Abstract
Carbonic anhydrases (CAs, EC 4.2.1.1) are metalloenzymes which catalyze a simple but physiologically crucial reaction in all life Domains, the carbon dioxide hydration to bicarbonate and protons: CO2 + H2O ⇔ + H+. These enzymes are involved in many physiologic processes, such as photosynthesis, respiration, CO2 transport, as well as metabolism of xenobiotics. Five different, genetically distinct CA families are known to date: the α-, β-, γ-, δ- and ζ-CAs. α-, β- and δ-CAs use Zn(II) ions at the active site, the γ-CAs are probably Fe(II) enzymes (but they are active also with bound Zn(II) or Co(II) ions), whereas the ζ-class uses Cd(II) or Zn(II) to perform the physiologic reaction catalysis. Bacteria encode for enzymes belonging to the α-, β-, and γ-CA classes. They contain zinc ion (Zn2+) in their active site, coordinated by three histidine residues and a water molecule/hydroxide ion (in the α and γ) or by two cysteine and one histidine residues (in the β class), with the fourth ligand being a water molecule/hydroxide ion. Here we propose that bacterial CAs can be used as markers for understanding the evolution and genetic variability of the Gram-positive and Gram-negative bacteria. We addressed several questions such as: (1) why are α-CAs present only in the genome of Gram-negative bacteria; (2) why are α-CAs not present in all Gram-negative bacteria; (3) why do Bacteria show an intricate pattern of CA gene expression; (4) what are the physiologic roles of such diverse CAs in these prokaryotes. We proposed possible answers to the previous questions. Moreover, we speculated on the evolution of the CA classes (α, β and γ) identified in the Gram-negative and -positive bacteria. Our main hypothesis is that from the ancestral Ur-CA, the γ-class arose first, followed by the β-class; the α-class CAs came last it is found only in the Gram-negative bacteria.
Introduction
All living organisms are related to each other by the presence of common biomolecules confirming that they all evolved from a common, universal ancestorCitation1–3. However, discerning how the different major groups (Domains) of organisms are related to each other and tracing back their evolution from the common ancestor remains a controversial and often unresolved issueCitation1. Prokaryotes and eukaryotes are two types of cells that are easily distinguishable, and they probably constitute the major divergence point in the evolution of life on earthCitation1. In the classification and identification of prokaryotes one empirical criterion that has huge practical value is the response of these organisms to the Gram stain, discovered by Christian Gram (1884)Citation4. The “Gram-positive bacteria”, i.e. those bacteria which are stained by the dye proposed by Gram, generally contain a thick cell wall that is very rich in cross-linked peptidoglycans, but also teichoic acids, teichuronic acid, and polysaccharidesCitation5. On the other hand, the “Gram-negative bacteria” have a thin layer of peptidoglycan and an outer membrane containing lipopolysaccharides, which lies outside of the peptidoglycan layer, and they are not stained by the Gram reagentCitation6–8. Accordingly, prokaryotes can be subdivided in three groups: (i) mycoplasma which do not synthesize a cell wall (Gram-negative), whose cell membrane serves as the outer bounding layer; (ii) the Gram-positive bacteria, which synthesize a monolayered cell wall; and (iii) the Gram-negative bacteria, which synthesize a cell wall composed of at least two structurally distinct layersCitation1,Citation9. The evolutionary history of organisms started to be investigated by comparing the nucleic acid sequences of common genes belonging to various species. The initial studies compared the oligonucleotide catalogs of 16S rRNA. Following this strategy, Woese and collaborators deduced that prokaryotes consisted of two monophyletic groups: eubacteria and archaebacteriaCitation10,Citation11. Subsequently, by comparing amino acid sequences of many proteins the organismal evolutionary history was boosted. The phylogenetic analysis, in fact, can be carried out by using either nucleic acid or protein sequencesCitation12,Citation13.
In this present paper we present an overview of the carbonic anhydrases (CAs, EC 4.2.1.1) present in Bacteria, and how their amino acid sequences might be used as new markers for understanding the genetic variability of the Gram-positive and Gram-negative bacteria. Moreover, this analysis may give more insights on the bacterial evolutionary history.
CAs catalyze a simple reaction in all life domains: the carbon dioxide hydration to bicarbonate and protons: CO2 + H2O ⇔ + H+ Citation14,Citation15. These enzymes are thus involved in many physiologic processes, such as photosynthesis, respiration, CO2 transport, as well as metabolism of xenobiotics (e.g. cyanate in Escherichia coli)Citation14,Citation15. Five different, genetically distinct CA families are known to date, the α-, β-, γ-, δ- and ζ-CAsCitation16–18. Whereas α-, β- and δ-CAs use Zn(II) ions at the active site, the γ-CAs are probably Fe(II) enzymes (but they are active also with bound Zn(II) or Co(II) ions), whereas the ζ-class uses Cd(II) or Zn(II) to perform the physiologic reaction catalysisCitation19–22. The 3D fold of the five CA classes is very different: α-CAs are normally monomers and rarely dimmers; β-CAs are dimers, tetramers or octamers; γ-CAs are trimers, whereas the δ- and ζ-CAs are less well understood (the only ζ-CA crystallized so far has three slightly different active sites on the same polypeptide chain whereas no X-ray crystal structures of δ-CAs are available so far)Citation19–21. Many representatives of all these enzyme classes have been crystallized and characterized in detail, except the δ-CAsCitation23–29.
