Abstract
In this study, protease-producing capacity of Bacillus pumilus D3, isolated from hydrocarbon contaminated soil, was evaluated and optimized. Optimum growing conditions for B. pumilus D3 in terms of protease production were determined as 1% optimum inoculum size, 35 °C temperature, 11 pH and 48 h incubation time, respectively. Stability studies indicated that the mentioned protease was stable within the pH range of 7–10.5 and between 30 °C and 40 °C temperatures. Surprisingly, the activity of the enzyme increased in the presence of SDS with concentration up to 5 mM. The protease was concentrated 1.6-fold with ammonium sulfate precipitation and dialysis. At least six protein bands were obtained from dialysate by electrophoresis. Four clear protein bands with caseinolytic activity were detected by zymography. Dialysate was further purified by anion-exchange chromatography and the caseinolytic active fraction showed a single band between 29 and 36 kDa of reducing conditions.
Introduction
Proteases are hydrolytic enzymes catalyzing the breakdown of peptide bonds so proteins are degraded into peptides and amino acidsCitation1,Citation2. All living organisms require proteases which play a significant role in various vital physiological processes such as food digestion, blood clotting, transcription control, growth factor secretion, embryogenesis, wound healing, cell migration, cell signaling, regeneration, molting, metamorphosis, defense mechanisms and immune responsesCitation3. Proteases are one of the most important enzymes for industries such as detergent, textile, pharmaceuticals. Furthermore, they also play a significant role for manufacturing of protein hydrolysates, flavor and color in cookies, meat tenderization process and silver recovery from X-ray filmsCitation4–6. Enzymes used in industrial applications can be obtained from plants, animals and microorganisms. Microorganisms serve as a preferred protease source since they grow rapidly and they require limited space for cultivation. Additionally, recent developments in the fields of genetic engineering and protein chemistry provide a significant option to analyze enzyme structure and function; thereby, research on new enzymes with novel characteristics has an advantage for various industrial applications. Microbial proteases represent ∼40% of all the industrial enzyme sales throughout the worldCitation7.
Microbial proteases consist of acidic, neutral and alkaline enzymes; furthermore, depending on the characteristics of the active site group, metallo, aspartic, cysteine and serine types may occurCitation7,Citation8. Alkaline proteases, physiologically and commercially important catalysts, are active in a neutral to alkaline pH range, holding >50% of the total enzyme marketCitation9. Moreover, alkaline proteases are used in dehairing, peptide synthesis in organic solvents, fortification of fruit juice, manufacturing of protein-rich therapeutic dietsCitation10, preparation of slow-release dosage forms of therapeutic agentsCitation11, bioprocessing of used X-ray films for silver recoveryCitation12.
Most microbial proteases extracted from Bacillus species are one of the most significant industrial enzymes, especially as laundry detergent additivesCitation13. These stable enzymes are active under various conditions. The first alkaline protease extracted from Bacillus licheniformis was commercialized for detergent industry in the 1960s. So far, more alkaline proteases have been exploited from Bacillus species which have broad stability and substrate specificityCitation14,Citation15.
The aim of this study is to screen all isolates from hydrocarbon-contaminated soil and determine optimal conditions and stability in terms of the production of protease. Furthermore, purification of this mentioned enzyme was targeted.
Materials and methods
Isolation of microorganisms
The soil samples were collected from hydrocarbon-polluted soil in the province of Eskişehir, Turkey. Soil sample (10 g) was suspended in 90 ml of distilled water and a set of serial dilution was made. Soil suspension was plated onto minimal salt agar medium and incubated at 35 °C for 48 h. Purified isolates were investigated in terms of the abilities of protease production using 10% skim milk. Clearance zones in the surrounding isolated colonies indicated proteolytic activities. Three bacterial isolates with a higher zone of clearance were selected and determined spectrophotometrically by following digestion of casein.
Protease assay
Proteolytic activity was determined by the Anson methodCitation16. The reaction mixture containing 2.5 ml of 0.6% casein solution in 50 mM glycine–NaOH–NaCl buffer having pH 10.5 and 0.5 ml of crude enzyme were incubated at 30 °C for 20 min and the reaction was then ceased by the addition of 2.5 ml of trichloroacetic acid. The mixture was then incubated at room temperature for 30 min. Following incubation, the reaction mixture was centrifuged at 9000 rpm for 5 min. Supernatant (0.5 ml) was mixed with 0.5 M sodium carbonate and 0.5 ml of the Folin–Ciocalteu reagent and incubated at room temperature for 30 min. The amount of tyrosine in the solution was measured by reading the absorbance at 660 nm. Protein concentrations were measured by the Bradford methodCitation17. One unit of enzyme activity is defined as the amount of the enzyme resulting from the release of 1 µg of tyrosine per minute at 30 °C.
