0.05] and stress × drug interaction [F(1,21) = 10.023, p < 0.01] on the fEPSPs slopes. Bonferroni post hoc analysis showed that the mean fEPSPs slopes were significantly smaller in the Str group than that in both the Con group (p < 0.001) and the Str + AMA group (p < 0.01, ). There was no significant difference of the mean fEPSPs slope between the Con and Con + AMA groups ().
Figure 4. The effects of chronic unpredictable stress and amantadine treatment on the long-term potentiation and depotentiation from Schaffer collaterals to the hippocampal CA1. (A) The changes of time coursing in fEPSPs slopes in four groups. The first 20 min of evoked responses were normalized and used as the baseline responses of LTP. The last 15 min of evoked responses during LTP were normalized and used as the baseline responses of depotentiation. (B) Magnitude of LTP was determined as responses between 45 and 60 min after the TBS. (C) Magnitude of depotentiation was determined as responses between 45 and 60 min after low-frequency stimulation. Data represent mean ± SEM. *p < 0.05, **p < 0.01, ***p < 0.001 comparison between the Con group and the Str group; #p < 0.05, ##p < 0.01, ###p < 0.001 comparison between the Str group and the Str + AMA group; the Con, Str and Str + AMA groups, n = 7; the Con + AMA group, n = 4.
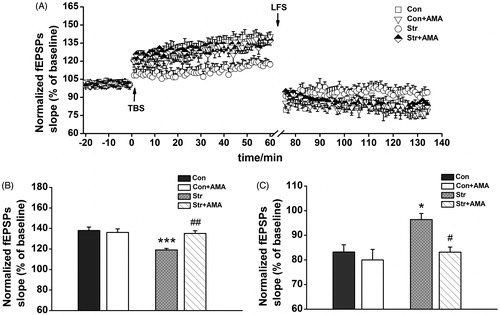
To examine whether depotentiation, a form of LTP reversal, was involved in the process, an LFS induction protocol was employed for eliciting depotentiation (, right). Since there have been significant differences of fEPSPs slopes between stressed rats and normal ones before the LFS, the observed differences after the LFS cannot be used to properly evaluate the effect of CUS + isolated feeding or AMA treatment on synaptic plasticity. Accordingly, LTP-evoked responses in the last 15 min were normalized and used as the baseline of depotentiation (, left). A two-way factorial AVONA confirmed the statistically significant differences for the effect of stress [F(1,21) = 8.084, p < 0.01] and the effect of drug [F(1,21) = 8.225, p < 0.01]. Bonferroni post hoc test indicated that there was significantly suppressive depotentiation in the Str group compared to that in the Con group (p < 0.05), while a considerably facilitated depotentiation existed in the Str + AMA group compared to that in the Str group (p < 0.05, ). Furthermore, there was also no significant difference of the depotentiation between the Con group and Con + AMA group (p > 0.05).
Effects of AMA administration on NR2B and PSD-95 expression in stressed rats
In the Western-blotting assay test, two prominent bands at ∼180 and 95 kDa were detected by NR2B and PSD-95 antibodies, respectively (). A two-way factorial AVONA showed that there were significant differences for the effect of stress [F(1,25) = 8.422, p < 0.01] and the effect of stress × drug interaction [F(1,25) = 5.423, p < 0.05] on the NR2B expression. Subsequently, the LSD post hoc analysis showed that the expression of hippocampal NR2B was significantly decreased in the Str group compared to that in the Con group (p < 0.01, ). It was further found that the expression of NR2B was significantly enhanced by amatadine (p < 0.05 between the Str + AMA group and the Str group, ).
Figure 5. The effects of chronic unpredictable stress and amantadine treatment on expression of the NR2B and PSD-95 in the hippocampus. (A) Results are immunoblots from single representative experiments. (1) the Control group, (2) the Str group, (3) the Str + AMA group, (4) the Con + AMA group. The expression values of the NR2B (B) and the PSD-95 (C) were normalized with β-actin value, then compared to control. Data are presented as mean ± SEM. **p < 0.01, ***p < 0.001, comparison between the Con group and the Str group; #p < 0.05, ##p < 0.01, comparison between the Str group and the Str + AMA group; the Con, Str and Str + AMA groups: PSD-95, n = 7/group; NR2B, n = 8/group; the Con + AMA group: PSD-95/NR2B, n = 5.