Bacteria encode for enzymes belonging to the α-, β-, and γ-CA classesCitation30–43. They contain zinc ion (Zn2+) in their active site, coordinated by three histidine residues and a water molecule/hydroxide ion (in the α- and γ-CAs) or by two Cys and one His residues (in the β class), with the fourth ligand being a water molecule/hydroxide ion. Some of the catalytically active α-CAs can also catalyze the hydrolysis of esters, for example 4-nitrophenyl acetate (4-NpA) (and other hydrolytic reactions as well). However, no esterase activity was detected so far for enzymes belonging to the other four classesCitation21,Citation44–46.
Results and discussion
As mentioned above, Bacteria encode CAs belonging to the α-, β- and γ-CAs. However, how these enzyme classes are distributed in various bacteria is much less well understood. In fact, some of them encode CAs belonging only to one family, others from two or even three different genetic families, whereas quite a few species do not contain CAs at allCitation47, as the Gram-negative bacteria belonging to the genera Buchnera and Rickettsia. These observations provide some evidence that the evolution of CAs in prokaryotes has been characterized by a high degree of complexity. Why the evolution of Bacteria led to this intricate pattern of CA gene expression in various representatives of this Domain, and what are the physiologic roles of such diverse CAs in these prokaryotes, are again a poorly understood phenomena. In this paper we will examine the distributions of the three CA families in different bacteria for which the genomes have been cloned, and provide an analysis and a viewpoint connecting evolution of the CAs with evolution of Bacteria. A tentative explanation of the roles of the various CA types in these organisms will also be provided, again from an evolutionary perspective.
In we report the CA classes present in the Gram-negative and Gram-positive bacteria whose genome has bee cloned. was constructed analyzing the genome of bacteria for the presence of different CA classes. The search was carried out using the program Blast (Basic Local Alignment Search Tool)Citation48, employing the amino acid sequence of an α-, β- or γ-CA as query sequence, and comparing it with the genomic library of the microorganisms considered in the analysis.
Table 1. Distribution of CA families in Gram-negative/positive bacteria (for which the genome was cloned).
As shown in , α-CAs have been detected only in the genomes of Gram-negative bacteria whereas β-and γ-CAs have been identified in both Gram-negative and Gram-positive bacteria. Intriguingly, among the Gram-negative bacteria shown in the , only six microorganisms (Neisseria gonorrhoeae, Helicobacter pylori, Vibrio cholerae, Sulfurihydrogenibium yellowstonense, Sulfurihydrogenibium azorense and Ralstonia eutropha) expressed CAs belonging to the α-class. Moreover, N. gonorrhoeae is a Gram-negative bacterium encoding for only one class of CAs, the α-CA, whereas the genome of Staphylococcus aureus and Enterococcus faecalis (Gram-positive bacteria) encode only for γ-CAs.
Here we will try to answer first the following questions: (1) Why are α-CAs present only in the genome of Gram-negative bacteria? (2) Why are α-CAs not present in all Gram-negative bacteria?
A common feature of all bacterial α-CAs known to date (and as we mentioned earlier, there are not many of them) is that they have an N-terminal signal sequence indicating a periplasmic or extracellular location and a possible physiological role in CO2 uptake processesCitation49. As aforementioned, Gram-negative bacteria are surround by a second outer membrane which functions as an effective barrier. These bacteria must develop mechanisms to bring nutrients inside their cells from the surroundings. Several essential metabolic pathways require either CO2 or bicarbonate as a substrate. Indeed, bicarbonate is the substrate of several important enzymes of central metabolic pathways, such as phosphoenolpyruvate carboxylase, pyruvate carboxylase, acetyl-CoA carboxylase and methylcrotonyl CA, etc. We hypnotize here that in Gram-negative bacteria the spontaneous diffusion of CO2 to the outer membrane and the conversion to bicarbonate inside the cell are not sufficient for the metabolic needs of the organism. Thus, evolution adopted a brilliant strategy to accomplish the aforementioned function: the N-amino terminal sequence of a primordial CA was changed by introducing a signal peptide and generating a new CA class, named thereafter α-CA, localized in a secretory compartment, such as the periplasmic space. This is localization with respect to the cytoplasmic classes are named β-and γ-CAs. We believe that the bacterial α-CAs are able to convert the diffused CO2 in the periplasmic space into bicarbonate necessary for the bacterial metabolism, while the CAs belonging to the β- or γ-classes, with their cytoplasmic localization, are responsible for the CO2 feeding of carboxylase enzymes or for pH homeostasis and other intracellular functions.
Helicobacter pylori is a neutralophile with a bioenergetics profile suited for growth at neutral pH. However this pathogenic bacterium lives in the highly acidic environment of the stomach. Its genome encodes three CAs: one α-, one β- and one γ-CA, respectivelyCitation49–53. The α-CA was shown to possess a periplasmic localization, the β-CA has been found in the cytoplasm (ref), whereas no information is available on the expression/localization of the H. pylori γ-CA. It was suggested that the activity of periplasmic α- and β-CAs could be an additional requirement for the gastric colonization of the bacterium. H. pylori have developed exclusive adaptive mechanisms for growing in the highly acid environment of the stomach (pH between 1 and 2Citation54). These mechanisms involve the urease and the CA enzymes, which allow the bacteria to colonize the stomach by regulating the periplasmic pH and elevating the cytoplasmic pH trough the production of NH3. Under acidic conditions, urea goes into the cytoplasm through the urea channelCitation14 (). In the bacteria cytoplasm 2NH3 and CO2 are produced by hydrolysis of urea (). CO2 in the cytoplasm is hydrated by the β-CA, while the CO2 that diffused into the periplasm is converted to by the periplasmic α-CA. The produced ions, such as H+ are used by NH3 to form
in the periplasm and cytoplasm, respectivelyCitation14. Thus, as showed in , the role of periplasmic α-CA and the cytoplasmic β-CA is to generate
and protons, to both neutralize entering acid and buffer the periplasm and the cytoplasmCitation14.