Enzyme production
Among the isolates, the isolates A6, C3 and D3 showing higher clearance zone diameter were chosen for enzyme production. The basal culture medium for the protease production included (g/l) peptone, 5; glucose, 10; NaCl, 0.5; CaCl2.2H2O, 0.1; K2HPO4, 0.3; KH2PO4, 0.4; MgSO4. 7H2O, 0.1; and yeast extract, 5. The pH was adjusted to 7.5 and the medium was inoculated with 5% (v/v) of seed culture and incubated at 35 °C for 18 h. The cultures were centrifuged at 9000 rpm for 10 min in an aim to recover the cell-free supernatant, and protease activities were checked in the culture supernatant. The bacterium isolate with maximum protease activity was selected for further experimental studies.
Identification of protease-producing organisms
Genomic DNA of protease-producing isolate was extracted by boiling 200 µl of liquid culture for 15 min at 95 °C and centrifuging for 5 min at 13 000 rpm. This obtained supernatant was used for a template for PCR reactions. Reaction mixture included 10× Taq buffer, 2.5 mM of MgCl2, 0.2 mM of each oligonucleotide primer, 200 μM of each deoxyribonucleotide triphosphate (Invitrogen, Carlsbad, CA), 1 U of Taq DNA Polymerase (Invitrogen) and 1 µl of template DNA in a total volume of 25 μl. The sequence of the forward primer was 27f (5′-AGAGTTTGATCATGGCTCAG-3′), the reverse primer was 1387r (5′-GGG CGG WGT GTA CAA GGC-3′)Citation18. PCR condition was a cycle of 94 °C for 3 min as initial denaturation, 30 cycles of 94 °C for 15 s, 55 °C for 30 s and 72 °C for 2 min; and 10 min at 72 °C as extension step. The PCR product was purified using SureClean (Bioline, London, UK). The purified PCR product was sequenced using a genetic analyser (Beckman Coulter CQ8000, Brea, CA). The primer sets used were 27f and 1387r primer set. Obtained sequences were searched against nucleic acid database using blast algorithms (http://www.ncbi.nlm.nih.gov/blast) to identify this geneCitation19.
Determination of optimum growth conditions
Effect of pH on enzyme production was determined by adjusting pH of basal medium from 5 to 12, using Na2HPO4–NaH2PO4 buffer solution (pH 5–8) and NaHCO3–NaOH buffer solution (pH 9–12).
To determine the effect of inoculum size, culture was incubated in the medium with inoculum ranging from 1% to 10% (v/v). Reaction mixtures were incubated at 35 °C by shaking at 150 rpm for 18 h, and protease activity was measured according to the protease assay.
To determine the most effective carbon source for maximum protease production, glucose in the basal medium was substituted by lactose, sucrose, fructose, maltose, cellulose, glycerol, molasses and starch. The effect of different nitrogen sources on protease production was determined by supplementing basal medium with organic nitrogen sources such as malt extract, tyrosine, yeast extract, skim milk, gelatine, glycine and inorganic nitrogen sources such as ammonium nitrate and ammonium chloride. The medium was incubated at 35 °C by shaking at 150 rpm for 18 h and cell-free supernatant was analyzed for protease activity. The obtained carbon and nitrogen sources were optimized within the range of 0.25–5%.
The optimum temperature for better protease production was determined by incubating the culture at different temperatures ranging between 30 °C and 55 °C at 150 rpm for a period of 18 h.
In order to determine the optimum incubation time for maximum enzyme production, 100 ml of basal medium inoculated with 1% (v/v) of the seed culture was incubated at optimum temperature and at 150 rpm for 6–96 h.
Characterization of protease
Optimum reaction temperature and pH
The optimum pH for protease activity was determined by using casein as a substrate dissolved in a different pH value of NaOH–glycine–NaCl buffer (pH 8–12.5). To determine the pH profile, casein was dissolved in 50 mM of glycine–NaOH buffer at pH values ranging from 8.0 to 12.0. To determine the effect of temperature on protease activity, varying temperatures between 30 °C and 90 °C were investigated.
Thermal and pH stability of protease
Thermal stability was tested by pre-incubating the culture supernatant at various temperatures ranging from 25 °C to 70 °C for 1 h before measurement of enzyme activity. Protease activities of pre-incubated culture supernatants were assayed as described earlier. The pH stability of protease was determined for pH values ranging from 6 to 13 for 1 h at 30 °C. The residual activities of the protease were measured. The maximum activity of crude enzyme was expressed as 100%.
Purification procedures
The cell-free supernatant was obtained as described in the Section “Enzyme production” and solid ammonium sulfate was slowly added to obtain 75% saturation at 4 °C under constant magnetic stirring for overnight. The precipitated proteins were pelleted by centrifugation at 10 000 rpm, 4 °C for 30 min; then re-suspended in buffer solution containing 50 mM NaOH–glycine–NaCl at pH 10.5, and dialyzed against the same buffer for 24 h in a dialysis tubing (Thermo Scientific SnakeSkin®, Pleated Dialysis Tubing, 3500 MWCO). The dialysate was purified by anion-exchange chromatography on DEAE-cellulose at room temperature in a 30 cm × 1 cm column which was previously equilibrated with the same buffer. The flow rate was held at 20 ml/h.