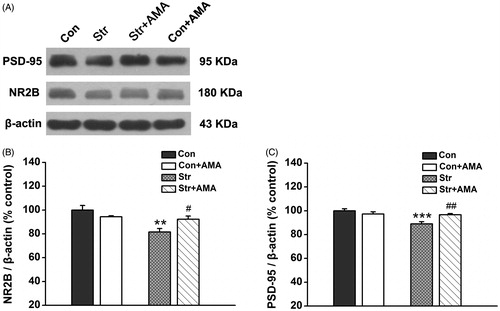
Moreover, A two-way factorial AVONA showed that there were significant differences for the effect of stress [F(1,22) = 13.781, p < 0.01] and the effect of stress × drug interaction [F(1,22) = 11.139, p < 0.01] on the expression of hippocampal PSD-95. LSD post hoc analysis showed that the expression of PSD-95 was significantly decreased in the Str group compared to that in the Con group (p < 0.001, ), while the expression of PSD-95 was significantly enhanced by AMA (p < 0.01 between the Str + AMA group and the Str group, ).
Discussion
The present study demonstrated that the NMDA receptor antagonism effectively impeded the development of depressive-like symptoms in a rat model of chronic stress after chronic AMA administration for 20 consecutive days. More importantly, the spatial memory deficits including spatial learning and re-acquisition in reversal learning were effectively attenuated by AMA treatment. The impairment of synaptic plasticity induced by CUS + isolated feeding was considerably delayed by AMA administration. Finally, AMA treatment up-regulated the expression of NR2B and PSD-95 in the hippocampus of stressed rats.
It is well known that the CUS animal model can successfully simulate depression-like symptoms with decreased body weight and sucrose preference, which are the core symptoms of depression. Accordingly, our study further supports the validity of CUS as an animal model to study depression, consistent with the previously reported (Willner, Citation1997). After establishing a rat model of chronic stress, significant differences were observed in body weight and sucrose consumption between the Con group and the Str group. Moreover, the data showed that there was no statistical difference of total fluid intake among these groups. A previous study showed that reduced intake of a sucrose preference was a sign of anhedonia, or a decrease in the rewarding properties of the solution (Willner, Citation2005). Thus, our data indicate that the CUS rat model, combined with isolation rearing, simulated depression-like behavior.
AMA treatment caused significant antidepressant-like effect on depression related behaviors, similar to our previous findings related to memantine treatment (Quan et al., Citation2011b). An earlier study reported that AMA produced antidepressant-like activity, as shown by the decreased immobility time in the forced swim test (used to assess the behavioral despair, symptom of depression) (Moryl et al., Citation1993). Additionally, clinical data demonstrate that AMA exerts an antidepressant action in patients with Parkinson’s disease as well as in depressed patients (Zarate et al., Citation2002).
To evaluate whether AMA could impede the development of cognitive dysfunction in a rat model of chronic stress, we performed the MWM test to assess spatial memory. In both the IT and SET stages, our data indicated prolonged response latency and reductions in both quadrant dwell time and platform crossings in stressed rats relative to controls, indicating that spatial learning and reference memory were significantly impaired in the stressed rats. This finding is in line with that of previous studies (Henningsen et al., Citation2009; Mizoguchi et al., Citation2000). It has been reported that exposure to CUS over several weeks could produce a marked long-term deficit in learning and memory in rats (Conrad, Citation2010). Importantly, rats in the Str + AMA group required less time to find the platform, and platform crossings and quadrant dwell time were significantly enhanced compared to that in the Str group, suggesting that AMA could effectively impede the impairments of cognition induced by stress. The results were in accordance with the findings of previous studies, demonstrating that chronic administration of AMA could attenuate water maze performance deficits resulting from traumatic brain injury (Dixon et al., Citation1999; Wang et al., Citation2014). Furthermore, in both the RT and RET stages, the hidden platform was moved into the contralateral quadrant to examine learning flexibility, which can be attributed to erasure of previously acquired information and development of a new memory. Our data showed that the stressed rats failed to adjust the search strategy in response to the variation of platform position and resulted in impaired re-acquisition of a learned skill, indicating that the cognitive flexibility was significantly impaired. However, the escape latencies in the Str + AMA group were greatly reduced, suggesting that AMA could effectively improve cognitive flexibility. This is also in line with the findings of our previous study, in which memantine was applied in the treatment of stressed rats (Quan et al., Citation2011b). It is crucial to broaden our current knowledge of the potential effect of AMA on the potential treatment of cognitive dysfunction, not only as it shows a positive effect in traumatic brain injury (Dixon et al., Citation1999) and Parkinson’s patients (Cera et al., Citation2014) but also in stress-induced cognitive deficits.