Figure 1. Role of α- and β-CA in the maintenance of periplasmic pH in Helicobacter pylori. (see text for details). Figure from referenceCitation14.
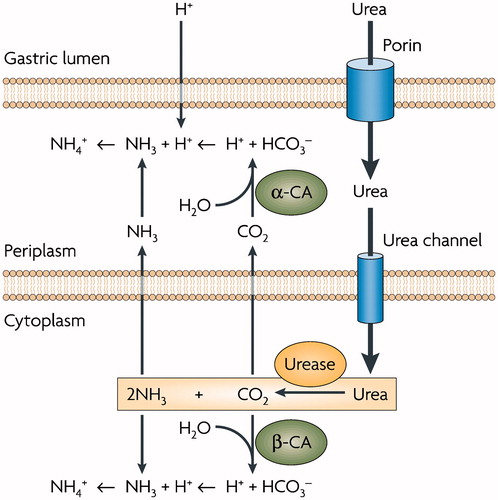
The crucial role played by these CAs present in H. pylori is the acid acclimatization of the pathogen within the stomachCitation55,Citation56. This role is corroborated by the in vivo inhibition studies carried out using inhibitors α and β-CAs in H. pylori. The inhibition of these two enzymes, in fact, led to the death of the bacteria and a possible eradication of H. pylori from the stomach and has been used clinically for the treatment of gastric ulcersCitation49,Citation50.
A similar case was reported for Ralstonia eutropha, a Gram-negative soil bacteriumCitation57. Its genome encodes for the three representatives of this gene superfamily, one α-, one β-, and one γ-CA, as H. pylori. A periplasmic α-CA was identified in this bacterium, again like in H. pylori, suggesting that this enzyme assists the transport of bicarbonate across the cell membraneCitation57.
We can also include in this discussion the Gram-negative bacterium Vibrio cholerae, which is the causative agent of choleraCitation32,Citation42,Citation58. The genome of V. cholerae encodes for putative CAs belonging to each bacterial class: α, β and γ. The α-CA has been cloned, purified, and characterized from our group and it was named VchCACitation32,Citation42,Citation58. This new enzyme showed significant catalytic activity, being more active than the human isoform hCA I or than the H. pylori α-class enzyme. An inhibition study with a panel of sulfonamides and one sulfamate led to the detection of a large number of low nanomolar VchCA inhibitors, including methazolamide, acetazolamide, ethoxzolamide, dorzolamide, brinzolamide, benzolamide, and indisulam (with KI in the range of 0.69–8.1 nM)Citation32,Citation42,Citation58. Recently it was reported that sodium bicarbonate induces cholera toxin (CT) expressionCitation59,Citation60. Although the mechanism for bicarbonate mediated CT induction has not been defined in detail, it was demonstrated that bicarbonate stimulates virulence gene expression by enhancing ToxT, a regulatory protein that directly activates transcription of the genes encoding CT activityCitation60. Addition of CA inhibitors (CAI) caused a significant reduction in virulence gene expression. Thus, bicarbonate was the first positive effector for ToxT activity to be identified. The bicarbonate ion is present at a high concentration in the upper small intestine colonized by V. cholera suggesting that the potential of V. cholera CAs could be implicated in the microbial virulenceCitation32,Citation42,Citation58. Since ethoxzolamide was shown to inhibit this virulence in vivoCitation42, we propose that VchCA may be a target for antibiotic development, exploiting a mechanism of action rarely considered until now. One may suggest that the V. cholerae α-CA has a periplasmic localization with the function of increasing the concentration of bicarbonate outside the bacterial cells which is necessary for the pathogenicity and the survival of the microorganism.
But now a new question arises: why are α-CAs not present in all Gram-negative bacteria? We can address this question assuming that not all Gram-negative bacteria need a periplasmic α-CA to adapt to the extreme habitats colonized by the microorganisms. In fact, if one considers the two bacteria belonging to the genus Sulfurihydrogenibium living in hot springs all over the world, at temperatures of up to 110 °C, they have α-CAs characterized by a signal peptide indicating a periplasmic or extracellular location of this enzymeCitation33,Citation35,Citation37,Citation40,Citation41,Citation43,Citation61,Citation62. The α-CAs from S. yellowstonense and S. azorense, denominated SspCA and SazCA, respectively, are probably involved in the CO2 fixation and biosynthetic processes, as for other bacteria, algae and plantsCitation33,Citation35,Citation37,Citation40,Citation41,Citation43,Citation61,Citation62. Such enzymes are highly thermostable, maintaining a good catalytic activity even after heating for a prolonged period (more than 3 h) to 100 °C, and they are highly effective catalysts for the CO2 hydration reaction. SazCA is the fastest CA known to date, and the second most efficient enzyme (after superoxide dismutase), with a kcat value of 4.40 × 106 and a kcat/KM value of 3.5 × 108 M−1 s−1. SspCA also showed a good catalytic activity for the same reaction, with a kcat value of 9.35 × 105 s−1 and a kcat/KM value of 1.1 × 108 M−1 s−1, proving that the “extremo-α-CAs” are indeed among the most effective CAs known to dateCitation33,Citation35,Citation37,Citation40,Citation41,Citation43,Citation61,Citation62. Thus, extreme habitats need the presence of α-CA in the outer membrane of the Gram-negative bacteria. Otherwise, when Gram-negative bacteria colonize habitats defined as “not extreme”, a periplasmic α-CA is not necessary.
