Abstract
Objective: Statins proved potential bone healing properties. Rosuvastatin is a synthetic, hydrophilic, potent and highly efficacious statin. In the current work, an attempt was investigated to develop, evaluate various bioerodible composite sponges enclosing rosuvastatin and explore their potential in augmenting bone healing and regeneration.
Method: Twelve lyophilized sponge formulae were prepared adapting a 41.31 full factorial design. Xanthan gum, polycarbophil, Carbopol® and sodium alginate were investigated as anionic polymers, each at three chitosan:anionic polymer ratios (1:3, 1:1, 3:1). The formula of choice was implanted in fractured rat femora.
Results: Visual and microscopic examination showed flexible homogenous porous structures with considerable bending ability. Polyelectrolyte complex formation was proved by DSC and FT-IR for all chitosan/anionic combinations except with xanthan gum where chitosan probably bound to the drug rather than xanthan gum. Statistical analysis proved that anionic polymer type and chitosan: polymer ratio, as well as, their interactions, exhibited significant effects on the release parameters at p ≤ 0.05. The optimum chitosan/anionic polymer complexation ratios were 3:1 for polycarbophil and 1:1 for Carbopol and alginate. The release at these ratios followed Fiction diffusion while other ratios had anomalous diffusion. Imwitor® 900K and HPMC K100M were added as release retarardants for further release optimization. The formula of choice was implanted in fractured rat femora. Histopathological examination revealed advanced stages of healing in treated femora compared to control ones.
Conclusion: Biodegradable sponges for local rosuvastatin delivery proved significantly enhanced wound healing and regeneration properties to fractured bones.
Introduction
Bone anabolic agents are ideal treatments for diseases of bone loss such as osteoporosis, for bone fractures, as well as, for serious bone defects following severe trauma or tumor resection. Such bone related musculoskeletal problems are highly associated with physical disabilities and morbidities (Monjo et al., Citation2010b). Being inhibitors of the 3-hydroxy-3-methylglutaryl coenzyme A reductase enzyme, statins were investigated as bone forming molecules. In vitro and in vivo studies have proven a stimulant effect of statins in bone formation and fracture healing both in animals (Mundy et al., Citation1999; Oxlund & Andreassen, Citation2000; Wilkie et al., Citation2000) and humans (Sugiyama et al., Citation2000; Maeda et al., Citation2001). This effect comes directly through activation of bone morphogenic protein (BMP-2) and TGF-b1 (Mundy et al., Citation1999; Nyan et al., Citation2010; Stojadinovic et al., Citation2010) as well as, indirectly by the suppression of osteoclast activity (Hughes et al., Citation2007; Ayukawa et al., Citation2009; Luisetto & Camozzi, Citation2009; Moriyama et al., Citation2010). Studies proved that this control of bone remodeling process by statins was prominent after subcutaneous, oral and local administration (Oxlund & Andreassen, Citation2004; Gutierrez et al., Citation2006; Moriyama et al., Citation2008). Local application could reduce the side effects and eliminate the doubts about the biodistribution of parenteral and oral statins to bone (Hirabayashi & Fujisaki, Citation2003; Miyamoto et al., Citation2008). Both hydrophilic and hydrophobic statins were thoroughly investigated for their bone healing and regeneration effects; the most investigated of which were simvastatin, fluvastatin, cerivastatin, lovastatin and pravastatin (Wilkie et al., Citation2000; Gutierrez et al., Citation2006; Hughes et al., Citation2007; Nyan et al., Citation2007,Citation2010; Moriyama et al., Citation2010; Stojadinovic et al., Citation2010). Rosuvastatin, as a synthetic, hydrophilic, potent and highly efficacious statin has proven favorable pharmacologic properties compared to other statins including minimal metabolism, reduced hepatic selectivity and enhanced inhibition of HMG-CoA reductase (McTaggart et al., Citation2001; Hughes et al., Citation2007; Monjo et al., Citation2010a; Stojadinovic et al., Citation2010). Recently, work by Monjo et al., (Citation2010b) showed that rosuvastatin, as other statins, promoted BMP-2 expression and osteoblast differentiation in vitro and potentially helped in the local treatment of bone defects in rabbits. Yet, literature lacks detailed investigations about statin formulation and optimization for this new use.
This study will investigate polyelectrolyte complex sponge matrices as carriers for rosuvastatin. Chitosan will be examined as the polycationic part with carbopol, polycarbophil, xanthan gum and sodium alginate as polyanionic polymers. The obtained sponges will be tested for complex formation and in vitro evaluated for fulfilling the requirements of sustained release local delivery systems. Further, bone healing and regeneration potential will be evaluated in rats after local application.
Materials and methods
Materials
Rosuvastatin was kindly gifted by AstraZeneca, chitosan, medium MW, 85% degree of deacteylation, viscosity of 1% solution in 1% acetic acid at 25 °C is 550 cp, sodium alginate and xanthan gum were purchased from Sigma-Aldrich Chemical Co. (St. Louis, MO). Carbopol® 974P NF (Carbomer Homopolymer 934P, type B, 25 000–45 000 cp for 0.5%) and Polycarbophil, NOVEON® AA-1 POLYCARBOPHIL were kind gifts from Noveon (Calvert city, KY). Glacial acetic acid (Merck, Darmstadt, Germany). Imwitor® 900K (glyceryl monostearate) was kindly gifted from Sasol, Germany, and HPMC, METHOCEL™ K100M Premium (COLORCON, UK). Other chemicals were of pharmaceutical or analytical grades and were used as received.