Effect of heavy metals and denaturants on protease activity
To examine the effect of heavy metals such as FeCl3, CuCl2, CdCl2, CuSO4, NiCl2 and HgCl2, the protease was incubated for 20 min separately together with these metals at a final concentration of 5 mM. The remaining activity was measured by the previously described method. The purified protease was incubated with SDS at a final concentration of 0.5, 1.0, 5.0 and 10.0 mM, and with urea at a final concentration of 0.5, 1.0 and 5.0 mM to determine the enzyme stability.
SDS–polyacrylamide gel electrophoresis and zymogram assays
To determine the protein profile of the dialysate and purified protease, SDS–polyacrylamide gel electrophoresis (PAGE) was performed according to the method of LaemmliCitation20. The protein concentration was measured by the method of Bradford for electrophoresis experiment using bovine serum albumin (fraction V) as standard and 100 µg of samples were submitted to a 5% stacking and 12% resolving the gel electrophoresis under reducing conditions. The electrophoresis was performed at room temperature, at 80 V for 15 min and then 120 V, approximately for 2 h. After electrophoresis, the gel slabs were stained with Coomassie brilliant blue R-250 in 25% isopropanol and 10% acetic acid and subsequently were destained in a 10% methanol and 5% acetic acid solution. Wide range molecular weight standards were used as markers.
The protease activity of dialysate was also analyzed by SDS–PAGE–zymogram assays by 12% acrylamide containing 1 0 mg/ml of casein as a substrate in the resolving gelCitation21. The dialysate was applied onto the gel under non-reducing conditions without heating and electrophoresis was performed for 3 h at 4 °C and at a constant current voltage of 100 V. After electrophoresis, the gel was washed in 2.5% Triton X-100 for 30 min to remove SDS and were incubated overnight at 37 °C in the buffer as described in “Purification procedures” to determine proteolytic activity. Following the incubation procedure, the gels were stained and destained as described earlier. The clear zones of substrate lysis against a blue background stain indicated the presence of casein-degrading activity.
Inhibition of the proteolytic activity was determined using the same buffer at pH 10.5 contained in the presence of two different types of proteinase inhibitor such as 10 mM ethylene diamine tetra acetic acid (EDTA) containing 5 mM CaCl2 and 10 mM phenyl methyl sulfonyl fluoride (PMSF) for overnight incubation at 37 °CCitation22. As positive control, the gel was developed in the absence of any protease inhibitor under the same condition.
Results and discussion
Screening of proteolytic activity
Microorganisms with higher tolerance of carbon make use of hydrocarbon pollutants as carbon source and increase of some microorganism populations have been observed in such polluted soilsCitation23. Besides, according to the study of Margesin et al.Citation24, hydrocarbons caused increase of microbial protease activity. The rationale in selecting the type of polluted soil used in this work was also richness of hydrocarbons in soil, so it was considered that hydrocarbons induced protease activity.
A total of 69 isolates were obtained among hydrocarbon-polluted soil samples as per their morphologically different characteristics. They were screened for protease production based on the zone of clearance on agar plates containing skim milk. Among the 69 isolates, 28 of them had the ability to produce protease. Three isolates with a prominent zone of clearance were selected for further spectrophotometric protease assay. When D3 isolate was able to grow better in basal medium, it produced the best protease (7.65 U/ml/min) in the incubation period and was finally selected for further studies.
Identification of the strain
The partial 16 S rRNA gene sequence of protease producer strain was determined. The sequence was aligned and compared with the bacterial sequences available in the GenBank database. According to BLAST analysis, the highest similarity level (100%) detected was from the 16 S rDNA sequence of the strain of B. pumilus S_T_TSA_70 deposited in the GenBank (accession number JX860616).
Determination of optimum growth conditions
To determine the effect of pH on protease production, the B. pumilus D3 isolate was allowed to grow in various basal culture media with pH ranging between 5 and 12. The highest levels of protease production was achieved in the culture grown at pH 11, thereby indicating the isolate can be classified as alkaliphilic microorganism (data shown in ). This result is in good agreement with the studies by some researchers, who reported maximum protease activity at pH 11 with B. pumilusCitation25–27. Similarly, Tekin et al.Citation28 also reported that B. cohnii produced maximum protease at pH 11. The production of an alkaline protease at 11.5 from Bacillus sp. was reported by some researchersCitation29–31. However, Padmapriya et al.Citation32 found that Bacillus sp. showed maximum protease activity at pH 8.