In this paper we have provided an insight into the role, the presence or the non-presence of the periplasmic α-CAs in some model organisms such as H. pylori, R. eutropha, V. cholerae, S. yellowstonense and S. azorense. But there are few species of Gram-negative bacteria belonging to the genera Buchnera and Rickettsi which do not contain any class of CAsCitation47. Recently, CAs knockout mutants of Ralstonia eutropha, Escherichia coli, and Saccharomyces cerevisiae were obtainedCitation47. These mutants were unable to grow under ambient air but grew normally under an atmosphere with a CO2 level in the range of 1–5%Citation47. High levels of CO2, in fact, generate high concentrations of bicarbonate spontaneously, through the uncatalyzed reaction. CA-negative microorganisms at low level of CO2 (0.035%) cannot initiate growth unless they are supplied with a sufficient concentration of bicarbonateCitation47. This indicates that CA is not essential for microbial growth in high-CO2 environments, such as in soil, seawater, intestine, and some other syntrophic and commensal situations, but it becomes essential when low concentrations of this gas are available. Thus, Ueda et al. suggested that the CA distribution in microbial genome reflects the history of adaptation of microorganisms to their environmentCitation47.
We want address another unanswered question. Could the different distribution of the α-, β- and γ-CAs in the Gram-negative and Gram-positive bacteria be associated with the evolutionary history of these microorganisms?
That the ancestral organisms were prokaryotes and that the eukaryotes originated from them at a later time, is a view consistent with the fossil record and the new molecular/genome data. The view that archaebacteria are monophyletic and distinct from other prokaryotes is supported by the existence of protein sequences which are present in Archaea but not in any eubacteriaCitation1. Moreover, most of the phylogenetic methods support a close relationship of Archaea with Gram-positive bacteria, whereas Gram-negative bacteria form a separate clade, indicating their phylogenetic distinctness. Gupta et al. sustain that Gram-positive bacteria occupy an intermediate position between Archaea and Gram-negative bacteria, and this latter group has evolved from themCitation1.
Here we have constructed several phylogenetic trees in order to better investigate the evolutionary relationship of the α, β and γ-CAs with respect to the Gram-negative and Gram-positive bacteria evolutionary history. The dendrograms showed in and have been obtained by aligning the amino acid sequences of the β- or γ-CAs identified in the genome of the bacteria reported in . shows that γ-CAs from Gram positive and Gram-negative bacteria seemed closely associated to each other. In some cases, γ-CAs from Gram-positive bacteria seemed clustered in branches distinct from that of Gram-negative bacteria. Intriguingly, from the dendrogram showed in , all the β-CAs from the Gram-positive bacteria, with the exception of two β-CAs, i.e. LxiCAbeta_ + and PgiCAbeta_-, seemed closely associated to each other forming two distinct clusters: one contained the β-CAs only from Gram-negative bacteria and the other included all the β-CAs only from Gram-positive bacteria. Our hypothesis is that the two sequences aforementioned sequences (LxiCAbeta_ + and PgiCAbeta_-) can be considered as the transition amino acid sequences before the divergence of Gram-positive and Gram-negative bacteria.
Figure 2. Phylogenetic analysis of the γ-CAs of the Gram-negative and -positive bacteria indicated in . The tree was constructed using the program PhyML 3.0. Branch support values are reported at branch point. Accession numbers of the amino acid sequences used in the phylogenetic analysis are given in .
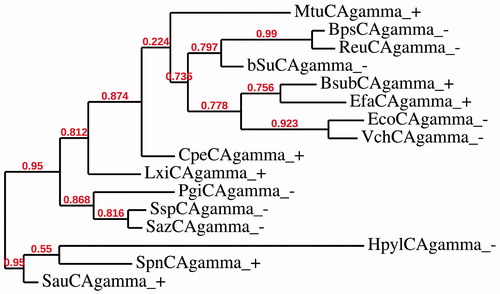
Figure 3. Phylogenetic analysis of the β-CAs of the Gram-negative and -positive bacteria indicated in . The tree was constructed using the program PhyML 3.0. Branch support values are reported at branch point. Accession numbers of the amino acid sequences used in the phylogenetic analysis are given in .
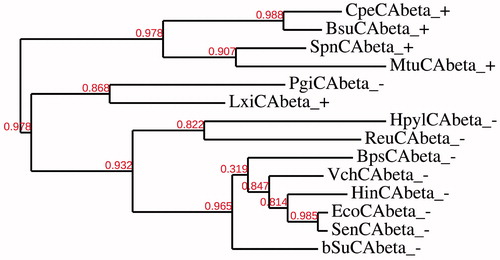
We have thus constructed a new dendrogram to better investigate the relationship among the bacterial CA classes. From the dendrogram showed in we notice again that the two sequences LxiCAbeta_ + and PgiCAbeta_- are positioned between the β-CAs from Gram-negative and Gram-positive bacteria. But, the most interesting aspect emerging from this new dendrogram was that PgiCAbeta_- can be considered as the ancestral sequence possibly at the origin of the common ancestor of all the α-CAs of the Gram-negative bacteria. The tree was obtained by using the amino acid sequences belonging to all three bacterial CA classes (α, β and γ) from Gram-positive and Gram-negative bacteria (). α-CAs, in fact, formed a distinct cluster, which is positioned in the same cluster of the β-CAs from Gram-negative bacteria. This information prompts us to speculate that the γ-CAs from Gram-negative and -positive bacteria have amino acid substitutions that make them closely related to any γ-CAs identified from Gram-negative or -positive bacteria. On the other hand, the β-CAs from Gram-negative and positive bacteria forming two distinct clusters may be the result of the duplication of an ancestral CA gene. Here, we hypothesize that the most ancestral CA is the γ-class of CAs and we nominate this most ancient enzyme as the “Ur-CA” (from the German word Ur meabning the ancestral form). In fact, although the γ class is widely distributed among all three Domains of life, it is the only CA class mainly identified in Archaea, the most ancient microorganisms that exist on earthCitation63–66. This is consistent with the theory, which supports a close relationship of Archaea to Gram-positive bacteria and considers Gram-negative bacteria phylogenetically distinct. Moreover, β-CAs from Gram-positive bacteria are phylogenetically distinct from the β-CAs identified in Gram-negative bacteria (). Probably we can assume that starting from the γ-class, the β-class originated and this event is associated with the origin of Gram-negative bacteria, leading to the two distinct clusters of β-CAs, one for Gram-positive and the other for Gram-negative bacteria (). Again, from such an ancestral β-CA from a Gram-negative bacterium, all the α-CAs arose, which are exclusively present only in the Gram-negative bacteria. The hypothesis presented above is the result of the detailed phylogenetic analysis performed by us, according to which from the ancestral Ur-CA, the γ-class has arisen first, followed by the β-class, whereas the α-class CAs was the last originated CA class. This hypothesis might be corroborated also by considering the theory of “enzyme promiscuity”Citation67–69. Catalytic sites can undergo structural changes while the function of the enzyme remains almost the sameCitation70. The positioning of key elements in the catalytic site can alter slightly through mutations without affecting the enzyme function, and in the mutant enzymes changes of the nature or sequence of catalytic residues may occurCitation70. For example, the zinc ion (Zn2+) in the active site of the bacterial CAs is coordinated by three histidine residues and a water molecule/hydroxide ion (in the α- and γ) or by two cysteine and one histidine residues (in the β class), with the fourth ligand being a water molecule/hydroxide ionCitation71,Citation72. Thus, the function of an enzyme depends upon the geometry of the catalytic and substrate binding residues.