Preparation of rosuvastatin-loaded sponges
Preliminary studies were conducted examining various polyanionic polymers, as well as, different chitosan: polyanionic polymer ratios to find the optimum levels for developing promising drug-loaded sponges.
Twelve sponge formulae were prepared adapting a 41.31 full factorial design. Xanthan gum, polycarbophil, Carbopol® and sodium alginate were investigated as anionic polymers, each at a time (independent variable X1). The sponges were prepared at three chitosan:anionic polymer ratios of 1:3, 1:1 and 3:1 (independent variable X2). Composition of the prepared formulae is shown in .
Table 1. Composition and evaluation of rosuvastatin sponge formulae prepared adapting a 41.31 full factorial design.
Initially, 1% w/v stock gels of chitosan and each of the anionic polymers were prepared in 1% acetic acid and distilled water, respectively. The suitable weights of stock gels were mixed by magnetic stirring to form a total weight of 20 g for each sponge formula. The drug was dissolved in the anionic polymer gels prior to mixing. Stirring time was fixed at 30 min at ambient conditions. An accurately weighed amount of 2 g of the prepared drug loaded cationic/anionic gels (equivalent to 2.5 mg drug) were poured into plastic blister molds and frozen at −20 °C for 24 h. The samples were lyophilized using a Novalyphe-NL 500 freeze-dryer (Savant Instruments, Farmingdale, NY) at a condenser temperature of −45 °C and a pressure of 7 × 102 mbar for 24 h. The obtained sponges were stored in desiccators until use.
Evaluation of rosuvastatin-loaded sponges
Physical assessment and microscopic examination
Lyophilized sponge formulae were visually inspected for physical integrity, texture, shape, color, structural defects, softness and plasticity. Thin layers of 1–2 mm were prepared and observed under light microscope (Leica Image Analyzer: Q5501 W, Cambridge, England). Photographs were captured at suitable magnifications for xanthan gum-based, polycarbophil-based, Carbopol®-based and Na alginate-based sponges, all at 1:1 chitosan to anionic polymer ratio.
Uniformity of rosuvastatin-loaded sponges
Six sponges of each formula were randomly selected and accurately weighed. The mean weight values and % RSD were calculated. Drug content uniformity was determined by cutting and soaking each sponge formula overnight at 25 °C in 100 mL of phosphate buffer (pH 7.4). The samples were filtered through a cellulose acetate membrane filter (0.45 μm) and rosuvastatin concentration was determined spectrophotometrically at λmax 244 nm (1601-PC double beam spectrophotometer, Shimadzu, Kyoto, Japan) (Orlu et al., Citation2006; Moffat et al., Citation2011). Experiments were conducted in triplicates. The acceptance values (AV) were calculated using the following equation:
where M is label claim (100%), X the average (%) of individual contents, k the acceptability constant (2.2) and s is the standard deviation. Acceptance values, according to the British Pharmacopeia 2007, should be less than 15 (British Pharmacopoeial Commission, Citation2007).
Mechanical characteristics
The tensile properties of the drug-loaded sponges were determined after storing freshly prepared sponges over CaCl2 at 25 °C for 1 week to equilibrate (Instron® mechanical strength testing machine 3345, GmbH, Germany). Sponge samples were fixed between the apparatus clamps and the force at tearing and elongation were determined. The percent elongations at break (% E) and elastic modulus (EM) were computed as follows:
where Lo is the initial length of the sample, L is its length after elongation, F is the breaking load (N) and A is the cross-sectional area of the sponge formulation. An average of five determinations was taken for each formula.
Differential scanning calorimetry (DSC)
The thermotropic properties and phase transition behavior of rosuvastatin, chitosan, anionic polymers, 1:1 cationic/anionic polymer physical mixtures and drug loaded sponge formulae were evaluated using Differential Scanning Calorimeter, DSC-50 (Shimadzu, Kyoto, Japan). The apparatus was calibrated using purified indium (99.9%) and samples of about 5 mg were sealed in a 50 μl flat-bottomed aluminum pans at a constant heating rate of 10 °C/min throughout the analysis over a temperature range of 30–330 °C. Empty aluminum pans were used as references and the whole thermal behaviors were studied under a nitrogen purge (30 mL/min).
Fourier transform infrared spectroscopy (FT-IR)
To inspect the possible chemical interactions between the sponge components, the FT-IR spectra of the samples were scanned, at a signal/noise ratio of 30 000:1, using the potassium bromide (KBr) disc technique via FT-IR spectrophotometer (IR Affinity-1, Shimadzu, Kyoto, Japan). Briefly, the powdered samples were mixed with KBr and compressed into a thin disk using a hydraulic pressing machine. The FT-IR spectrum of each KBr disk was then measured in the spectral region 4000–400 cm−1. Smoothing of the spectra and the baseline correlation procedures were applied.
In vitro release study and mathematical modeling
The in vitro release profile of rosuvastatin from different drug loaded sponges was studied using USP dissolution apparatus with basket (Hanson Research Dissolution tester, Chatsworth, CA). The test was performed in 150 mL phosphate buffer of pH 7.4. The apparatus was operated at 37 ± 0.5 °C and 50 rpm. Samples were withdrawn at predetermined time intervals of 0.5, 1, 2, 3, 4, 6 and 8 h. They were filtered and analyzed spectrophotometrically.