Figure 1. Effect of pH on protease production (experimental conditions: incubation time 18 h, incubation temperature 35 °C, inoculum size 5%, glucose as carbon source, yeast extract as nitrogen source).
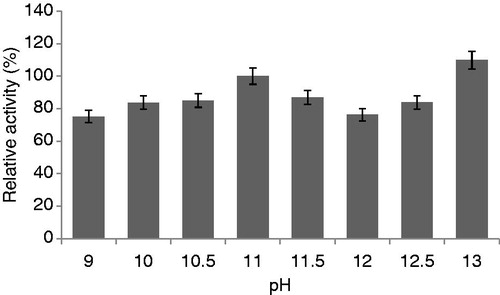
Various amounts of inoculum ranging from 1% to 10% (v/v) were evaluated for optimum production of alkaline protease and maximum activity was found at 1% of inoculum size (). There was a decrease in alkaline protease production with a further increase in inoculum size. Higher inoculum concentrations may cause decrease of nutrients or autolysis of the enzymes and resulted in less growth and alkaline protease production. This result is in line with previous studies by Mrudula and ShyamCitation33 for yielding maximum protease production by immobilized B. megaterium MTCC 2444. However, optimum protease production with 10% of inoculum size was reported by Nadeem et al.Citation34.
Figure 2. Effect of inoculum size on protease production (experimental conditions: incubation time 18 h, incubation temperature 35 °C, glucose as carbon source, yeast extract as nitrogen source, pH 11).
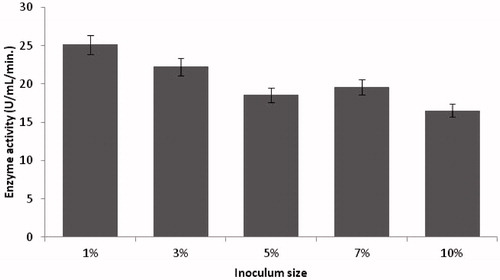
Various carbon sources such as lactose, sucrose, fructose, maltose, cellulose, glycerol, molasses and starch were used to replace glucose which is the original carbon source growth medium. As shown in , the results showed that lactose yielded the highest protease production. Maltose and fructose also showed high protease production. The effect of nitrogen source was studied in the growth medium, where yeast extract was replaced by malt extract, tyrosine, yeast extract, skim milk, gelatine, glycine, ammonium nitrate and ammonium chloride. According to , among the various nitrogen sources examined, yeast extract was found to be the best nitrogen source for protease production. Ammonium chloride and ammonium sulfate suppressed alkaline protease production significantly.
Figure 3. Effect of carbon sources on protease production (experimental conditions: incubation time 18 h, incubation temperature 35 °C, inoculum size 1%, yeast extract as nitrogen source, pH 11.0).
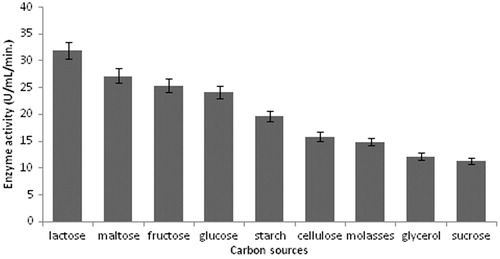
Figure 4. Effect of nitrogen sources on protease production (incubation time 18 h, incubation temperature 35 °C, inoculum size 1%, lactose as carbon source, pH 11.0).
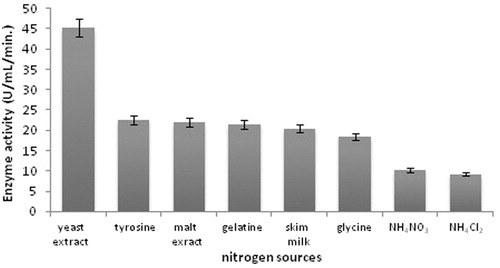
The temperature profile for protease production showed maximum activity at 40 °C (). These results comply with the literature reports where 40 °C is the best temperature for alkaline protease productionCitation33 and by newly isolated Bacillus sppCitation35. In addition, Singh et al.Citation36 reported that Bacillus sp. SSR-1 achieved maximum protease activity at 40 °C.
Figure 5. Effect of temperature on protease production (experimental conditions: incubation time 18 h, inoculum size 1%, lactose as carbon source, yeast extract as nitrogen source, pH 11.0).
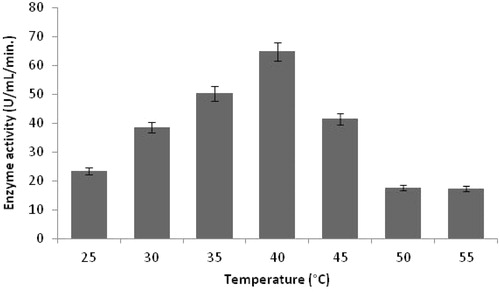
Effect of incubation time on protease production was investigated within the range of 12–96 h. As shown in , 48 h was selected as the optimal period for production of this enzyme. Similar results were reported in the literatureCitation32.