Figure 4. Phylogenetic analysis of the α-, β- and γ-CAs of the Gram-negative and -positive bacteria indicated in . The tree was constructed using the program PhyML 3.0. Branch support values are reported at branch point. Accession numbers of the amino acid sequences used in the phylogenetic analysis are given in .
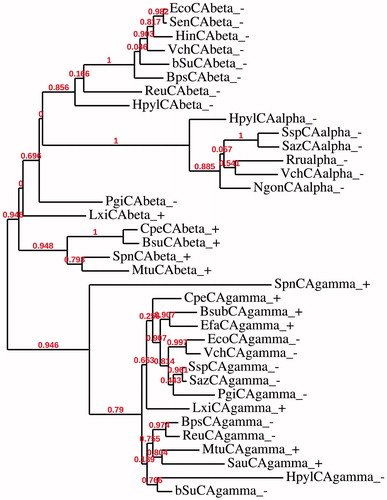
Figure 5. Three-dimensional structure of gamma- (Cam), beta- (Can2) and alpha- (hCA II) CAs, with a view of the catalytic pocket.
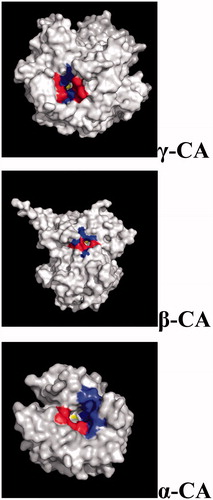
By analyzing for example the size of the catalytic site of the α, β and γ-CAs we noticed that the catalytic pocket is rather small for the γ-CAs, it gets bigger for β-CAs, and it becomes quite large in the α-CAs (). However, the catalytic function of CO2 hydration is maintained for all the bacterial CA classes, despite the diverse spatial reorganization of the active sites. Interestingly, in general the catalytic efficiency of the γ-CAs is low compared to the β-CAs which in turn is less efficient catalysts compared to many bacterial α-CAs ().
Table 2. Kinetic parameters for the CO2 hydration reaction catalyzed by various archaeal/bacterial CAs belonging to the various families and their inhibition data with the sulfonamide acetazolamide.
Table 3. Proteins, organisms, accession numbers and cryptonyms of the sequences used in the phylogenetic analysis.
In this regard, again we will use the concept of enzyme promiscuity to analyze the catalytic versatility of bacterial CAs. This often takes the form of an enzyme that catalyzes similar reaction chemistry on a range of substrates; for example, chymotrypsin catalyzes the hydrolysis not only of peptides, but also esters, thiol esters, acid chlorides and anhydridesCitation69. Tawfik considers the promiscuity a key factor in the evolution of a new protein functionCitation69. We wish to highlight the fact that γ-CAs use only CO2 as substrate, while β-CAs can hydrolyze CO2, H2S and COSCitation73. Furthermore, α-CAs not only hydrate CO2, CS2 but also possess esterase activity, with a range of esters of carboxylic, sulfonic or phosphate esters. This observation suggests that, as seen in phylogenetic analysis, the most recent form is the α-CA class (which acquired additional catalytic activities) whereas the γ-class, with the smallest catalytic pocket is the most ancient CA class, catalyzing only CO2 hydration (with a rather weak catalytic efficiency).
Conclusion
In this paper we propose that bacterial CAs can be used as markers for understanding the evolution and genetic variability of the Gram-positive and Gram-negative bacteria, based on a detailed phylogenetic analysis of the CA families present in these organisms. We addressed several questions as: (1) why are α-CAs present only in the genome of Gram-negative bacteria; (2) why are α-CAs not present in all Gram-negative bacteria; (3) why do Bacteria show an intricate pattern of CA gene expression, and (4) what are the physiologic roles of such diverse CAs in these prokaryotes. Possible explanations to all of them have been proposed. Moreover, the paper speculates on the evolution of the CA classes (α, β and γ) identified in the Gram-negative and -positive bacteria. Our main hypothesis is that from the ancestral Ur-CA, the γ-class has arisen first, followed by the β-class, and the α-class were the last originated CAs, being present only in Gram-negative bacteria. This is also discussed considering that the γ-CAs were the least effective as catalysts for CO2 hydration among the three classes, have small active site and and possess no additional catalytic activity. The β-CAs have larger active sites compared to the γ-CAs and in addition to the CO2 hydration they also catalyze COS and CS2 hydration reactions. The most recent class, the α-CAs possess the largest active site as well as high catalytic versatility, being esterases with a range of substrates in addition to hydrating CO2, cyanamide, cyanate, and COS among others.