Since LTP is recognized as the cellular basis of learning and memory (Bliss & Collingridge, Citation1993), LTP from Schaffer collaterals to hippocampal CA1 region was measured in the present study. A higher fEPSPs slope is commonly associated with more effective synaptic transmission and better learning and memory. Previous studies report that MWM training could increase the glutamate release from the presynaptic membrane, implying that behavioral training might enhance synaptic transmission (Richter-Levin et al., Citation1995, Citation1998). In the present study, behavioral training was performed before the LTP/depotentiation recordings for all the rats of four groups. The electrophysiological results showed that the strength of synaptic connections was significantly weaker in stressed rats, as shown by reduced fEPSPs slopes relative to controls. The data also illustrated that synaptic plasticity could be impaired in the hippocampus after prolonged exposure to aversive events (Alfarez et al., Citation2003). Interestingly, the negative effect of stress on LTP was remarkably attenuated by chronic AMA treatment, which may be related to the improvements in cognitive deficits in stressed rats. Hippocampal LTP is thought to be a cellular mechanism of learning and memory; however, the effect of depotentiation could be another crucial synaptic plasticity to erase memory by selectively reversing experience-dependent plasticity (Neves et al., Citation2008). Furthermore, depotentiation plays a role in the time- and state-dependent erasure of memory and enables the storage of new information by the hippocampus (Qi et al., Citation2013). In the present study, it was found that depotentiation was significantly suppressed in stressed rats, indicating that the resilience of synaptic structures was seriously damaged. This impairment of synaptic plasticity was greatly impeded by AMA intervention, consistent with improved performance in reversal learning of MWM. One of our previous studies showed that the balance between LTP and depotentiation played a key role in cognitive stability and flexibility (An et al., Citation2013a). Accordingly, the bidirectional synaptic plasticity, which shows integrated LTP and depotentiation, may be the possible cellular basis of cognitive function in learning and reversal learning of spatial information.
As a functional subunit of NMDA receptor complex, NR2B receptor is very important in LTP induction. It has been shown that memantine improves cognitive dysfunction by mediating the NR2B receptor (Quan et al., Citation2011b). In addition, a previous study reports that the GluN2B subunit-containing NMDA receptor is necessary for LFS to induce depotentiation (Qi et al., Citation2013). Accordingly, we examined expression of the NR2B subunit in the hippocampus. Expression of the NR2B subunit of NMDA receptor was significantly reduced in stressed rats relative to controls, suggesting that there was lower expression of NR2B protein in the depression-like animal model. It might be translated into reduced numbers of functional receptors (Dong et al., Citation2010; Lou et al., Citation2010), which is linked to impaired plasticity. Importantly, the expression of NR2B receptor was significantly enhanced by AMA, which could be part of an underlying mechanism that attenuates impairments of LTP and depotentiation as well as the cognitive deficits induced by chronic stress. Moreover, it is well known that PSD-95 plays a crucial role in the integration of NMDA receptor complexes, since it enables anchoring of NMDA receptors via the combination with NR2 subunits to the postsynaptic membrane. Consequently, PSD-95 may be essential for LTP induction and the regulation of synaptic function (Cousins & Stephenson, Citation2012). Our data showed that PSD-95 was markedly reduced in the hippocampus in the Str group compared to the Con group, which could explain why both LTP and depotentiation were damaged in stressed rats. AMA significantly up-regulated the expression of PSD-95, suggestive of enhanced communication/coupling of the NMDA receptors in the postsynaptic membrane. Consequently, the induction and maintenance of glutamate receptor-dependent plasticity could be preserved by AMA (Li et al., Citation2003). The AMA improvement of synaptic plasticity and resilience may be due to preventing the alteration of these two proteins expression in the hippocampus.