The median dissolution time (MDT) (Lindner and Lippold n.d.) and the release efficiency from 0 to 8 h (RE8h) (Modi & Tayade, Citation2006) were calculated as estimates for the release rate and release extent, respectively.
Statistical analysis was performed to evaluate the individual and combined influences of the tested independent variables on the selected evaluation responses (MDT and RE8h) according to the established factorial design using Design-Expert software Version. 7.0.0 (Stat-Ease Inc., Minneapolis, MN).
The release data were fitted to Higuchi equation and its modified forms with burst release (F0) and lag time (Tlag) using the excel add-in software package, DDSolver. The adjusted coefficient of determination (R2adjusted) was considered for assessing the appropriateness of a model. The release mechanism was described in terms of the exponent (n) values in Korsmeyer–Peppas model (Korsmeyer et al., Citation1983). In addition, the release data were fitted to Baker–Lonsdale model (Baker & Lonsdale, Citation1974). The Baker–Lonsdale model is developed from the Higuchi model to describe drug release as a diffusion process based on the Fickian law (Higuchi, Citation1961,Citation1963).
Release profile optimization
In order to further optimize rosuvastatin release from the formulated sponge, hydrophobicity- imparting agents (Imwitor® 900K and HPMC K100M) were added to the selected sponge formulae, each at two concentrations (Formulae F13-19, ). The optimizing additives were dispersed after complete mixing of both cationic and anionic polymers with stirring for further 30 min. The formed gels were then poured into molds and freeze dried. The optimized formulae were evaluated for their release profiles. The desirability function was used for optimization according to Derringer & Suich (Citation1980). The targeted release profile should have the highest MDT value and the lowest RE8h.
Table 2. Release kinetics and mechanism of the release data of rosuvastatin from the prepared sponges analyzed using DDsolver software.
Sterilization
The formula of choice was sterilized by gamma radiation in cobalt-60 Gamma Chamber 4000-A. Sponges were placed separately in sealed plastic bags and irradiated at a dose of 25 KGy (Egyptian Atomic Energy Authority). The sterilized sponges were re-evaluated for their appearance, drug content and in vitro release profile and kinetics. The similarity factor f2 (Shah et al., Citation1998) was calculated from the mean release data and used to evaluate the effect of sterilization on the release profile.
In vivo evaluation of bone regeneration activity of rosuvastatin-loaded sponge
Study design and surgical procedure
Sixteen Wister rats with an average weight of 257 ± 41.2 g were used in this study (supplied by the Laboratory Animal Center of Faculty of Pharmacy, Cairo University, Egypt). The rats were treated according to our institution’s guidelines for animal care and the study protocol was submitted and approved (PI 708) by the Research Ethics Committee for experimental and clinical studies at Faculty of Pharmacy, Cairo University, Cairo, Egypt (REC-FOPCU). During the study, animals were housed individually in standard rat cages with an ambient temperature of 24–26 °C, and a 12/12 h light/dark cycle, and they were provided with free access to drinking water and commercially available standard rodent food.
The bone regeneration effect of the optimum sponge formulae (F16) was assessed on fractured rats’ femurs. For each rat, one side was used to study the effect of rosuvastatin sponge implantation on the fracture healing while the other side was used as a control. The surgery was performed under chloral hydrate anesthesia (300 mg/kg intraperitoneally). Preoperatively, the surgical sites were depilated, washed with soft soap and disinfected.
On the operation site, as presented in , the surgical procedure started by making an incision on the proximal anterior part of both femora, penetrating all soft tissue layers, then each femur was fractured at its mid shaft using an osteotome and a 2-mm segment was removed. Then, both femora were fixed using an intramedullary K-wire of appropriate diameter. In the right femora, rosuvastatin sponge (Formula F16) was inserted into the removed segment space, while the left one was left untreated as a control (only fixed with the k-wire).The soft tissues were repositioned and closed with a 4-0 sutures. The animals received SC analgesic and the surgery sites were treated with an antibiotic and observed till complete healing. After 1 month, the rats were euthanized and autopsy samples were taken from the right and left femora of each rat and fixed in 10% formaldehyde solution for 24 h.
Histological preparation and examination
Bone healing of the fractured sites was assessed via histological examination. Autopsy samples taken from the right and left femora of each rat were fixed in 10% formaldehyde solution for 24 h, Decalcification was done in 5% formic acid followed by washing with tap water. Serial dilutions of alcohol (methyl, ethyl and absolute ethyl) were then used for dehydration. Specimens were cleared in xylene, embedded in paraffin and kept at 56 °C for 24 h. Paraffin beeswax tissue blocks were prepared for sectioning at 4 μm thicknesses by sledge microtome. The obtained tissue sections were collected on glass slides, deparaffinized and stained by hematoxylin/eosin. They were examined under the light electric microscope and photos were taken at suitable magnifications (Bancroft & Gamble, Citation2008).