Declaration of interest
The authors declare no conflict of interest.
References
- Gupta RS. Protein phylogenies and signature sequences: a reappraisal of evolutionary relationships among archaebacteria, eubacteria, and eukaryotes. Microbiol Mol Biol Rev 1998;62:1435–91
- Hoenigsberg H. Evolution without speciation but with selection: LUCA, the Last Universal Common Ancestor in Gilbert's RNA world. Genet Mol Res 2003;2:366–75
- Kim KM, Caetano-Anolles G. The evolutionary history of protein fold families and proteomes confirms that the archaeal ancestor is more ancient than the ancestors of other superkingdoms. BMC Evol Biol 2012;12:13
- Gram C. Ueber die isolierte farbung der Schizomyceten in Schnittund Trockenpraparaten. Fortschr Med 1888;2:185–9
- Orsi N, Fabozzi G, Ambrosi C, Visca P. Is there an answer? Is it better for a bacterium to be gram-positive or gram-negative? IUBMB Life 2004;56:361–3
- Page MG. The role of the outer membrane of Gram-negative bacteria in antibiotic resistance: Ajax' shield or Achilles' heel? Handb Exp Pharmacol 2012;211:67–86
- Varbanets LD. Outer membrane proteins of Gram-negative bacteria. Mikrobiol Zh 1980;42:661–71
- Nakae T, Nikaido H. The structural basis of outer membrane permeability in gram-negative bacteria (author’s transl). Nihon Saikingaku Zasshi 1978;33:715–27
- Schaechter M, Ingraham JL, Neidhardt FC. The road from The Microbial world to Microbe. Int Microbiol 2007;10:153–6
- Stackebrandt E, Woese CR. The phylogeny of prokaryotes. Microbiol Sci 1984;1:117–22
- Fox GE, Stackebrandt E, Hespell RB, et al. The phylogeny of prokaryotes. Science 1980;209:457–63
- Kibak H, Taiz L, Starke T, et al. Evolution of structure and function of V-ATPases. J Bioenerg Biomembr 1992;24:415–24
- Gogarten JP, Starke T, Kibak H, et al. Evolution and isoforms of V-ATPase subunits. J Exp Biol 1992;172:137–47
- Supuran CT. Carbonic anhydrases: novel therapeutic applications for inhibitors and activators. Nat Rev Drug Discov 2008;7:168–81
- Supuran CT. Bacterial carbonic anhydrases as drug targets: toward novel antibiotics? Front Pharmacol 2011;2:34. doi: 10.3389/fphar.2011.00034
- Supuran CT. Carbonic anhydrase inhibitors: an editorial. Expert Opin Ther Pat 2013;23:677–9
- De Simone G, Alterio V, Supuran CT. Exploiting the hydrophobic and hydrophilic binding sites for designing carbonic anhydrase inhibitors. Expert Opin Drug Discov 2013;8:793–810
- Supuran CT. Structure-based drug discovery of carbonic anhydrase inhibitors. J Enzyme Inhib Med Chem 2012;27:759–72
- Vullo D, Del Prete S, Osman SM, et al. Sulfonamide inhibition studies of the delta-carbonic anhydrase from the diatom Thalassiosira weissflogii. Bioorg Med Chem Lett 2014;24:275–9
- Del Prete S, Vullo D, Scozzafava A, et al. Cloning, characterization and anion inhibition study of the delta-class carbonic anhydrase (TweCA) from the marine diatom Thalassiosira weissflogii. Bioorg Med Chem 2014;22:531–7
- Del Prete S, Vullo D, De Luca V, et al. Biochemical characterization of the delta-carbonic anhydrase from the marine diatom Thalassiosira weissflogii, TweCA. J Enzyme Inhib Med Chem 2014. [Epub ahead of print]. doi: 10.3109/14756366.2013.868599
- Capasso C, Supuran CT. Anti-infective carbonic anhydrase inhibitors: a patent and literature review. Expert Opin Ther Pat 2013;23:693–704
- Lehneck R, Neumann P, Vullo D, et al. Crystal structures of two tetrameric beta-carbonic anhydrases from the filamentous ascomycete Sordaria macrospora. FEBS J 2014;281:1759–72
- Di Fiore A, Truppo E, Supuran CT, et al. Crystal structure of the C183S/C217S mutant of human CA VII in complex with acetazolamide. Bioorg Med Chem Lett 2010;20:5023–6
- Avvaru BS, Wagner JM, Maresca A, et al. Carbonic anhydrase inhibitors. The X-ray crystal structure of human isoform II in adduct with an adamantyl analogue of acetazolamide resides in a less utilized binding pocket than most hydrophobic inhibitors. Bioorg Med Chem Lett 2010;20:4376–81
- Temperini C, Scozzafava A, Supuran CT. Carbonic anhydrase inhibitors. X-ray crystal studies of the carbonic anhydrase II-trithiocarbonate adduct – an inhibitor mimicking the sulfonamide and urea binding to the enzyme. Bioorg Med Chem Lett 2010;20:474–8
- Alterio V, Hilvo M, Di Fiore A, et al. Crystal structure of the catalytic domain of the tumor-associated human carbonic anhydrase IX. Proc Natl Acad Sci USA 2009;106:16233–8
- Di Fiore A, Monti SM, Hilvo M, et al. Crystal structure of human carbonic anhydrase XIII and its complex with the inhibitor acetazolamide. Proteins 2009;74:164–75