Chromatographic separation and characterization of alkaline protease
Protease purification
For obtaining alkaline protease from the cell-free supernatant, concentrated ammonium sulfate precipitation (75%) and dialysis were used (). The dialysate, which has ∼21% of the enzyme yield, was obtained with 1.6 purification fold. The specific activity of the dialysate was 574.49 U/mg of protein. Then, the column chromatography was performed as the first step purification. The fractions were collected and those possessing protease activity were pooled. The purification of the crude enzyme through the column chromatography resulted in 5.05 increase in purity along with 18% recovery of protease from the cell-free supernatant which is similar to other reports in the literature. Mazar et al.Citation37 reported purification steps of protease from the isolate of B. subtilis with 4.8-fold with a yield of 39.7%. In the study on B. pumilus MK6-5 protease, Kumar employed multi-steps for purification as the protease could not bind to the first anion-exchange chromatography. Further chromatographic steps in purification process showed a 20-fold increase in the protease specific activity and plus a 13-fold increase in specific activityCitation31. While several steps of purification methodology were often used throughout the purification process, here we obtained the protease by using anion-exchange chromatography with one SDS–PAGE band as a proof of purity as discussed in the later section.
Table 1. Summary of the purification of an alkaline protease.
Characterization of alkaline protease
Optimum reaction temperature and pH
The optimum temperature for protease activity was tested by changing the reaction temperature at pH 10.5. The optimum temperature was observed as 55 °C, indicating the enzyme to thermoprotease. The results indicated that the protease activity was >90% at 60 °C and 65 °C. Our results were supported by those of Ravishankar et al.Citation38 who found maximum protease activity at 55 °C by B. subtilus AKR53. In addition, similar results showed that the optimum reaction temperature of protease enzyme by B. pumilus was 60 °CCitation25 and 50 °CCitation39.
The optimum pH was found to be 11, indicating that this enzyme might be the alkaline proteaseCitation40,Citation41. An optimum pH of 11 of alkaline protease was observed in the protease produced by Bacillus spCitation41,Citation42.
Temperature and pH stability
The crude protease solution was incubated at various temperatures for 1 h to determine the thermal stability. The enzyme showed good thermostability <40 °C (). These results showed that the protease activity retains >85%, 70% and 57% of its initial activity after incubation 1 h at 45 °C, 50 °C and 55 °C, respectively. Moreover, in this study, the proteases obtained from the cultivation of B. pumilus D3 were stable at 45 °C and 50 °C for 30 min.
Figure 7. Effects of temperature on protease activity (experimental conditions: reaction pH 10.5, incubation time 20 min, lactose as carbon source, yeast extract as nitrogen source, pH 10.5).
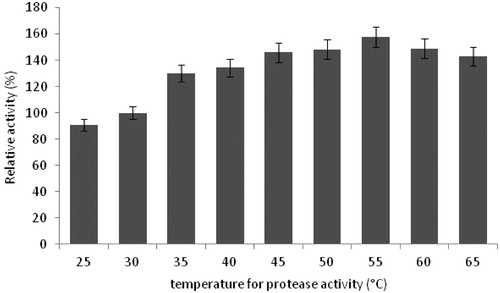
When the effect of pH on the protease activity was investigated, the optimum pH of enzyme was determined as 11.0 (). Nearly 100% of enzyme activity was still detectable at pH 13.0 after 20 min at 30 °C. This might be the alkaline protease. The protease activity had relative activity ∼80% in NaOH–glycine–NaCl buffer pH ranging from 6 to 10.5Citation33. This was in agreement with a research by ChomsriCitation43 who reported a protease activity which was stable within the pH range from 7 to 10.
Effect of heavy metals and denaturants
The effect of various heavy metal ions at a final concentration of 5 mM on the enzyme activity was tested and results were presented in . All metal ions had an inhibitory effect on the enzyme activity. The maximum inhibitory effect was observed when FeCl2, CdCl2 and HgCl2 were used.
Table 2. Effects of heavy metals on protease activity (experimental conditions: reaction temperature 30 °C, reaction pH 10.5, incubation time 20 min).
Protease from B. pumilus D3 isolate was highly stable in the presence of denaturants. Urea (0.5 mM) had no effect on protease activity. More than 80% of activity was retained after pre-incubation for 30 min in the presence of 1.0 and 5.0 (v/v) mM urea. In general, bacterial proteases are unstable against SDS. However, it was noteworthy that the addition of 0.5 and 1.0 mM SDS increase the protease activity. The addition of 0.5 mM of SDS increased the enzyme activity by 35%, 1.0 mM of SDS increased the activity by 67% and 5.0 mM of SDS increased the activity at the rate of 93% (). SDS resistance may be an important characteristic for industrial applications such as protease additive detergent and related products.