Results and discussion
Preparation and in vitro evaluation of rosuvastatin-loaded sponges
Polyelectrolyte complex sponges were proposed here as Bioerodible implantable delivery systems for rosuvastatin. A dual osteoinductive effect is expected from the formulated sponge due to the combination of rosuvastatin, with its potential effect in stimulating bone formation, together with chitosan which has proven wound and bone healing and regeneration properties (Venkatesan & Kim, Citation2010). Such dual effect could be achieved through slow erosion and biodegradation of the formulated polyelectrolyte complex to release simultaneously both material of known bone – healing and repairing compounds; rosuvastatin and chitosan. Natural polymer-based sponges have proven success when implanted for wound and bone healing. They ensure faster healing and more patient comfort due to their biodegradable nature and the soft and elastic texture. Actually, the expected crosslinking between polycationic polysaccharide (chitosan) and various polyanionic polysaccharides would suggest sustained release of the drug by the slow swelling and/or erosion of their cross-linked hydrophilic networks, as well as, by the charge–charge and other interactions frequently leading to complex formation (Pavli et al., Citation2011; Ayensu et al., Citation2012).
Physical assessment and microscopic examination
The sponges were easily removed from the molds after lyophilization. They had flexible textures with considerable bending ability without fracture. Visual inspection showed uniform mass and thickness without cracks or structural defects. Increasing chitosan (moving from 1:3 to 1:1, to 3:1 chitosan: polymer ratio) resulted in corresponding increase in the sponge cohesiveness, coherence and formulations of smooth surface, shiny appearance and better physical integrity. This could be due to stronger electrostatic interactions between the positively charged amino groups in chitosan and the negatively charged carboxylic groups in the interacting polymer with the formation of denser matrices. Microscopic examination of all sponge sections showed porous structures, . Chitosan/xanthan gum sponges had striated matrices with large pores, . Chitosan/Carbopol® sponges had the least porosity degrees, . Such porous structures and pore sizes are of surmount importance in sponge formulations as they represent key factors that ensure rapid hydration and gelation and control drug release rate from the polymeric matrix.
Uniformity of rosuvastatin-loaded sponges
The weights of the sponge formulae ranged from 19.88 to 24.38 mg with % relative standard deviations values ranging from 1.63 to 3.52. Such relatively small %RSD indicates the reproducibility of the preparation method. There were no significant weight differences among the formulations (p > 0.05, ANOVA). The drug content ranged from 94.64% to 105.35%. The acceptance values were found to lie between 2.1 and 13.51%.
Mechanical characteristics
The implantable sponges should possess acceptable mechanical strength and hardness. The percentage elongation ranged from 275 to 445.85 cm% for all the prepared sponge formulae which indicates satisfactory toughness and stretch-ability prior to breakage when a strain is applied. Similarly, the calculated elastic modulus values (0.011–0.024) showed acceptable stiffness.
Differential scanning calorimetry (DSC)
As presented in , The DSC thermogram of rosuvastatin had two broad endotherms at 85.4 °C and 222.96 °C showing no definitive melting point (Moffat et al., Citation2011). The DSC thermogram of chitosan showed single broad endothermic and exothermic transitions at 80.7 °C and 308 °C, respectively (Tadros & Fahmy, Citation2014). Carbopol® had two broad endotherms at 59.98 °C and 268.14 °C, polycarbophil showed two shallow endothermic transitions at 55.39 °C and 251.94 °C, xanthan gum had broad endotherm at 95.3 °C and sharp exotherm at 262.2 °C and alginate spectrum showed a broad endotherm at 94.39 °C and characteristic sharp exotherm at 250.34 °C.
Figure 3. DSC thermograms of rosuvastatin, chitosan, anionic polymer, chitosan/anionic polymer physical mixtures and drug laoded sponges for (a) xanthan gum-based, (b) polycarbophil-based, (c) Carbopol®-based and (d) Na alginate-based formulae.
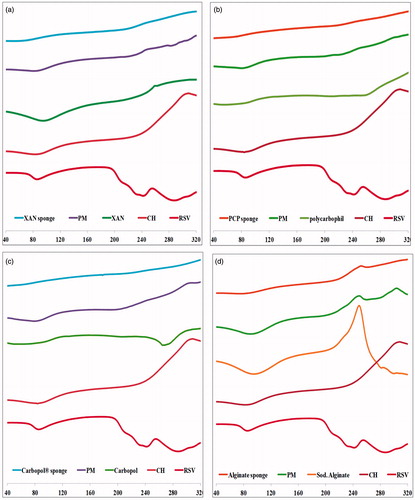
Those characteristic peaks were prominent in all chitosan/polyanionic polymer physical mixtures with decreased intensities probably due to dilution which proposes the absence of interaction between the polymers in their physical mixtures. On the other hand, the DSC thermograms of drug-loaded sponge formulae showed significant suppression of some of those characteristic peaks which might indicate changes in some physical properties during sponge preparation such as the crystalline state, degree of dehydration or melting point. Disappearance of drug peak could indicate the change of the crystalline drug into the amorphous state and/or possible inclusion in the developed polyelectrolyte complex during the DSC heating cycle (Mura et al., Citation2001).
Fourier transform infrared spectroscopy (FT-IR)
shows the IR spectra of all testes samples. The IR spectrum of chitosan showed the characteristic N–H, C–N and N–H stretching bands for amine groups between 3300–3500, 1080–1360 and 1600 cm−1, respectively. Similarly, the IR spectra of xanthan gum, polycarbophil, Carbopol® and sodium alginate had the carboxylic acid C = O and C–O stretch bands between 1700–1725 and 1210–1320 cm−1, respectively.
Figure 4. FT-IR of rosuvastatin, chitosan, anionic polymer, chitosan/anionic polymer physical mixtures and drug laoded sponges for (a) xanthan gum-based, (b) polycarbophil-based, (c) Carbopol®-based and (d) Na alginate-based formulae.