AMA, as a low-affinity NMDA receptor antagonist, has a neuroprotective effect with the “partial trapping” type of channel closure-like memantine, which may protect neurons from the glutamate excitotoxicity and consequently alleviate the cognitive deficits induced by chronic stress (Szakacs et al., Citation2012). Moreover, the fact that the NMDAR antagonists, like memantine, may enhance the cognition is by selectively inhibiting the “pathological activation” while preserving the “physiological activation” of NMDARs (Collingridge et al., Citation2013). Accordingly, we speculate that AMA has more or less similar characteristics of memantine. Additionally, AMA could enhance the release of dopamine (Baldessarini et al., Citation1972) and increase the expression of BNDF (Rogoz et al., Citation2008), which are important neurotransmitters to synaptic plasticity. In addition, NR2B expression may be up-regulated by chronically administrating AMA, which may function as an adaptive mechanism. Thus, AMA attenuation of impairments of synaptic plasticity and cognition following stress may act through several cellular mechanisms.
Additionally, a previous study showed that the low dose of AMA (15 mg/kg, 30 mg/kg, i.p.) was unable to impair memory, locomotion, exploration or motivation in mice, but the high dose (60 mg/kg) could produce neurotoxicity (Kaefer et al., Citation2010). Moreover, it was reported that the dose of AMA in human was in an average dose of 200 mg/day but not exceeding 400 mg/day (Hosenbocus & Chahal, Citation2013). The AMA dose used in the present study is converted from animal to human equivalently, based on the body surface area on the recommended daily dose of 200 mg in human (Nishikawa et al., Citation2009; Reagan-Shaw et al., Citation2008). Accordingly, we argue that AMA at a dose of 25 mg/kg generates no significant effect on either the LTP/depotentiation or behavior in the absence of prior stress exposure.
Conclusion
In summary, our results suggest that AMA at a dose of 25 mg/kg is effective in impeding the symptom of weight loss and anhedonia in the rat model of chronic stress, exhibiting potential antidepressant-like properties. Moreover, it significantly attenuates the impairments of synaptic plasticity and cognitive functions seen following chronic stress. A potential underlying mechanism is that AMA prevents the alterations of NR2B receptor and PSD-95 in the hippocampus of stressed rats. Nevertheless, further investigation is needed to explore the possible mechanism of NMDA antagonists in antidepressant efficacy. In conclusion, the data suggest that there are possible novel therapeutic targets for antidepressant drug development as well as a possibility for treating cognitive dysfunction in stress-related diseases.
Acknowledgements
We are grateful to Drs Xiaxia Xu and Ning Cheng for their kindly assistance in performing statistical analysis.
Declaration of interest
This work was supported by grants from the National Natural Science Foundation of China (31171053, 11232005), Funds for National Basic Science Personnel Training (J1103503) and 111 Project (B08011). The authors have no conflict of interest to declare.