Figure 9. Effect of SDS concentration on relative activity of protease (the activity in the absence of SDS, referred to 100% relative activity).
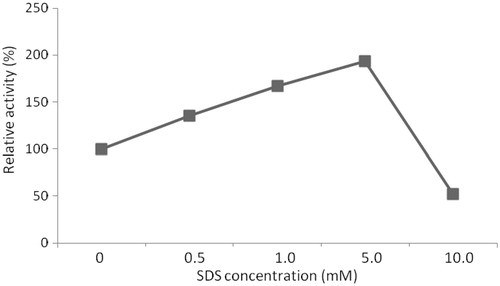
The alkaline protease extracted from Bacillus SB5 showed stability in the presence of 10% of H2O2 and 1% of SDS; furthermore, this enzyme had an optimum activity at pH 10 and 60 °C to 70 °CCitation13. Oberoi et al.Citation44 also produced an alkaline, SDS-stable protease from Bacillus sp. RGR-14 suggesting an ideal detergent additive for detergent formulations. The alkaline protease from B. clasuii I-52 is important owing to its ability to function in a broad pH and temperature ranges, its tolerance and stability in the presence of SDS and oxidantsCitation45.
SDS–PAGE and zymography
Electrophoretic profile of dialysate was obtained as shown in , lane 1. At least six different protein bands (indicated with arrow) appeared; one band between 116 and 205 kDa, one very strong band between 84 and 97 kDa, one band between 45 and 55 kDa, one wide but weak band between 29 and 36 kDa, one weak band between 24 and 29 kDa and one band <6.5 kDa. Several fractions from column chromatography were eluted and tested for caseinolytic activities. The active fractions were pooled and loaded in the same conditions to SDS–PAGE gel for confirmation of final purity. The fraction was observed as a one wide and weak band between 29 and 36 kDa (see lane 2 of ). The molecular weight of the purified protease was higher as compared with other bacterial alkaline protease where it normally ranged from 15 to 30 kDaCitation46. However, bacterial alkaline proteases with molecular mass between 32 and 35 kDa has also been reported earlierCitation47.
Figure 10. (A) Electrophoretic profiles of dialysate (lane 1) and purified enzyme (lane 2) by 12% SDS–PAGE under reducing conditions. Molecular weight markers are shown on the left. (B) Caseinolytic profiles of dialysate (lane 1) were performed by 12% SDS–PAGE–zymogram assay containing casein as a substrate. Zymograms were developed overnight at 37 °C in the presence of protease inhibitors as PMSF (lane 2) and EDTA (lane 3) at pH 10.5. Caseinolytic activity was observed as colorless bands.
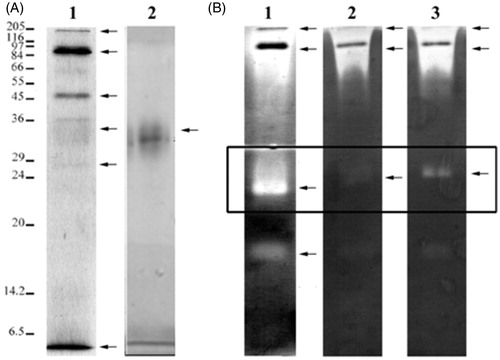
Results of caseinolytic activity on the SDS–PAGE–zymogram assay with dialysate (used as a control) are presented in , lane 1. At least five different protein bands were observed. Two bands between 116–205 and 84–97 kDa were obtained with wide caseinolytic activities. But at the same time, centers of these bands were observed as dark non-active bands as shown in the , lane 1. We assume that both bands are not pure and have various caseinolytic active and non-active peptides close to molecular mass. Molecular masses of these two bands were similar to results obtained from SDS–PAGE reducing gel. One strong and clear zone appeared as a new band ∼24 kDa on the non-reducing gel. When we compare reducing and non-reducing conditions of the loaded samples, this band profile seems to correspond to 29–36 kDa protein like partially purified caseinolytic enzyme. Additionally, one wide and weak new band was determined ∼20 kDa under non-reducing conditions. We also assumed this band possibly corresponds to 24–29 kDa protein band of reducing samples as indicated with arrow in , lane 1. Dialysate was further analyzed to determination the subtype of protease activity. Overnight incubation of the gel with PMSF (serine protease inhibitor) and EDTA (metalloproteinase inhibitor) resulted as shown in , lane 2 and lane 3, respectively. Results of the inhibitor effects on protease bands were stronger with PMSF and EDTA was not strongly effective. Especially the band between 20 and 24 kDa (see black square in ) was strongly inhibited by PMSF but partially inhibited by EDTA. The inhibition profile revealed by PMSF implied that the protease from B. pumilus D3 possibly belongs to the family of serine proteases. In addition, enzyme inhibited with some heavy metals suggests that the enzyme is non-metalloprotease.