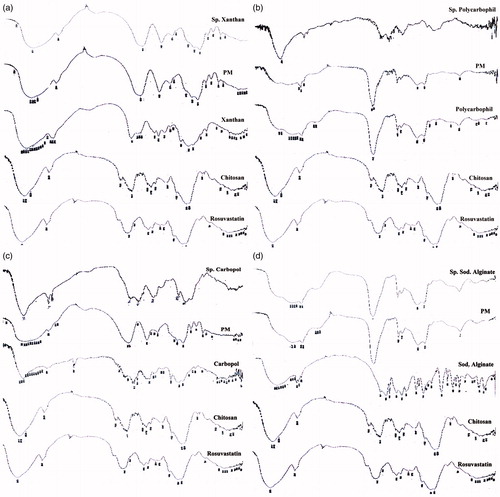
The IR spectra of the physical mixtures of chitosan with each of the anionic polymers appeared as a combination of the separate polymer spectra. The IR spectra of the prepared chitosan/polycarbophil, chitosan/Carbopol® and chitosan/sodium alginate sponges showed prominent shifting to the amide C = O stretching, N–H stretching and N–H bending absorptions between 1640–1690, 3100–3500 and 1550–1640 cm−1, respectively. This proves the formation of the PEC complexes. On the other hand, chitosan/xanthan gum spectrum failed to prove complete cross-linking between both polymers at the tested ratios and the used method of preparation. The bulky and rigid helical structure of xanthan with minimal negative charge as compared to the other polyanions could be the cause. The drug rather than the xanthan gum could be bound to the chitosan.
In vitro release profile and mathematical modeling
shows the in vitro release profiles of rosuvastatin from the prepared sponges while the calculated release parameters are listed in .
Figure 5. In vitro release profiles of rusovastatin from (a) xanthan gum-based, (b) polycarbophil-based, (c) Carbopol®-based and (d) Na alginate-based sponges prepared adapting a 41.31 full factorial design (n = 3, Error bars indicate SD).
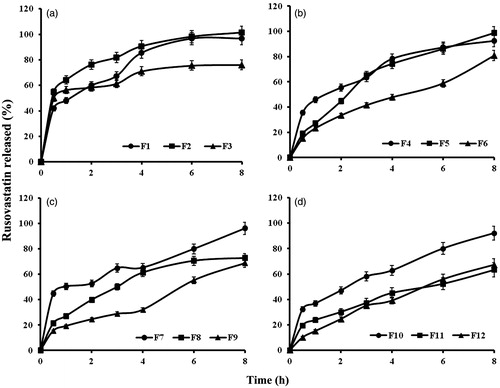
Statistical analysis proved that anionic polymer type and chitosan: polymer ratio, as well as, the interactions between them, exhibited significant effects on the release parameters at p ≤ 0.05 (). The polymers can be arranged in an ascending order according to their ability to sustain the drug release as xanthan gum < polycarbophil < Na alginate < Carbopol®. This could be explained by the differences in porosity as seen under microscope. Increasing the chitosan amount decreased both release rate and extent for all polymers except xanthan gum, where the most drug release sustainment was obtained at chitosan:polymer ratio of 1:1. This could be explained by the failure of PEC formation between xanthan gum and chitosan. The release was a function of viscosity of the cationic/anionic gel and consequently, the porosity and wettability of the lyophilized sponge. The drug rather than the xanthan gum could be bound to the chitosan. This observation comes in agreement with the previously discussed FT-IR results.
Figure 6. Effect of anionic polymer type and chitosan:polymer ratio on median dissolution time (MDT) and release efficiency (RE8h).
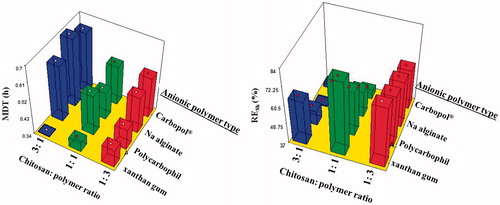
The results of the mathematical modeling of the release data are shown in . The release data of chitosan/xanthan gum sponges (F1–F3) fitted Higuchi equation with burst with high R2 adjusted values indicating diffusion-controlled drug release. The lowest burst release was recorded for xanthan gum-rich formula and the highest was for the formula with higher chitosan content. This could be attributed to the higher molecular weight and the slower wetting process of xanthan. Formula F2 prepared at chitosan/xanthan ratio of 1:1 showed intermediate burst release value. Further fitting to the Korsmeyer–Peppas model was not applicable due to the high initial burst (>60% drug was released in less than three time intervals).
For chitosan/polycarbophil sponge formulae, the release data best fitted the Higuchi equation without burst. The outputted (n) exponent values on fitting the initial 60% drug release values to the Korsmeyer–Peppas model were 0.650, 0.527 and 0.316 for chitosan:polycarbophil ratios of 1:3, 1:1 and 3:1, respectively. The R2 adjusted values obtained for the three ratios on fitting the data to the Baker–Lonsdale model were 0.8004, 0.8511 and 0.9794, respectively. The release followed anomalous diffusion for the first two chitosan/polycarbophil ratios and Fickian diffusion for the later one. The anomalous mode of drug release from F4 and F5 could be due to the presence of soluble uncomplexed polycarbophil. This suggests that the optimum ratio for the PEC formation between chitosan and polycarbophil is 3:1, where the release was controlled by diffusion through the insoluble PEC only. At lower ratios, there was a dual release mechanism, namely, diffusion through the complexed insoluble part plus erosion of the uncomplexed polycarbophil. Similarly, it was stated that non-stoichiometric polyelectrolyte complexes are usually soluble (Kramarenko et al., Citation2006; Hamman, Citation2010).