References
- Airaksinen E, Larsson M, Lundberg I, Forsell Y. (2004). Cognitive functions in depressive disorders: evidence from a population-based study. Psychol Med 34:83–91
- Alfarez DN, Joels M, Krugers HJ. (2003). Chronic unpredictable stress impairs long-term potentiation in rat hippocampal CA1 area and dentate gyrus in vitro. Eur J Neurosci 17:1928–34
- An L, Yang Z, Zhang T. (2013a). Imbalanced synaptic plasticity induced spatial cognition impairment in male offspring rats treated with chronic prenatal ethanol exposure. Alcohol Clin Exp Res 37:763–70
- An L, Yang Z, Zhang T. (2013b). Melamine induced spatial cognitive deficits associated with impairments of hippocampal long-term depression and cholinergic system in Wistar rats. Neurobiol Learn Mem 100:18–24
- Baldessarini RJ, Lipinski JF, Chace KV. (1972). Effects of amantadine hydrochloride on catecholamine metabolism in the brain of the rat. Biochem Pharmacol 21:77–87
- Bliss TV, Collingridge GL. (1993). A synaptic model of memory: long-term potentiation in the hippocampus. Nature 361:31–9
- Casarotto PC, Andreatini R. (2007). Repeated paroxetine treatment reverses anhedonia induced in rats by chronic mild stress or dexamethasone. Eur Neuropsychopharmacol 17:735–42
- Cera N, Bifolchetti S, Martinotti G, Gambi F, Sepede G, Onofrj M, Di Giannantonio M, Thomas A. (2014). Amantadine and cognitive flexibility: decision making in Parkinson’s patients with severe pathological gambling and other impulse control disorders. Neuropsychiatr Dis Treat 10:1093–101
- Collingridge GL, Volianskis A, Bannister N, France G, Hanna L, Mercier M, Tidball P, et al. (2013). The NMDA receptor as a target for cognitive enhancement. Neuropharmacology 64:13–26
- Conrad CD. (2010). A critical review of chronic stress effects on spatial learning and memory. Prog Neuropsychopharmacol Biol Psychiatry 34:742–55
- Cousins SL, Stephenson FA. (2012). Identification of N-methyl-D-aspartic acid (NMDA) receptor subtype-specific binding sites that mediate direct interactions with scaffold protein PSD-95. J Biol Chem 287:13465–76
- Dixon CE, Kraus MF, Kline AE, Ma X, Yan HQ, Griffith RG, Wolfson BM, Marion DW. (1999). Amantadine improves water maze performance without affecting motor behavior following traumatic brain injury in rats. Restor Neurol Neurosci 14:285–94
- Dong J, Min S, Wei K, Li P, Cao J, Li Y. (2010). Effects of electroconvulsive therapy and propofol on spatial memory and glutamatergic system in hippocampus of depressed rats. J ECT. 26:126–30
- Ehrlich I, Klein M, Rumpel S, Malinow R. (2007). PSD-95 is required for activity-driven synapse stabilization. Proc Natl Acad Sci USA 104:4176–81
- Fox CJ, Russell KI, Wang YT, Christie BR. (2006). Contribution of NR2A and NR2B NMDA subunits to bidirectional synaptic plasticity in the hippocampus in vivo. Hippocampus 16:907–15
- Henningsen K, Andreasen JT, Bouzinova EV, Jayatissa MN, Jensen MS, Redrobe JP, Wiborg O. (2009). Cognitive deficits in the rat chronic mild stress model for depression: relation to anhedonic-like responses. Behav Brain Res 198:136–41
- Hosenbocus S, Chahal R. (2013). Amantadine: a review of use in child and adolescent psychiatry. J Can Acad Child Adolesc Psychiatry 22:55–60
- Huber TJ, Dietrich DE, Emrich HM. (1999). Possible use of amantadine in depression. Pharmacopsychiatry 32:47–55
- Kaefer V, Semedo JG, Kahl VFS, Von Borowsky RG, Gianesini J, Kist TBL, Pereira P, Picada JN. (2010). DNA damage in brain cells and behavioral deficits in mice after treatment with high doses of amantadine. J Appl Toxicol 30:745–53
- Kim JJ, Diamond DM. (2002). The stressed hippocampus, synaptic plasticity and lost memories. Nat Rev Neurosci 3:453–62
- Li B, Otsu Y, Murphy TH, Raymond LA. (2003). Developmental decrease in NMDA receptor desensitization associated with shift to synapse and interaction with postsynaptic density-95. J Neurosci 23:11244–54
- Lou JS, Li CY, Yang XC, Fang J, Yang YX, Guo JY. (2010). Protective effect of gan mai da zao decoction in unpredictable chronic mild stress-induced behavioral and biochemical alterations. Pharm Biol 48:1328–36