Conclusions
The present study focused on optimization, characterization and purification of B. pumilus D3 protease. This protease showed the maximal activity at pH 11.0, 1% of inoculum size, 35 °C of temperature and 48 h of incubation period. Because the optimal reaction temperature was 55 °C, this thermostable enzyme might have advantages for industrial applications. Furthermore, the activity of the mentioned enzyme increased in the presence of SDS with concentration up to 5 mM. This enzyme might be a candidate biocatalyst for many industries due to its unique characteristics. A further experiment for enhanced enzyme production for commercialized process is needed.
Declaration of interest
The abstract of this study was sent to the 15th European Congress on Biotechnology and the only abstract was published in New Biotechnology.
Acknowledgements
This research is a part of the MSc thesis by B.Ö.
References
- Turk B. Targeting proteases: successes, failures and future prospects. Nat Rev Drug Discov 2006;5:785–99
- Sumantha A, Larroche C, Pandey A. Microbiology and industrial biotechnology of food-grade proteases: a perspective. Food Technol Biotechnol 2006;44:211–20
- Cleynen E, Schoofs L, Salzet M. A review of the most important classes of serine protease inhibitors in insects and leeches. Med Chem Rev 2005;2:197–206
- Kumar CG, Takagi H. Microbial alkaline proteases: from a bioindustrial view point. Biotechnol Adv 1999;17:561–94
- Chutmanop J, Chuichulcherm S, Chisti Y, Srinophakun P. Protease production by Aspergillus oryzae in solid-state fermentation using agroindustrial substrates. J Chem Technol Biotechnol 2008;83:1012–18
- Hema TA, Shiny M. Production of protease enzyme from Bacillus clausii Sm3. J Pharm Biol Sci 2012;1:37–40
- Rao MB, Tankasale AM, Ghatge MS, Deshpandeet VV. Molecular and biotechnological aspects of microbial proteases. Microbiol Mol Biol Rev 1998;62:597–634
- Raut S, Sen SK, Kabir NA, et al. Isolation and characterization of protease producing bacteria from upper respiratory tract of wild chicken. Bioinformation 2012;8:326–30
- Godfrey TA, Reichelt J. Industrial enzymology: the application of enzymes in industry. London: The Nature Press; 1985
- Wilson SA, Daniel RM, Peek K. Peptide synthesis with a proteinase from the extremely thermophilic organism Thermus Rt41A. Biotechnol Bioeng 1994;44:337–46
- Barthomeuf C, Pourrat H, Pourrat A. Collagenolytic activity of a new semi-alkaline protease from Aspergillus niger. J Ferment Bioeng 1992;73:233–6
- Fujiwara N, Yamamoto K, Masui A. Utilization of a thermostable alkaline protease from an alkaliphilic thermophile for the recovery of silver from used X-ray film. J Ferment Bioeng 1991;72:306–8
- Gupta R, Gupta K, Saxena RK, Khan S. Bleach-stable, alkaline protease from Bacillus sp. Biotechnol Lett 1999;21:135–8
- Saeki K, Ozaki K, Kobayashi T, Ito S. Detergent alkaline proteases: enzymatic properties, genes, and crystal structures. J Biosci Bioeng 2007;103:501–8
- Haddar A, Agrebi R, Bougatef A, et al. Two detergent stable alkaline serine proteases from Bacillus mojavensis A21: purification, characterization and potential application as a laundry detergent additive. Bioresour Technol 2009;100:3366–73
- Takami H, Akiba T, Horikoshi K. Production of extremely thermostable alkaline protease from Bacillus sp. Appl Microbiol Biotechnol 1989;30:120–4
- Bradford MM. A rapid and sensitive method for the quantification of protein using the principle of protein-dye binding. Anal Biochem 1976;72:248–54
- Marchesi JR, Sato T, Weightman AJ, et al. Design and evaluation of useful bacterium-specific PCR primers that amplify genes coding for bacterial 16S rRNA. Appl Environ Microbiol 1998;64:795–9
- Altschul SF, Madden TL, Schaffer AA, et al. Gapped BLAST and PSI-BLAST: a new generation of protein database search programs. Nucleic Acids Res 1997;25:3389–402
- Laemmli UK. Cleavage of structural proteins during assembly of the head of bacteriophage T4. Nature (London) 1970;277:680–5
- Wilkesman J, Kurz L. Protease analysis by zymography: a review on techniques and patents. Recent Pat Biotechnol 2009;3:175–84
- Veiga SS, Da Silveira RB, Dreyfuss JL, et al. Identification of high molecular weight serine-proteases in Loxosceles intermedia (brown spider) venom. Toxicon 2000;38:825–39