The release data of chitosan/Carbopol® sponges best fitted the Higuchi equation with burst release for formulae F7 and F8 (prepared at 1:3 and 1:1 chitosan/Carbopol® ratios) and Higuchi equation for formulae F9 (3:1 ratio based). The burst release (F0) value was markedly lower for the 1:1 ratio. The release data of 1:1 based sponge formula recorded (n) exponent value of 0.115 and a near unity R2 adjusted value for the Baker-Lonsdale model, which proposes this chitosan/Carbopol® ratio as optimum for PEC formation. On the other hand, the release mechanism from the 1:3 and 3:1 chitosan/Carbopol® ratio-based sponges was a combination of diffusion, as well as, erosion of uncomplexed Carbopol® and chitosan, respectively. The lower release rate and extent, as well as, the absence of initial burst release at higher chitosan ratio (F9) could be either attributed to rosuvastatin/chitosan interaction and/or to the lag time required for chitosan swelling.
Similarly, the optimum chitosan/Na alginate complexation ratio was 1:1. This formula (F11) fitted the Baker–Lonsdale model with R2 adjusted value of 0.9800 and had a Korsmeyer–Peppas exponent of 0.347. Higher chitosan amounts guaranteed lower burst and dual mode of release.
Release profile optimization
For further optimization, the formula with the most drug sustainment behavior for each cationic/anionic combination was selected. Imwitor® 900K and HPMC K100M were added as hydrophobicity imparting and release retarding additives, respectively. In some instances, rapid diffusion of drugs through the hydrophilic gel-like networks can be surpassed by incorporation of hydrophobic polymers such as cellulose derivatives or waxy retardant polymers or fats such as Compritol 888 ATO, or Imwitor® 900K, respectively, into the hydrophilic matrix to enhance their sustained-release properties (Reddy et al., Citation2003; Gu et al., Citation2004). Imwitor® 900K, a Glyceryl monostearate, has been used as a waxy retardant polymer to form sustained-release delivery systems including implantable, biodegradable and controlled-release matrices (Peri et al., Citation1994).
The results as presented in and confirmed that Imwitor® 900K was more efficient in prolonging the drug release from the sponges. Initial burst release markedly decreased and the release data best fitted the Higuchi equation without burst. All formulae scored Korsmeyer–Peppas exponent values < 0.5 and had near linearity fitting to the Baker–Lonsdale model proving Fickian diffusion mechanism, outputted values are not shown.
Table 3. Composition and evaluation of the optimized sponge formulae.
Statistical analysis of the results were optimized using Design Expert 7.0.0 software with the targeted release profile should have the highest MDT value and the lowest RE8h. Formula F16 prepared at 3:1 chitosan to Carbopol® ratio in presence of 2% Imwitor® had the highest desirability value (0.869) and was proposed for further investigation.
Sterilization
After the optimized formula (F16) was subjected to sterilization by gamma radiation, assessment of the sterilized formula revealed that it retained its appearance and drug content. The release profiles of the sterilized formulation was compared to the fresh ones and the calculated similarity factor (f2) was 56 proving insignificant effect for the radiation on the release profile and kinetics of rosuvastatin release from the sponge.
In vivo evaluation of bone regeneration activity of rosuvastatin-loaded sponge
Fourteen rats completed the study while two were dead. For the treated right femora, the area of healing showed osteoblasts as a remodeling bone formation () associated with arrangement of woven bony structure (). Those findings prove advanced stage of healing. On the other hand, the histopathological examination of the control left femora showed empty lacunae and collagen fibers, as well as, osteoclasts and fibroblasts at the fractured area () indicating early stage of healing. Occupying the initiated bone defect with newly formed bone tissue is due to the previously reported osteogenic effect of locally applied statins (Wong & Rabie Citation2003; Ozeç et al., Citation2007). Statins were proved to augment bone formation and fracture healing through two mechanisms; either anabolic effects on bone metabolism, likely through activation of bone morphogenic protein (BMP-2), a growth factor targeting bone formation, and TGF-b1 (Mundy et al., Citation1999; Nyan et al., Citation2010). The other mechanism is anti-resorptive mechanism through suppression of osteoclast activity associated with decreased osteoblast apoptosis in the healing sites, thus controlling the bone remodeling process (Hughes et al., Citation2007; Luisetto & Camozzi, Citation2009; Moriyama et al., Citation2010).
Figure 8. Photographs of treated rat femur treated with formula F16 showing osteoblasts (→) and remodeling (m) phase of bone healing at magnifications of (a) 16×, (b) 40× and (c) 64×.
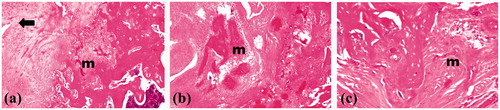
Figure 9. Photographs of control rat femur showing empty lacunae (→), collagen fibers (c), osteoclasts (cl) and fibroblasts (f) in area of healing at magnifications of (a) 16×, (b) 40×, (c) 64× and (d) 160×.