- Temperini C, Scozzafava A, Puccetti L, Supuran CT. Carbonic anhydrase activators: X-ray crystal structure of the adduct of human isozyme II with l-histidine as a platform for the design of stronger activators. Bioorg Med Chem Lett 2005;15:5136–41
- Vullo D, Del Prete S, Osman SM, et al. Sulfonamide inhibition studies of the gamma-carbonic anhydrase from the oral pathogen Porphyromonas gingivalis. Bioorg Med Chem Lett 2014;24:240–4
- Nishimori I, Vullo D, Minakuchi T, et al. Anion inhibition studies of two new beta-carbonic anhydrases from the bacterial pathogen Legionella pneumophila. Bioorg Med Chem Lett 2014;24:1127–32
- Del Prete S, De Luca V, Scozzafava A, et al. Biochemical properties of a new alpha-carbonic anhydrase from the human pathogenic bacterium, Vibrio cholerae. J Enzyme Inhib Med Chem 2014;29:23–7
- Alafeefy AM, Abdel-Aziz HA, Vullo D, et al. Inhibition of carbonic anhydrases from the extremophilic bacteria Sulfurihydrogenibium yellostonense (SspCA) and S. azorense (SazCA) with a new series of sulfonamides incorporating aroylhydrazone-, 1,2,4triazolo3,4-b1,3,4thiadiazinyl- or 2-(cyanophenylmethylene)-1,3,4-thiadiazol-3(2H)-yl moieties. Bioorg Med Chem 2014;22:141–7
- Vullo D, Sai Kumar RS, Scozzafava A, et al. Anion inhibition studies of a beta-carbonic anhydrase from Clostridium perfringens. Bioorg Med Chem Lett 2013;23:6706–10
- Vullo D, De Luca V, Scozzafava A, et al. The extremo-alpha-carbonic anhydrase from the thermophilic bacterium Sulfurihydrogenibium azorense is highly inhibited by sulfonamides. Bioorg Med Chem 2013;21:4521–5
- Luca VD, Vullo D, Scozzafava A, et al. An alpha-carbonic anhydrase from the thermophilic bacterium Sulphurihydrogenibium azorense is the fastest enzyme known for the CO2 hydration reaction. Bioorg Med Chem 2013;21:1465–9
- Di Fiore A, Capasso C, De Luca V, et al. X-ray structure of the first `extremo-alpha-carbonic anhydrase', a dimeric enzyme from the thermophilic bacterium Sulfurihydrogenibium yellowstonense YO3AOP1. Acta Crystallogr D Biol Crystallogr 2013;69:1150–9
- Del Prete S, Vullo D, De Luca V, et al. A highly catalytically active gamma-carbonic anhydrase from the pathogenic anaerobe Porphyromonas gingivalis and its inhibition profile with anions and small molecules. Bioorg Med Chem Lett 2013;23:4067–71
- Del Prete S, De Luca V, Vullo D, et al. Biochemical characterization of the gamma-carbonic anhydrase from the oral pathogen Porphyromonas gingivalis, PgiCA. J Enzyme Inhib Med Chem 2013. [Epub ahead of print]. doi: 10.3109/14756366.2013.822371
- Vullo D, De Luca V, Scozzafava A, et al. The first activation study of a bacterial carbonic anhydrase (CA). The thermostable alpha-CA from Sulfurihydrogenibium yellowstonense YO3AOP1 is highly activated by amino acids and amines. Bioorg Med Chem Lett 2012;22:6324–7
- Vullo D, De Luca V, Scozzafava A, et al. Anion inhibition studies of the fastest carbonic anhydrase (CA) known, the extremo-CA from the bacterium Sulfurihydrogenibium azorense. Bioorg Med Chem Lett 2012;22:7142–5
- Del Prete S, Isik S, Vullo D, et al. DNA cloning, characterization, and inhibition studies of an alpha-carbonic anhydrase from the pathogenic bacterium Vibrio cholerae. J Med Chem 2012;55:10742–8
- De Luca V, Vullo D, Scozzafava A, et al. Anion inhibition studies of an alpha-carbonic anhydrase from the thermophilic bacterium Sulfurihydrogenibium yellowstonense YO3AOP1. Bioorg Med Chem Lett 2012;22:5630–4
- Guzel O, Innocenti A, Vullo D, et al. 3-phenyl-1H-indole-5-sulfonamides: structure-based drug design of a promising class of carbonic anhydrase inhibitors. Curr Pharm Des 2010;16:3317–16
- Temperini C, Innocenti A, Guerri A, et al. Phosph(on)ate as a zinc-binding group in metalloenzyme inhibitors: X-ray crystal structure of the antiviral drug foscarnet complexed to human carbonic anhydrase I. Bioorg Med Chem Lett 2007;17:2210–15
- Winum JY, Temperini C, El Cheikh K, et al. Carbonic anhydrase inhibitors: clash with Ala65 as a means for designing inhibitors with low affinity for the ubiquitous isozyme II, exemplified by the crystal structure of the topiramate sulfamide analogue. J Med Chem 2006;49:7024–31
- Ueda K, Nishida H, Beppu T. Dispensabilities of carbonic anhydrase in proteobacteria. Int J Evol Biol 2012;2012:324549 (1–5)
- Menlove KJ, Clement M, Crandall KA. Similarity searching using BLAST. Methods Mol Biol 2009;537:1–22
- Nishimori I, Onishi S, Takeuchi H, Supuran CT. The alpha and beta classes carbonic anhydrases from Helicobacter pylori as novel drug targets. Curr Pharm Des 2008;14:622–30