- Labud V, Garcia C, Hernandez T. Effect of hydrocarbon pollution on the microbial properties of a sandy and a clay soil. Chemosphere 2007;66:1863–71
- Margesin R, Walder G, Schinner F. The impact of hydrocarbon remediation (diesel oil and polycyclic aromatic hydrocarbons) on enzyme activities and microbial properties of soil. Acta Biotechnologica 2000;20:313–33
- Jayakumar R, Jayashree S, Annapurna B, Seshadri S. Characterization of thermostable serine alkaline protease from an alkaliphilic strain Bacillus pumilus MCAS8 and its applications. Appl Biochem Biotechnol 2012;168:1849–66
- Feng YY, Yang WB, Ong SL, et al. Fermentation of starch for enhanced alkaline protease production by constructing an alkalophilic Bacillus pumilis strain. Appl Microbiol Biotechnol 2001;57:153–60
- Shaheen MM, Shah AA, Hameed A, Hasan F. Influence of culture conditions on production and activity of protease from Bacillus subtillus BS1. Pak J Bot 2008;40:2161–9
- Tekin N, Cihan AÇ, Takaç ZS, et al. Alkaline protease production of Bacillus cohnii APT5. Turk J Biol 2012;36:430–40
- Cheetham PSJ. Principles of industrial biocatalysis and bioprocessing. In: Wiseman A, ed. Handbook of enzyme biotechnology. UK: Ellis Harwood; 1995
- Muderrizade A, Ensari NY, Aguloglu S, Otludil B. Purification and characterization of alkaline protease from alkalophilic Bacillus sp. Prik Biokhim Mikrobiol 2001;37:674–7
- Kumar CG. Purification and characterization of a thermostable alkaline protease from alkalophilic Bacillus pumilus. Lett Appl Microbiol 2002;34:13–17
- Padmapriya B, Rajeswari T, Nandita R, Raj F. Production and purification of alkaline serine protease from marine Bacillus species and its application in detergent industry. Eur J Appl Sci 2012;4:21–6
- Mrudula S, Shyam N. Immobilization of Bacillus megaterium MTCC 2444 by Ca-alginate entrapment method for enhanced alkaline protease production. Braz Arch Biol Technol 2012;55:135–44
- Nadeem M, Shahjahan B, Syed QA, et al. Microbial production of alkaline proteases by locally isolated Bacillus subtilis PCSIR-5. Pak J Zool 2006;38:109–18
- Irfan M, Gulsher M, Nadeem M, et al. Submerged production of alkaline protease by newly isolated Bacillus sp. Pak J Food Sci 2009;19:12–16
- Singh J, Batra N, Sobti RC. Serine alkaline protease from a newly isolated Bacillus sp., SSR1. Proc Biochem 2001;36:781
- Mazar FM, Mohammadi HS, Ebrahimi-Rad M, et al. Isolation, purification and characterization of a thermophilic alkaline protease from Bacillus subtilis BP-36. J Sci Islam Repub Iran 2012;23:7–13
- Ravishankar K, Kumar MA, Saravanan K. Isolation of alkaline protease from Bacillus subtilis AKRS3. Afr J Biotechnol 2012;11:13415–27
- Aoyama M, Yasuda M, Nakachi K, et al. Soybean-milk-coagulating activity of Bacillus pumilus derives from a serine proteinase. Appl Microbiol Biotechnol 2000;53:390–5
- Alencar RB, Biondi MM, Paiva PMG, et al. Alkaline proteases from the digestive tract of four tropical fishes. Braz J Food Technol 2003;6:279–84
- Gupta AI, Roy I, Patel RK, et al. One step purification and characterization of an alkaline protease from haloalkaliphilic Bacillus sp. J Chromatogr 2005;1075:103–8
- Uchida H, Kondo D, Yamashita S, et al. Purification and properties of a protease produced by Bacillus subtilis CN2 isolated from a Vietnamese fish sauce. World J Microbiol Biotechnol 2004;20:579–82
- Chomsri N. Thermostable protease enzymes. Master Thesis in Biotechnology. Chiangmai: The Graduate School, Chiangmai University; 2001
- Oberoi R, Beg QK, Puri S, et al. Characterization and wash performance analysis an SDS-stable alkaline protease from a Bacillus sp. World J Microbiol Biotechnol 2001;17:493
- Joo HS, Kumar CG, Park GC, et al. Oxidant and SDS-stable alkaline protease from Bacillus clasuii I-52: production and some properties. J Appl Microbiol 2003;95:267--72
- Bhaskar N, Sudeepa ES, Rashmi HN, Tamil Selvi A. Partial purification and characterization of protease of Bacillus proteolyticus CFR 3001 isolated from fish processing waste and its antibacterial activities. Biores Technol 2007;98:2758–64
- Banik R, Prakash M. Laundry detergent compatibility of the alkaline protease from Bacillus cereus. Microbiol Res 2004;159:135–40