Monjo et al., (Citation2010b) confirmed similar results for rosuvastatin. They claimed that when rosuvastatin was administered locally in bone, it may have a potential effect in stimulating bone formation; as they observed that the cortical architecture of bone defects analyzed by micro-computed tomography showed a trend towards higher bone volume in the rosuvastatin site compared with control group.
In addition, chitosan might also contribute to this bone and tissue healing as Venkatesan & Kim (Citation2010) stated that degradation of chitosan by tissue enzymes could be effective in regenerating the tissue in wounds.
Minimal traces of the sponge material were found in the implanted area after 4 weeks indicating high biodegradability of the used polymers. The absence of any material-induced inflammation proves compatibility of the degradation products of those polymers.
Biodegradability and biocompatibility of the used polymers were clearly seen in the minimal traces of the sponge materials found in the implanted area after 4 weeks and the absence of any inflammation response, respectively (Shi & Huang, Citation2009; Tanigo et al., Citation2010).
Conclusion
Biodegradable sponges were successfully prepared for local rosuvastatin delivery. They may represent an interesting approach to bring the drug into immediate contact with the fractured bone. Combining RSV with chitosan in the sponge formulations is assumed to provide significantly enhanced wound healing and regeneration properties. Rosuvastatin has proved potential effects in stimulating bone formation, and also, chitosan might augment the drug osteoinductive activity inducing promoted bone regeneration.
Declaration of interest
The authors report no conflict of interest including any financial, personal or other relationships with other people or organizations that could inappropriately influence, or be perceived to influence, the work in this article.
Acknowledgements
Authors would like to thank Dr Abubakr Zein, MD, Department of Orthopedic Surgery, Faculty of medicine, Cairo University for help with the surgical procedure and Dr Adel Bakeer Kholoussy, Professor of Pathology, Cairo University for conducting the histopathological study.
References
- Ayensu I, Mitchell JC, Boateng JS. (2012). Development and physico-mechanical characterisation of lyophilised chitosan wafers as potential protein drug delivery systems via the buccal mucosa. Colloids Surf B Biointerfaces 91:258–65.
- Ayukawa Y, Yasukawa E, Moriyama Y, et al. (2009). Local application of statin promotes bone repair through the suppression of osteoclasts and the enhancement of osteoblasts at bone-healing sites in rats. Oral Surg Oral Med Oral Pathol Oral Radiol Endod 107:336–42.
- Baker RW, Lonsdale HS. (1974). Controlled release of biologically active agents. New York: Plenum Press.
- Bancroft JD, Gamble M. (2008). Theory and practice of histological techniques. 6th ed. EdinburghChurchill Livingstone: Elsevier Health Sciences. p. 332--62.
- British Pharmacopoeial Commission. (2007). B.P. (British Pharmacopoeia). London: HMSO.
- Derringer G, Suich R. (1980). Simultaneous optimization of several response variables. J Quality Technol 12:214–19.
- Gu X, Fediuk DJ, Simons FER, Simons KJ. (2004). Evaluation and comparison of five matrix excipients for the controlled release of acrivastine and pseudoephedrine. Drug Dev Ind Pharm 30:1009–17.
- Gutierrez GE, Lalka D, Garrett IR, et al. (2006). Transdermal application of lovastatin to rats causes profound increases in bone formation and plasma concentrations. Osteoporos Int 17:1033–42.
- Hamman JH. (2010). Chitosan based polyelectrolyte complexes as potential carrier materials in drug delivery systems. Marine Drugs 8:1305–22.
- Higuchi T. (1961). Rate of release of medicaments from ointment bases containing drugs in suspension. J Pharm Sci 50:874–5.
- Higuchi T. (1963). Mechanism of sustained-action medication. Theoretical analysis of rate of release of solid drugs dispersed in solid matrices. J Pharm Sci 52:1145–9.
- Hirabayashi H, Fujisaki J. (2003). Bone-specific drug delivery systems: approaches via chemical modification of bone-seeking agents. Clin Pharmacokinet 42:1319–30.
- Hughes A, Rogers MJ, Idris AI, Crockett JC. (2007). A comparison between the effects of hydrophobic and hydrophilic statins on osteoclast function in vitro and ovariectomy-induced bone loss in vivo. Calcif Tissue Int 81:403–13.
- Korsmeyer RW, Gurny R, Doelker E, et al. (1983). Mechanisms of solute release from porous hydrophilic polymers. Int J Pharm 15:25–35.
- Kramarenko EY, Khokhlov AR, Reineker P. (2006). Stoichiometric polyelectrolyte complexes of ionic block copolymers and oppositely charged polyions. J Chem Phys 125:194. DOI: 10.1063/1.2387173.
- Lindner WD, Lippold BC. (1995). Drug release from hydrocolloid embeddings with high or low susceptibility to hydrodynamic stress. Pharm Res 12:1781–5.
- Luisetto G, Camozzi V. (2009). Statins, fracture risk, and bone remodeling. J Endocrinol Invest 32:32–7
- Maeda T, Matsunuma A, Kawane T, Horiuchi N. (2001). Simvastatin promotes osteoblast differentiation and mineralization in MC3T3-E1 cells. Biochem Biophys Res Commun 280:874–7.
- McTaggart F, Buckett L, Davidson R, et al. (2001). Preclinical and clinical pharmacology of rosuvastatin, a new 3-hydroxy- 3-methylglutaryl coenzyme A reductase inhibitor. Am J Cardiol 87:28–32.