- Morishita S, Nishimori I, Minakuchi T, et al. Cloning, polymorphism, and inhibition of beta-carbonic anhydrase of Helicobacter pylori. J Gastroenterol 2008;43:849–57
- Nishimori I, Minakuchi T, Kohsaki T, et al. Carbonic anhydrase inhibitors: the beta-carbonic anhydrase from Helicobacter pylori is a new target for sulfonamide and sulfamate inhibitors. Bioorg Med Chem Lett 2007;17:3585–94
- Nishimori I, Vullo D, Minakuchi T, et al. Carbonic anhydrase inhibitors: cloning and sulfonamide inhibition studies of a carboxyterminal truncated alpha-carbonic anhydrase from Helicobacter pylori. Bioorg Med Chem Lett 2006;16:2182–8
- Nishimori I, Minakuchi T, Morimoto K, et al. Carbonic anhydrase inhibitors: DNA cloning and inhibition studies of the alpha-carbonic anhydrase from Helicobacter pylori, a new target for developing sulfonamide and sulfamate gastric drugs. J Med Chem 2006;49:2117–26
- Chu S, Schubert ML. Gastric secretion. Curr Opin Gastroenterol 2012;28:587–93
- Marcus EA, Moshfegh AP, Sachs G, Scott DR. The periplasmic alpha-carbonic anhydrase activity of Helicobacter pylori is essential for acid acclimation. J Bacteriol 2005;187:729–38
- Sachs G, Weeks DL, Wen Y, et al. Acid acclimation by Helicobacter pylori. Physiology (Bethesda) 2005;20:429–38
- Gai CS, Lu J, Brigham CJ, et al. Insights into bacterial CO2 metabolism revealed by the characterization of four carbonic anhydrases in Ralstonia eutropha H16. AMB Express 2014;4:2–12
- Vullo D, Isik S, Del Prete S, et al. Anion inhibition studies of the alpha-carbonic anhydrase from the pathogenic bacterium Vibrio cholerae. Bioorg Med Chem Lett 2013;23:1636–8
- Cobaxin M, Martinez H, Ayala G, et al. Cholera toxin expression by El Tor Vibrio cholerae in shallow culture growth conditions. Microb Pathog 2014;66:5–13
- Abuaita BH, Withey JH. Bicarbonate induces Vibrio cholerae virulence gene expression by enhancing ToxT activity. Infect Immun 2009;77:4111–20
- Vullo D, Luca VD, Scozzafava A, et al. The alpha-carbonic anhydrase from the thermophilic bacterium Sulfurihydrogenibium yellowstonense YO3AOP1 is highly susceptible to inhibition by sulfonamides. Bioorg Med Chem 2013;21:1534–8
- Akdemir A, Vullo D, De Luca V, et al. The extremo-alpha-carbonic anhydrase (CA) from Sulfurihydrogenibium azorense, the fastest CA known, is highly activated by amino acids and amines. Bioorg Med Chem Lett 2013;23:1087–90
- Zimmerman S, Innocenti A, Casini A, et al. Carbonic anhydrase inhibitors. Inhibition of the prokariotic beta and gamma-class enzymes from Archaea with sulfonamides. Bioorg Med Chem Lett 2004;14:6001–6
- Tripp BC, Bell CB 3rd, Cruz F, et al. A role for iron in an ancient carbonic anhydrase. J Biol Chem 2004;279:6683–7
- Tripp BC, Smith K, Ferry JG. Carbonic anhydrase: new insights for an ancient enzyme. J Biol Chem 2001;276:48615–18
- Smith KS, Jakubzick C, Whittam TS, Ferry JG. Carbonic anhydrase is an ancient enzyme widespread in prokaryotes. Proc Natl Acad Sci USA 1999;96:15184–9
- Baas BJ, Zandvoort E, Geertsema EM, Poelarends GJ. Recent advances in the study of enzyme promiscuity in the tautomerase superfamily. Chembiochem 2013;14:917–26
- Chakraborty S, Minda R, Salaye L, et al. Promiscuity-based enzyme selection for rational directed evolution experiments. Methods Mol Biol 2013;978:205–16
- Khersonsky O, Tawfik DS. Enzyme promiscuity: a mechanistic and evolutionary perspective. Annu Rev Biochem 2010;79:471–505
- Torrance JW, Holliday GL, Mitchell JB, Thornton JM. The geometry of interactions between catalytic residues and their substrates. J Mol Biol 2007;369:1140–52
- Supuran CT. Carbonic anhydrases – an overview. Curr Pharm Des 2008;14:603–14
- Supuran CT. Carbonic anhydrase inhibitors. Bioorg Med Chem Lett 2010;20:3467–74
- Smeulders MJ, Barends TR, Pol A, et al. Evolution of a new enzyme for carbon disulphide conversion by an acidothermophilic archaeon. Nature 2011;478:412–16
- Maresca A, Vullo D, Scozzafava A, Supuran CT. Inhibition of the alpha- and beta-carbonic anhydrases from the gastric pathogen Helycobacter pylori with anions. J Enzyme Inhib Med Chem 2013;28:388–91
- Joseph P, Ouahrani-Bettache S, Montero JL, et al. A new beta-carbonic anhydrase from Brucella suis, its cloning, characterization, and inhibition with sulfonamides and sulfamates, leading to impaired pathogen growth. Bioorg Med Chem 2011;19:1172–8
- Joseph P, Turtaut F, Ouahrani-Bettache S, et al. Cloning, characterization, and inhibition studies of a beta-carbonic anhydrase from Brucella suis. J Med Chem 2010;53:2277–85