- Marsden WN. (2013). Synaptic plasticity in depression: molecular, cellular and functional correlates. Prog Neuro Psychopharmacol Biol Psychiatry 43:168–84
- McEwen BS, Magarinos AM. (2001). Stress and hippocampal plasticity: implications for the pathophysiology of affective disorders. Hum Psychopharmacol 16:S7–19
- Mizoguchi K, Yuzurihara M, Ishige A, Sasaki H, Chui DH, Tabira T. (2000). Chronic stress induces impairment of spatial working memory because of prefrontal dopaminergic dysfunction. J Neurosci 20:1568–74
- Moryl E, Danysz W, Quack G. (1993). Potential antidepressive properties of amantadine, memantine and bifemelane. Pharmacol Toxicol 72:394–7
- Neves G, Cooke SF, Bliss TV. (2008). Synaptic plasticity, memory and the hippocampus: a neural network approach to causality. Nat Rev Neurosci 9:65–75
- Nishikawa N, Nagai M, Moritoyo T, Yabe H, Nomoto M. (2009). Plasma amantadine concentrations in patients with Parkinson’s disease. Parkinsonism Relat Disord 15:351–3
- Paxinos G, Watson C. The rat brain in stereotaxic coordinates. Burlington, MA: Elsevier Academic Press; 2005
- Qi Y, Hu NW, Rowan MJ. (2013). Switching off LTP: mGlu and NMDA receptor-dependent novelty exploration-induced depotentiation in the rat hippocampus. Cereb Cortex 23:932–9
- Quan M, Zheng C, Zhang N, Han D, Tian Y, Zhang T, Yang Z. (2011a). Impairments of behavior, information flow between thalamus and cortex, and prefrontal cortical synaptic plasticity in an animal model of depression. Brain Res Bull 85:109–16
- Quan MN, Zhang N, Wang YY, Zhang T, Yang Z. (2011b). Possible antidepressant effects and mechanisms of memantine in behaviors and synaptic plasticity of a depression rat model. Neuroscience 182:88–97
- Reagan-Shaw S, Nihal M, Ahmad N. (2008). Dose translation from animal to human studies revisited. FASEB J 22:659–61
- Richter-Levin G, Canevari L, Bliss TV. (1995). Long-term potentiation and glutamate release in the dentate gyrus: links to spatial learning. Behav Brain Res 66:37–40
- Richter-Levin G, Canevari L, Bliss TV. (1998). Spatial training and high-frequency stimulation engage a common pathway to enhance glutamate release in the hippocampus. Learn Mem 4:445–50
- Rogoz Z, Skuza G, Daniel WA, Wojcikowski J, Dudek D, Wrobel A. (2007). Amantadine as an additive treatment in patients suffering from drug-resistant unipolar depression. Pharmacol Rep 59:778–84
- Rogoz Z, Skuza G, Legutko B. (2008). Repeated co-treatment with fluoxetine and amantadine induces brain-derived neurotrophic factor gene expression in rats. Pharmacol Rep 60:817–26
- Sapolsky RM. (2000). Glucocorticoids and hippocampal atrophy in neuropsychiatric disorders. Archiv Gen Psychiatry 57:925–35
- Stryjer R, Strous RD, Shaked G, Bar F, Feldman B, Kotler M, Polak L, et al. (2003). Amantadine as augmentation therapy in the management of treatment-resistant depression. Int Clin Psychopharmacol 18:93–6
- Szakacs R, Janka Z, Kalman J. (2012). The “blue” side of glutamatergic neurotransmission: NMDA receptor antagonists as possible novel therapeutics for major depression. Neuropsychopharmacol Hung 14:29–40
- Vorhees CV, Williams MT. (2006). Morris water maze: procedures for assessing spatial and related forms of learning and memory. Nat Protoc 1:848–58
- Wang T, Huang XJ, Van KC, Went GT, Nguyen JT, Lyeth BG. (2014). Amantadine improves cognitive outcome and increases neuronal survival after fluid percussion traumatic brain injury in rats. J Neurotrauma 31:370–7
- Willner P. (1997). Validity, reliability and utility of the chronic mild stress model of depression: a 10-year review and evaluation. Psychopharmacology 134:319–29
- Willner P. (2005). Chronic mild stress (CMS) revisited: consistency and behavioural-neurobiological concordance in the effects of CMS. Neuropsychobiology 52:90–110
- Xu P, Xu J, Li Z, Yang Z. (2012). Expression of TRPC6 in renal cortex and hippocampus of mouse during postnatal development. PLoS One 7:e38503
- Zarate CA, Quiroz J, Payne J, Manji HK. (2002). Modulators of the glutamatergic system: implications for the development of improved therapeutics in mood disorders. Psychopharmacol Bull 36:35–83