- Miyamoto K, Takahashi-Nishioka T, Yokogawa K, et al. (2008). Targeted drug delivery to bone: pharmacokinetic and pharmacological properties of acidic oligopeptide-tagged drugs. Curr Drug Discov Technol 5:39–48.
- Modi A, Tayade P. (2006). Enhancement of dissolution profile by solid dispersion (kneading) technique. AAPS PharmSciTech 7:E87--E92.
- Moffat AC, Osselton MD, Widdop B. (2011). Clarke’s analysis of drugs and poisons. 4th ed. London: Pharmaceutical Press. p. 2034--5.
- Monjo M, Rubert M, Ellingsen JE, Lyngstadaas SP. (2010a). Rosuvastatin promotes osteoblast differentiation and regulates SLCO1A1 transporter gene expression in MC3T3-E1 cells. Cell Physiol Biochem 26:647–56.
- Monjo M, Rubert M, Wohlfahrt JC, et al. (2010b). In vivo performance of absorbable collagen sponges with rosuvastatin in critical-size cortical bone defects. Acta Biomater 6:1405–12.
- Moriyama Y, Ayukawa Y, Ogino Y, et al. (2008). Topical application of statin affects bone healing around implants. Clin Oral Implants Res 19:600–5.
- Moriyama Y, Ayukawa Y, Ogino Y, et al. (2010). Local application of fluvastatin improves peri-implant bone quantity and mechanical properties: a rodent study. Acta Biomater 6:1610–18.
- Mundy G, Garrett R, Harris S, et al. (1999). Stimulation of bone formation in vitro and in rodents by statins. Science 286:1946–9.
- Mura P, Faucci MT, Bettinetti GP. (2001). The influence of polyvinylpyrrolidone on naproxen complexation with hydroxypropyl-β-cyclodextrin. Eur J Pharm Sci 13:187–94.
- Nyan M, Miyahara T, Noritake K, et al. (2010). Molecular and tissue responses in the healing of rat calvarial defects after local application of simvastatin combined with alpha tricalcium phosphate. J Biomed Mater Res B 93:65–73.
- Nyan M, Sato D, Oda M, et al. (2007). Bone formation with the combination of simvastatin and calcium sulfate in critical-sized rat calvarial defect. J Pharmacol Sci 104:384–6.
- Orlu M, Cevher E, Araman A. (2006). Design and evaluation of colon specific drug delivery system containing flurbiprofen microsponges. Int J Pharm 318:103–17.
- Oxlund H, Andreassen TT. (2000). Simvastatin given orally to adult rats increases the compressive strength of vertebral bodies. J Bone Mineral Res 15:s549.
- Oxlund H, Andreassen TT. (2004). Simvastatin treatment partially prevents ovariectomy-induced bone loss while increasing cortical bone formation. Bone 34:609–18.
- Ozeç I, Kiliç E, Gümüş C, Göze F. (2007). Effect of local simvastatin application on mandibular defects. J Craniofac Surg 18:546–50.
- Pavli M, Baumgartner S, Kos P, Kogej K. (2011). Doxazosin-carrageenan interactions: a novel approach for studying drug-polymer interactions and relation to controlled drug release. Int J Pharm 421:110–19.
- Peri D, Bogdansky S, Allababidi S, Shah JC. (1994). Development of an implantable, biodegradable, controlled drug delivery system for local antibiotic therapy. Drug Dev Ind Pharm 20:1341–52.
- Reddy KR, Mutalik S, Reddy S. (2003). Once-daily sustained-release matrix tablets of nicorandil: formulation and in vitro evaluation. AAPS PharmSciTech 4:E61.
- Shah VP, Tsong Y, Sathe P, Liu JP. (1998). In vitro dissolution profile comparison—statistics and analysis of the similarity factor, f2. Pharma Res 15:889–96.
- Shi Y, Huang G. (2009). Recent developments of biodegradable and biocompatible materials based micro/nanoparticles for delivering macromolecular therapeutics. Crit Rev Ther Drug Carrier Syst 26:29–84.
- Stojadinovic O, Lebrun E, Pastar I, et al. (2010). Statins as potential therapeutic agents for healing disorders. Expert Rev Dermatol 5:689–98.
- Sugiyama M, Kodama T, Konishi K, et al. (2000). Compactin and simvastatin, but not pravastatin, induce bone morphogenetic protein-2 in human osteosarcoma cells. Biochem Biophys Res Commun 271:688–92.
- Tadros MI, Fahmy RH. (2014). Controlled-release triple anti-inflammatory therapy based on novel gastroretentive sponges: characterization and magnetic resonance imaging in healthy volunteers. Int J Pharm 472:27–39.
- Tanigo T, Takaoka R, Tabata Y. (2010). Sustained release of water-insoluble simvastatin from biodegradable hydrogel augments bone regeneration. J Control Release 143:201–6.
- Venkatesan J, Kim SK. (2010). Chitosan composites for bone tissue engineering – an overview. Marine Drugs 8:2252–66.
- Wilkie D, Bowman B, Lyga A, et al. (2000). Cerivastatin increases cortical bone formation in OVX rats. J Bone Mineral Res 15:S549.
- Wong RWK, Rabie ABM. (2003). Statin collagen grafts used to repair defects in the parietal bone of rabbits. Br J Oral Maxillofac Surg 41:244–8.